DOI:
10.1039/D3AN01727C
(Paper)
Analyst, 2024,
149, 82-87
A cascade signal-amplified fluorescent biosensor combining APE1 enzyme cleavage-assisted target cycling with rolling circle amplification†
Received
8th October 2023
, Accepted 19th November 2023
First published on 21st November 2023
Abstract
A cascade signal-amplified fluorescent biosensor was developed for miRNA-21 detection by combining APE1 enzyme-assisted target recycling and rolling circle amplification strategy. A key feature of this biosensor is its dual-trigger mechanism, utilizing both tumor-endogenous miRNA-21 and the APE1 enzyme in the initial amplification step, followed by a second rolling circle amplification reaction. This dual signal amplification cascade significantly enhanced sensitivity, achieving a detection limit of 3.33 pM. Furthermore, this biosensor exhibited excellent specificity and resistance to interference, allowing it to effectively distinguish and detect the target miRNA-21 in the presence of multiple interfering miRNAs. Moreover, the biosensor maintained its robust detection capabilities in a 10% serum environment, demonstrating its potential for clinical disease diagnosis applications.
1. Introduction
MicroRNAs (miRNAs) are endogenous RNA molecules found in eukaryotic cells, not encoding proteins themselves.1,2 MiRNAs actively participate in processes such as degrading or obstructing target mRNA after transcription, playing a pivotal role in regulating human cell development, proliferation, differentiation, and apoptosis. Over the past few years, extensive research has highlighted the potential of miRNAs as biomarkers for diagnosing different types of cancer. For instance, miRNA-21, specifically, exhibits a close correlation with the proliferation, apoptosis, and migration of malignant tumors. It's frequently found to be overexpressed in conditions like chronic lymphoblastic leukemia, lung cancer, breast cancer, and prostate cancer.3–5 Therefore, miRNAs have emerged as highly promising targets for both cancer diagnosis and therapeutic intervention. However, it's worth noting that relying on a single biomarker for diagnosis may not always guarantee accuracy, and how to improve the diagnostic system's precision remains a challenge.
In addition, sensitively and effectively detecting miRNA is challenging due to their small size, susceptibility to degradation, and sequence similarities.6 Various signal amplification methods are utilized in existing nucleic acid biosensors for miRNA detection. These methods include catalytic hairpin assembly (CHA),7 hybridization chain reaction (HCR),8 and entropy-driven circuit (EDC),9 which enable target cycling. However, they demand precise sequence design and can suffer from issues like background signal leakage and false positives. Enzyme-mediated signal amplification is widely used in biological detection due to enzymes’ inherent reactivity. However, most enzymes employed are external proteases, which are not well-suited for in vivo amplification needs.10–12 A promising exploration lies in leveraging endogenous enzymes within the body to construct signal amplification strategies.
Apurinic/apyrimidinic endonuclease 1 (APE1) is a crucial endogenous multifunctional enzyme that plays a significant role in the base excision repair (BER) pathway.13 It exhibits strong enzymatic activity in cleaving specific apurinic/apyrimidinic (AP) sites within random double-stranded DNA (dsDNA) through hydrolyzing DNA phosphodiester backbone.14–17 Hence, it is feasible to leverage its enzyme cleavage capability, designing a strategy for APE1-assisted miRNA recycling to enable signal amplification. Furthermore, APE1 is frequently found to be overexpressed in various cancer cells, making it a potential cancer biomarker.18,19 Considering the recognition of multiple cancer biomarkers that are overexpressed in tumor cells is crucial for precise cancer diagnosis, we believe it is significant to utilize APE1 in conjunction with other biomarkers, such as miRNA, to create a dual-trigger “AND” logic gate for in vitro detection and in vivo biological imaging.16,20 This innovative approach of incorporating APE1 in constructing biosensors has the potential to simultaneously establish sensitive and logic-controllable dual-trigger detection systems for precise tumor diagnosis.
Additionally, the rolling circle amplification (RCA) reaction is an isothermal amplification technique employed for DNA and RNA replication by producing DNA segments with repetitive units, which play a signal amplification role in nucleic acid biosensors with improved sensitivity.21–25 However, in most biosensors where the RCA reaction is triggered directly by the target miRNA as the primer, only single signal amplification is achieved.26 To address this limitation, we propose triggering the rolling circle amplification reaction using the primer released from the preceding target cycle reaction.27–29 This approach aims to accomplish cascade signal amplification,30–32 thereby enhancing target detection sensitivity.
Based on the above thoughts, we proposed a cascade signal-amplified fluorescent biosensor for highly sensitive detection of miRNA-21 (Scheme 1), combining an APE1 enzyme cleavage-assisted target cycling strategy with rolling circle amplification (RCA) strategy. The entire experimental design is given as follow: (1) first, we designed an HP hairpin chain containing an AP site on the loop and specific regions in the loop that could hybridize with the target miRNA 21. When target miRNA 21 is present, it can hybridize with the loop of the HP hairpin, and then the AP site on the hybridized duplex is cleaved by APE1 enzyme, leading to the cleavage of the HP strand into two parts, HP1 and HP2, with the miRNA-21 released for the next cycle. Hence, the APE1 enzyme-assisted target miRNA-21 circulation serves as the first signal amplification step in the biosensor. It should be noted that the design of the HP hairpin stem with 3-nt mismatches and 7-nt sticky ends aims to achieve improved separation of HP1, HP2, and miRNA-21 after APE1 cleavage. (2) To achieve secondary signal amplification, we prepared a circular template DP and hybridized the released HP1 from the first step to initiate the RCA reaction. The DP circular template was obtained through annealing dumbbell L-DP and connecting it with T4 DNA ligase. The released HP1 in the previous step, as a primer, can undergo the RCA reaction with DP as the transcriptional template driven by Bst polymerase when hybridizing with the dumbbell-shaped DP chain, as its 3′ end is –OH after APE1 enzyme cleavage. As a result of this process, the final obtained RCA product contains a large number of G-quadruplex repeat units, as designed. (3) The G-quadruplexes can be specifically recognized by the Thioflavin T (ThT) dye, leading to a significant ThT fluorescence enhancement, as the final signal output step.33–37 Both of the above enzymes (APE1 and polymerase) have high biological activity and can achieve efficient target cycling and rapid generation of G-quadruplex, thus achieving highly efficient cascade amplification of detection signals. Furthermore, the combination of target miRNA-21 and APE1 could serve as a dual-switch to initiate the cascade signal amplification reaction and final signal output, ensuring the specific detection of tumor-associated miRNA.
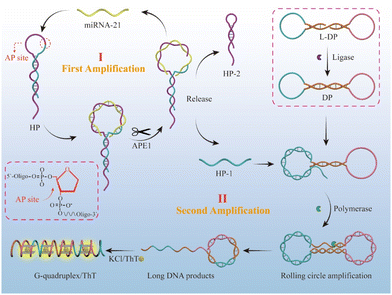 |
| Scheme 1 Schematic representation of a fluorescence biosensor employing a cascade signal amplification mechanism for the detection of microRNA-21. The biosensor combines an APE1 enzyme-assisted target cycling strategy with the rolling circle amplification (RCA) of a G-quadruplex structure, leading to a significant fluorescence enhancement by G-quadruplex recognizing Thioflavin T (ThT) dye as the signal output. | |
2. Experimental section
2.1 Materials
The Reagents and chemicals used are shown in the ESI.†
2.2 Apparatus
The apparatus used is shown in the ESI.†
2.3 Preparation of dumbbell template DP
Firstly, the L-DP template chain was dissolved in ultrapure water to achieve a concentration of 100 μM. Subsequently, a solution of 2 μM L-DP was prepared in a sterilized PCR tube with 1× DNA ligase buffer (containing 50 mM Tris HCl, 10 mM MgCl2, 1 mM ATP, 10 mM DTT, pH = 7.5) for PCR annealing, transforming it into a dumbbell configuration. Next, 2.5 μL of T4 DNA ligase (at a concentration of 400
000 U mL−1) was added to the system, resulting in a final total volume of 100 μL in the PCR tube. The mixture was then incubated for 6 hours at 16 °C, followed by heating at 65 °C for 10 minutes to inactivate the enzyme. Finally, the sample was stored at −20 °C for further use.
2.4 Detection of target miRNA-21
The experimental process involves three steps as follows: (1) A certain concentration of miRNA-21 was mixed with 200 nM HP and 0.01 U μL−1 of APE 1 in 1× Bst reaction buffer (containing 20 mM Tris HCl, 10 mM (NH4)2SO4, 10 mM KCl, 12 mM MgSO4, 0.1% Triton X-100, pH = 8.8). The mixture was then incubated at 37 °C for 1 hour. (2) Next, DP (200 nM), deoxyribonucleotide triphosphates (dNTPs) (0.5 mM), and Bst polymerase (0.2 U μL−1) were added into the above system, resulting in a total reaction volume of 20 μL. The RCA reaction was carried out at 37 °C for 3 hours and then at 85 °C for 20 minutes to inactivate Bst polymerase. (3) ThT (40 μM) and KCl (10 mM) were added to the RCA reaction product, and the fluorescence signal was detected using a fluorescence spectrophotometer with an excitation wavelength of 445 nm.
2.5 Specificity analysis
In the specificity detection, the targets miRNA-21 (200 nM) and other interfering RNAs, including miRNA-155 (200 nM), miRNA-141 (200 nM), let-7a (200 nM), and a mixture containing all four miRNAs at concentrations of 200 nM, were added separately into the detection system containing APE1 and hairpin HP. The mixture was then incubated at 37 °C for 1 hour. Subsequently, DP, dNTPs, and Bst polymerase were added into the system to accomplish the RCA reaction. ThT (40 μM) and KCl (10 mM) were added to generate a fluorescence signal. The detailed operation of this part was the same as in Experiment 2.4.
2.6 Real sample analysis
The healthy human serum samples were obtained from Academy of Military Sciences. The serum was diluted to 10 times and spiked with different concentrations of miRNA 21 (1 nM, 5 nM, 10 nM) for experimental group measurement. While the control group only contained 1× Bst reaction buffer with different concentrations of miRNA 21.
3. Results and discussion
3.1 Feasibility investigation
We first verified the feasibility of the experimental design sequence using polyacrylamide gel electrophoresis (PAGE). As shown in Fig. 1A, lane 1 represents miRNA-21; lane 2 shows the hairpin HP containing an AP site on the loop; lane 3 is the circular dumbbell template DP; lane 4 shows a new hybridized product band of miRNA-21 and HP; lane 5 shows the result of APE1 enzyme cleaving the hybridized duplex of miRNA-21 and HP, leading to the appearance of released HP1 and HP2 bands. In lane 6, new bands indicate the hybridization between DP circular template and the released HP1 from lane 5. The band at the top of lane 7 represents the long strand products of the RCA reaction after the hybridization of HP1 with DP template, catalyzed by Bst polymerase. The appearance of this band confirms that the designed DNA sequence can generate long strand RCA products under the trigger of the target miRNA-21. In comparison, the absence of APE1 in the lane 8 reaction system, and the absence of miRNA-21 in the lane 9 reaction system, demonstrate that in the absence of APE1 or miRNA-21, the HP hairpin will not be cleaved to release the HP1 chain, thereby not triggering the subsequent RCA reaction. Thus, these results confirm that the designed biosensor indeed utilizes miRNA-21 and APE1 as dual triggers for initiating the final RCA reaction.
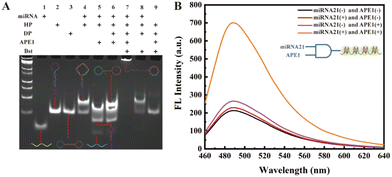 |
| Fig. 1 The feasibility verification of the designed experimental system in (A) 8% PAGE and (B) in fluorescence spectrum. The inset of (B) showing miRNA-21 and APE1 as dual triggers for initiating the RCA reaction and enhancing ThT fluorescence signal. | |
After obtaining the long DNA chain with G-quadruplex repeat units through the RCA reaction, we introduced KCl/ThT into the experimental system, leading to enhanced ThT fluorescence signal. Subsequently, we detected the changes in the fluorescence signal from different control groups. As depicted in Fig. 1B, the fluorescence signal is strongest when both the target miRNA-21 and APE1 enzyme are present, while the fluorescence signal is significantly weaker when either miRNA-21 or APE1 is absent in the system. These results demonstrate that only in the presence of dual-switch, the target miRNA-21 and APE1 enzyme, the HP1 primer chain is released and initiates the RCA reaction, resulting in the formation of G-quadruplex/ThT, and the enhanced fluorescent signal for biosensing. Based on the PAGE and fluorescence results, the proposed cascade signal-amplified strategy for the detection of miRNA 21 is feasible.
3.2 Optimization of assay conditions
The experimental process mainly consists of three parts, and to achieve better detection performance, we optimized the experimental conditions for each part. First, we optimized the APE1 concentration and reaction time. As shown in Fig. 2A, when the hairpin HP existed alone (lane 1), HP was not cut by APE 1 enzyme even as the APE1 concentration increased (lane 3, 5, 7); when HP hybridized with miRNA-21 to form a double stranded structure (lane 2), the addition of APE1 with the corresponding concentration (as lane 3, 5, 7) could cleave the AP site and release HP1 (lane 4, 6, 8). Therefore, based on the same cutting effect, we selected an APE1 concentration of 0.05 U μL−1 for the subsequent experiments. Subsequently, we proceeded to optimize the cleavage reaction time of the APE1 enzyme. In Fig. 2B, both the HP and miRNA-21 were present at concentrations of 1 μM, while the APE1 enzyme concentration was maintained at 0.05 U μL−1. These components were introduced simultaneously into the reaction system for different durations. In the case of the 0.5 h duration (lane 3), a small quantity of uncut HP/miRNA-21 double strands were detected. However, as the time progressed beyond 1 hour, a noticeable cleavage of the HP/miRNA-21 double strands by APE1 was observed. Hence, we concluded that the optimal reaction time for APE1 cleavage was determined to be 1 hour.
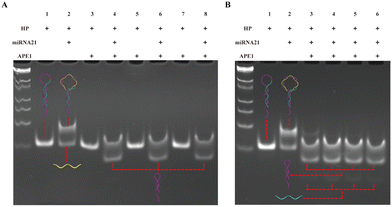 |
| Fig. 2 (A) 8% PAGE gel electrophoresis image for APE1 concentration optimization. Lane1/2: HP/HP + miRNA 21, without APE1; lane3/4: HP/HP + miRNA 21 with 0.05 U μL−1 APE1; lane5/6: HP/HP + miRNA 21 with 0.1 U μL−1 APE1; lane7/8: HP/HP + miRNA 21 with 0.15 U μL−1 APE1; (B) 8% PAGE gel electrophoresis image for APE1 enzyme cleavage time optimization. lane1:HP, lane 2:HP + miRNA-21, lane 3 to lane 6: HP + miRNA-21 + APE1 with different reaction time: lane 3, 0.5 h; lane 4, 1 h; lane 5, 1.5 h; lane 6, 2 h. | |
The second part is the RCA reaction initiated through the hybridization of HP1 with the dumbbell circular template DP. In Fig. 3A and D, as the concentration of Bst polymerase increased, the fluorescence intensity gradually increased and reached a state of equilibrium when reached 0.2 U μL−1. Then, we proceeded to fine-tune the concentration of dNTPs from 0.25 mM to 1 mM. As the dNTP concentration progressively increased, a corresponding gradual enhancement in fluorescence signal was observed. Notably, the optimal concentration of dNTPs was determined to be 0.5 mM (Fig. 3B and E). Subsequently, we undertook the optimization of the RCA reaction time. As the RCA reaction time increased, there was a noticeable and substantial increase in fluorescence signal enhancement. Particularly, a significant rise in fluorescence intensity was observed from 0.5 h to 2 h. Upon reaching a reaction time of 3 hours, the fluorescence intensity within the reaction system reached equilibrium (Fig. 3C and F). Hence, the optimal RCA reaction time was determined to be 3 hours.
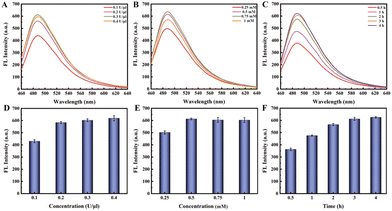 |
| Fig. 3 (A and D) Optimizing the concentration of Bst polymerase; (B and E) optimizing the concentration of dNTPs; (C and F) optimizing the reaction time of rolling circle amplification. | |
In the third phase of this experiment, the G-rich DNA products generated from the RCA process could assemble into multiple G-quadruplex repeat units in the presence of K+. Subsequently, ThT molecules could intercalate into these G-quadruplex structures, leading to fluorescence emission. We optimized the concentrations of both KCl and ThT (Fig. 4). Intriguingly, it revealed that the varying concentration of KCl, ranging from 5 mM to 40 mM, had no significant impact on the fluorescence intensity. Then, we focused on optimizing the concentration of ThT, and observed a distinct trend in the concentrations range of 5 μM to 50 μM. As the ThT concentration increased, fluorescence intensity substantially rose until it reached a state of equilibrium at 40 μM.
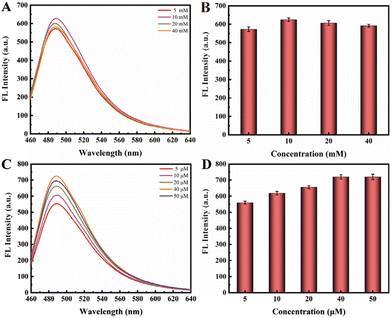 |
| Fig. 4 (A and B) Optimizing the concentration of KCl in the range of 5 mM to 40 mM; (C and D) optimizing the concentration of ThT in the range of 5 μM to 50 μM. | |
3.3 Analysis performance of the biosensor
Under the above optimal experimental conditions, the tumor marker miRNA-21 as the target with different concentrations were added into the designed cascade signal-amplified fluorescent biosensing system for verifying its analysis performance. As depicted in Fig. 5A, the fluorescence spectrum effectively illustrates the correlation between different target concentrations and the resulting ThT fluorescence signal. As anticipated, there is a discernible increase in the ThT fluorescence signal as the target concentration escalates. The logarithm of the target concentration clearly indicates that our biosensor exhibits a good linear relationship across a wide range of 0.1 nM to 100 nM (inset of Fig. 5B). The corresponding linear equation is Y = 134.916X + 377.586 (R2 = 0.998), where Y represents the fluorescence intensity and X represents the logarithm of the target concentration. The detection limit of this biosensor was calculated to be 3.33 pM, demonstrating our constructed fluorescent biosensor with cascade signal amplification strategy can achieve highly sensitive detection of target miRNA-21 with a wide linear range, and compared with other recently reported methods, its analytical performance exhibits a certain superiority (Table S2†), and hence provides a potential approach for tumor detection in clinical diagnosis. However, it should be noted that the limitation of our biosensor is that due to the RCA operation, it is not suitable for in vivo imaging, but only for in vitro detection, and the detection time is slightly longer. Hence, how to accelerate the detection process and shorten the detection time and further improve the sensitivity would be the challenges when considering this approach for practical applications.
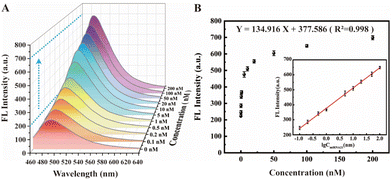 |
| Fig. 5 (A) Fluorescence spectra measurements after the addition of different concentrations of miRNA-21; (B) a curve illustrating the correlation between the level of miRNA-21 and the corresponding fluorescence intensity. Inset shows the linear range between the logarithm of miRNA-21 concentration and fluorescence intensity. | |
3.4 Specificity of the proposed biosensor
As a biological detection method with promising clinical applications, achieving not only high sensitivity but also robust specificity is crucial. In light of this, we conducted a comprehensive assessment of the selectivity and specificity of our proposed cascade signal-amplification biosensor in discerning the target miRNA-21 from other potentially interfering miRNAs. In our evaluation, all miRNAs, including the target miRNA-21, as well as miRNA-155, miRNA-141, and let-7a, were examined at an identical concentration of 200 nM. These miRNAs were tested both individually and in mixtures alongside miRNA-21. As showcased in Fig. 6A and B, a robust fluorescence signal was generated exclusively in the presence of the target miRNA-21. In contrast, when other tumor markers such as miRNA-155, miRNA-141, and let-7a were introduced, the fluorescence signal closely resembled that of the blank control group. The mixture, comprising all four miRNAs including miRNA-21, also exhibited discernible fluorescence signals that were similar to those generated when only miRNA-21 was present. These findings underscore the pivotal role of the target miRNA-21 in initiating the cascade of events. These outcomes highlight the biosensor's strong specificity, suggesting its potential application in clinical diagnosis.
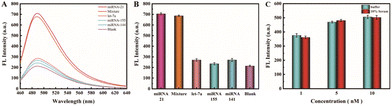 |
| Fig. 6 (A and B) Selectivity and specificity analysis of the proposed cascade signal-amplified fluorescence biosensors for miRNA 21. All miRNA samples were examined at an identical concentration of 200 nM. The mixture contains miRNA 21 target and three interfering miRNAs, each at a concentration of 200 nM. (C) Practical samples assessments of the developed fluorescence biosensors in PBS buffer and 10% serum samples. | |
3.5 Real sample analysis
To validate the biosensor's detection capability in real samples, we acquired samples of normal human serum and introduced them into the biosensor detection system. As depicted in Fig. 6C, the experimental group encompassed samples containing 10% serum, while the control group consisted of the reaction buffer solution. Various concentrations of miRNA-21 (1 nM, 5 nM, 10 nM) were added to each group. Notably, we observed no substantial difference in fluorescence intensity between the control group and the experimental group. As the concentration of the target miRNA-21 increased, the fluorescence signal intensity demonstrated an upward trend in both groups. This evidence suggests that the biosensor exhibits considerable potential for clinical applications.
4. Conclusion
In summary, we have successfully developed a highly sensitive fluorescent biosensor by employing a cascade signal amplification strategy. This biosensor relies on both APE1 enzyme excision target recycling and a rolling circle amplification reaction to detect miRNA-21. The unique feature of our biosensor is its utilization of tumor-endogenous miRNA-21 and the APE1 enzyme as dual triggers in the initial amplification step, which then initiates the second rolling circle amplification reaction. This dual signal amplification approach significantly enhances the sensitivity of this biosensor, achieving a detection limit as low as 3.33 pM. Furthermore, this biosensor demonstrates excellent specificity and anti-interference ability. It can effectively distinguish and detect the target from a mixture containing multiple interference miRNAs. Notably, the biosensor maintains its good detection capability in 10% serum environment, reliably identifying targets at varying concentrations. These results underscore the potential clinical applications of this study in disease diagnosis.
Author contributions
Zirui Liu: conceptualization, methodology, formal analysis, investigation, writing. Hongqun Yang: data curation, formal analysis, writing. Beibei Zhang: data curation, writing. Xinhao Li: analysis, review. Hong Wang: formal analysis, writing & review. Yingwei Zhang: project administration, funding acquisition, analysis, supervision, writing – review & editing.
Conflicts of interest
There are no conflicts to declare.
Acknowledgements
The authors thank the supports from Natural Science Foundation of China (grants 52072024, 51672022).
References
- Z. C. Wang, W. J. Xie and H. Z. Guan, Biomed. Pharmacother., 2023, 157, 114031–114046 CrossRef.
- H. Pietrykowska, I. Sierocka, A. Zielezinski, A. Alisha, J. C. Carrasco-Sanchez, A. Jarmolowski, W. M. Karlowski and Z. S. Kulinska, J. Exp. Bot., 2022, 73, 4528–4545 CrossRef.
- J. Shen, N. W. Todd, H. Zhang, L. Yu, X. L. Xiao, Y. P. Mei, M. Guarnera, J. P. Liao, A. Chou, C. L. Lu, Z. G. Jiang, H. B. Fang, R. L. Katz and F. Jiang, Lab. Invest., 2011, 91, 579–587 CrossRef PubMed.
- L. Mulrane, S. F. McGee, W. M. Gallagher and D. P. O'Connor, Cancer Res., 2013, 73, 6554–6562 CrossRef PubMed.
- K. K. Shukla, S. Misra, P. Pareek, V. Mishra, B. Singhal and P. Sharma, Urol. Oncol., 2017, 35, 92–101 CrossRef.
- C. Song, W. H. Chen, J. Y. Kuang, Y. Yao, S. Tang, Z. Zhao, X. J. Guo, W. Shen and H. K. Lee, Trends Anal. Chem., 2021, 139, 116269–116287 CrossRef CAS.
- M. G. Xu, X. M. Wang, J. J. Tian, J. Chen, X. J. Wei and W. Li, Sens. Actuators, B, 2022, 367, 132151–132162 CrossRef CAS.
- Y. T. Jiang, X. Y. Ma, X. J. Shao, M. Y. Wang, Y. Jiang and P. Miao, Sens. Actuators, B, 2019, 297, 126788–126795 CrossRef CAS.
- F. Y. Li, G. Li, S. J. Cao, B. S. Liu, X. L. Ren, N. Kang and F. Qiu, Biosens. Bioelectron., 2021, 172, 112757–112766 CrossRef CAS PubMed.
- R. F. Cai, S. X. Zhang, L. Chen, M. L. Li, Y. T. Zhang and N. D. Zhou, ACS Appl. Mater. Interfaces, 2021, 13, 4905–4914 CrossRef CAS PubMed.
- J. Ge, Y. Hu, R. J. Deng, Z. H. Li, K. X. Zhang, M. L. Shi, D. Yang, R. Cai and W. H. Tan, Anal. Chem., 2020, 92, 13588–13594 CrossRef CAS PubMed.
- X. Liu, H. Yang, Z. H. Xu, R. N. Liu, H. Zuo, Z. F. Chen, X. Y. Wang, C. Y. Xia and Y. X. Zhang, Biosens. Bioelectron., 2022, 209, 114185–114194 CrossRef CAS.
- B. Demple and J. S. Sung, DNA Repair, 2005, 4, 1442–1449 CrossRef CAS.
- Z. X. Li, X. Y. Feng, W. P. Hu and L. L. Li, Nanoscale, 2022, 14, 6465 RSC.
- X. M. Zhou, Y. Zhuo, T. T. Tu, R. Yuan and Y. Q. Chai, Anal. Chem., 2022, 94, 8732–8739 CrossRef CAS.
- Z. H. Cai, A. Wang, Y. Wang, Z. L. Qiu, Y. T. Li, H. R. Yan, M. Y. Fu, M. Y. Liu, Y. Y. Yu and F. L. Gao, Anal. Chem., 2022, 94, 9715–9723 CrossRef CAS.
- Y. Zhao, J. Zhao, J. F. Zhang, Y. Sun, L. L. Li, Z. P. Li and M. Y. Li, Anal. Chem., 2022, 94, 8883–8889 CrossRef CAS PubMed.
- X. C. Wang, J. T. Meng, H. P. Zhang, J. Y. Mou, J. P. Xiong, H. Wang, X. Su and Y. W. Zhang, Sens. Actuators, B, 2023, 381, 133425–133433 CrossRef CAS.
- Q. N. Kou, L. Wang, L. H. Zhang, L. Ma, S. N. Fu and X. Su, Small, 2022, 18, 2205191–2205201 CrossRef CAS PubMed.
- Z. T. Fan, J. Zhao, X. Chai and L. L. Li, Angew. Chem., Int. Ed., 2021, 60, 14887–14891 CrossRef CAS PubMed.
- Q. L. Wen, D. D. Li, G. D. Huang, H. Xi, H. C. Pan, L. M. Zhang, Z. Y. Li, X. F. Xiao and W. Y. Zhu, Analyst, 2022, 147, 4080–4085 Search PubMed.
- L. Zhang, S. W. Shi, P. H. Xiong, L. M. Chen, J. Xu, J. L. Jiang, S. L. Yang and H. X. Wu, Analyst, 2022, 147, 4158–4166 RSC.
- M. M. Ali, F. Li, Z. Q. Zhang, K. X. Zhang, D. K. Kang, J. A. Ankrum, X. C. Le and W. A. Zhao, Chem. Soc. Rev., 2014, 43, 3324–3341 RSC.
- X. D. Lai, X. Zhao, Y. N. Peng, R. Zhang, H. J. Pang, Z. J. Gao, D. X. Li, P. P. Cao, Q. M. Pu, B. Qiao, H. Pei and Q. Wu, Chem. Commun., 2023, 59, 10153–10156 RSC.
- J. Song, Y. Ju, S. Kim, H. Kim and H. G. Park, Chem. Commun., 2022, 58, 6518–6521 RSC.
- Y. Deng, T. C. Zhou, Y. Peng, M. H. Wang, L. L. Xiang, Y. Y. Zhang, J. L. Li, J. Yang and G. X. Li, Anal. Chem., 2023, 95, 3358–3362 CrossRef CAS.
- J. T. Yi, T. T. Chen, J. Huo and X. Chu, Anal. Chem., 2017, 89, 12351–12359 CrossRef CAS PubMed.
- S. J. Zhen, X. Xiao, C. H. Li and C. Z. Huang, Anal. Chem., 2017, 89, 8766–8771 CrossRef CAS PubMed.
- M. Hosseini, A. Akbari, M. R. Ganjali, M. Dadmehr and A. H. Rezayan, J. Fluoresc., 2015, 25, 925–929 CrossRef CAS.
- J. Su, F. B. Wu, H. P. Xia, Y. F. Wu and S. Q. Liu, Chem. Sci., 2020, 11, 80–86 RSC.
- Q. Xue and N. Hildebrandt, ACS Nano, 2015, 9, 8449–8457 CrossRef.
- L. Y. Duan, J. W. Liu, R. Q. Yu and J. H. Jiang, Biosens. Bioelectron., 2021, 177, 112976–112984 CrossRef CAS PubMed.
- X. F. Liao, N. Luo, M. Y. Li, F. Hao and L. Zou, Sens. Actuators, B, 2023, 381, 133459–133464 CrossRef CAS.
- X. Peng, J. Yang, W. B. Liang, Y. D. Sun, X. C. Mei, G. H. Zhang, R. Yuan and Y. C. Li, Sens. Actuators, B, 2022, 369, 132327–132337 CrossRef CAS.
- S. Li, Q. Dong, Y. Yu, B. X. Lin, L. Zhang, M. L. Guo, Y. J. Cao and Y. M. Wang, Anal. Chem., 2022, 94, 6711–6718 CrossRef CAS.
- M. X. Sun, B. R. Liu, T. Li, C. R. Li, W. J. Duan, B. P. Xie, M. M. Li, J. X. Chen, Z. Dai and J. Chen, Chem. Commun., 2022, 58, 11863–11866 RSC.
- J. Liu, G. Y. Xie, S. D. Lv, Q. Xiong and H. Y. Xu, Trends Anal. Chem., 2023, 160, 116953–116970 CrossRef.
|
This journal is © The Royal Society of Chemistry 2024 |
Click here to see how this site uses Cookies. View our privacy policy here.