Pseudo-equilibrium equations for calcium phosphate precipitation with multi-unit particles†‡
Received
2nd August 2023
, Accepted 13th November 2023
First published on 14th November 2023
Abstract
The chemistry underlying bone mineral formation in vertebrates is the reaction of calcium phosphate precipitation. In a near-neutral solution, an amorphous phase and hydroxyapatite nanoparticles appear successively, and the reaction system containing either of the two kinds of precipitates is in a non-equilibrium state. Here, we propose a pseudo-equilibrium approach to the solution chemistry of the precipitation reactions. We employed two series of reaction systems, collected samples at various stages, and analyzed the solution chemistry data on the basis of a simplified model of reaction. We derived two types of pseudo-equilibrium equations from the two series, respectively. These equations reveal the existence of multiple structural units in a precipitate particle and correlate the ionic product with the surface proportion per structural unit (m). The surface proportion, in turn, is related to the whole particle through a particle-surface equation. Notably, the two types of pseudo-equilibrium constants have the common expression of “Kd = ionic product” if the number of the structural units (u) is large enough. Together, these findings have revealed some aspects of the non-equilibrium thermodynamics of precipitation reactions, indicating the solution chemistry route to the equilibrium state. The concept of the multi-unit particle may shed new light on the study of precipitation reactions of other slightly soluble electrolytes. And the relationship between the ionic product and the surface proportion of a structural unit is not only fundamental in chemistry, but may also apply to non-equilibrium systems in nature and biology, such as marine sedimentation, human vascular calcification, and bone mineral metabolism.
1. Introduction
Calcium phosphates are the main inorganic components in the hard tissues of vertebrates. For example, the mineral in human bone is an analog of hydroxyapatite (HAP, Ca10(OH)2(PO4)6). In the form of nanocrystals, the bone mineral contains numerous impurities (CO32−, HPO42−, and Mg2+) and vacancies (missing OH−), yielding lower crystallinity and higher solubility than geologic apatite.1,2 Amorphous calcium phosphate (ACP) is the second type of calcium phosphate found in bone mineral. Shortly after the discovery of synthetic ACP in the 1960s,3 Posner et al. reported its presence in human and rat bones on the basis of X-ray diffraction intensity4,5 and infrared spectrophotometric analysis.6 They suggested that ACP is the precursor phase to HAP in bone formation, and its amount decreases with age and maturation.5–7 Some other researchers failed to detect the amorphous phase in even embryonic chick bone,8 though they later recognized the possibility of a “transient intermediate” that differs from the “sufficiently stable and long-lived” ACP originally proposed by Posner et al.9 In 2006, Crane et al. detected octacalcium phosphate (and possibly ACP) in mice nascent bone by Raman spectroscopy,10 which is considered strong evidence for the transient precursor strategy in bone mineral formation.11 However, taking into account the large specific surface area of HAP nanocrystals, the opposing side argued that it could be “the non-apatitic phosphates on the surface of the newly forming apatite mineral”.12 Further evidence came in 2008 when Mahamid et al. demonstrated the presence of ACP in the forming fin bones of zebrafish by multiple techniques, including high-resolution scanning and transmission electron microscopy imaging.13 But the opposing side insisted that it could be identified as very poorly crystalline calcium phosphate, stating “the theory that a solid amorphous precursor mineral phase is formed prior to the formation of a crystalline mineral phase has not been substantiated in bone.”14 Nevertheless, the decades-long debate appears to have settled with a flurry of new evidence emerging in recent years.15–17 Anyway, ACP and HAP nanoparticles are the focus of bone mineral research.
The chemistry underlying bone mineral formation is the reaction of calcium phosphate precipitation, for which the equilibrium constant is the solubility product (Ksp). In the expression of Ksp, the activity of the pure solid is considered as 1. But the reaction system containing either ACP or HAP nanoparticles is in a non-equilibrium state. Then, is the concept of Ksp applicable to such precipitates?
HAP is the least soluble calcium phosphate in aqueous solution at a near-neutral pH. Eqn (1) and (2) show the formation and the relevant Ksp expression, respectively, where the formula Ca10(OH)2(PO4)6 represents the chemical composition of one crystallographic cell. The number of unit cells (u) must be sufficiently large for the material to be solid. To indicate the multiple units of the crystalline particle, we rewrite eqn (1) as eqn (3). If the u value is not large enough, the particle fails to fulfill the criterion for a “pure solid”, as is the case with HAP nanoparticles. Formed from an aqueous medium, such a nanoparticle is coated by a surface layer18,19 that differs from the bulk crystal in composition and structure.18–22 The layer is also known as the “diffusion layer”,23 “non-apatitic hydrated layer”,24,25 or “interfacial phase”,26etc. A recent review has presented the amorphous nature of such layers.27 As observed by ultrahigh-resolution transmission electron microscopy, there is “an amorphous layer 1–2 nm thick”18 on the crystal surface. The Ca/P molar ratio at the surface is lower than that of the bulk crystal and, thus, rather “calcium deficient”.28 A factor accounting for the lower Ca/P value is the presence of HPO42− at the surface,19,22,29,30 which is the most abundant phosphate species in a near-neutral medium. Since the form of the lattice ion (PO43−) differs from its corresponding species in the solution (HPO42−), the chemical composition of the surface layer, under the impact of the solution, differs from the bulk crystal. Given the large specific surface area of a nanoparticle, the amount of the entities in the surface layer is comparable to that of the bulk crystal. Therefore, a HAP nanoparticle is a mixture of the surface phase and the bulk phase, rather than “a pure solid” required for the application of Ksp. The size effect on the solubility of calcium phosphate crystals has been analyzed.31 As Best noted in 1959: “Under such circumstances the solubility product will not be defined, a conclusion in agreement with the discrepant results of the various attempts to measure such a quantity experimentally.”26
|
10Ca2+ + 2OH− + 6PO43− = Ca10(OH)2(PO4)6
| (1) |
|
Ksp = [Ca2+]10[OH−]2[PO43−]6
| (2) |
|
 | (3) |
ACP is a metastable precursor to HAP,32 which has been observed to appear in a few seconds upon mixing the calcium and phosphate solutions at millimolar levels.33 The amorphous precipitate is known as the aggregate particles of calcium phosphate clusters in solution,34,35 “having variable chemical but identical glass-like, physicochemical properties”.29 Because every kind of calcium phosphate cluster in the solution has a chance to be incorporated into an aggregate particle, the chemical composition of ACP is determined by all kinds of clusters that are dependent on the solution pH.36 In the aqueous medium at a near-neutral pH, the dominant cluster Ca(HPO4) is the main constituent of the incipient ACP.33,37–39 A recent report demonstrated the preparation of amorphous Ca(HPO4) from near-neutral solutions.40 And the precipitates with Ca/P values close to 1.0 were detected from solutions with variable Ca/P ratios.41 Other reported clusters include Ca3(PO4)2,42 [Ca(HPO4)1+x·nH2O]2x− and [Ca(HPO4)·mH2O],43 Ca6(PO4)4 and Ca6(HPO4)3(PO4)2,36 and [Ca(HPO4)3]4−,44etc. Moreover, the formation of Ca(HPO4)22− is favored in the solution with excess phosphate ions.45 Posner's cluster with the formula Ca9(PO4)6 was originally proposed to appear in solutions,34 but it is more likely to occur at the late stage of ACP maturation.38 By analogy with the Ksp of a sparingly soluble electrolyte, early investigators determined the “equilibrium constants” for ACP with expressions like these: Ksp = [Ca][PO4]0.74[H]0.22,46Ksp = [Ca]3[PO4]1.87[HPO4]0.2,32 and Ksp = [Ca][PO4](2−2y)/3[HPO4]y for a kind of protein-sequestered ACP,47 to name a few. However, given the heavy hydration as indicated by the disk-shaped particles,48 an incipient ACP particle is more like “a droplet of concentrated cluster solution” than a pure liquid or solid, which is likely to contain more than one type of cluster under most circumstances. Therefore, ACP does not fulfill the criterion for the application of Ksp. In actuality, we have previously observed the association between the ionic product and the amount of the precipitate,49 and later derived a pseudo-equilibrium equation that relates the ionic product to the surface proportion of a precipitate particle.38
The present study aimed to propose a pseudo-equilibrium approach to the solution chemistry of precipitation reactions, exploring the non-equilibrium thermodynamics of the reaction systems with the precipitate that is not a “pure solid”. We employed two series of reaction systems, analyzed the solution chemistry data on a simplified model of the reaction, and derived two types of pseudo-equilibrium equations from the two series, respectively. These equations reveal the existence of multiple structural units in a precipitate particle and relate the ionic product to the surface proportion of a structural unit, indicating the solution chemistry route to the equilibrium state. Overall, our findings have extended the understanding of the precipitation reactions at equilibrium to those at non-equilibrium. The concept of the multi-unit particle and the pseudo-equilibrium approach may shed new light on the solution chemistry of precipitation reactions of other slightly soluble electrolytes. Furthermore, the relationship between the ionic product and the surface proportion of a structural unit in a precipitate particle is not only fundamental in chemistry, but may also apply to the non-equilibrium systems in nature and biology, such as marine sedimentation, human vascular calcification, and bone mineral metabolism.
2. Experimental
2.1 Materials and solutions
CaCl2·2H2O and Na2HPO4 were products of Sigma-Aldrich (Saint Louis, Missouri, USA). The standard solutions for calibrating the pH electrode were from Mettler Toledo (Switzerland). High purity HNO3 was from Crystal Clear Chemical Co., Ltd (UPS Grade, 68.0–70.0%, Suzhou, China). Other reagents (NaCl, HCl, and NaOH) were of analytical grade or higher.
Stock solutions of CaCl2 (20 mmol L−1 in 150 mmol L−1 NaCl, pH 6.6–7.0) and Na2HPO4 (20 mmol L−1 in 150 mmol L−1 NaCl, pH 8.00 ± 0.05) were freshly prepared with deionized water and their pH values were adjusted with NaOH (0.1 and 1.0 mol L−1) and HCl (0.1 and 1.0 mol L−1).
Working solutions were prepared according to Table 1, using their respective stock solutions and NaCl (150 mmol L−1). We prepared solutions for the two series (Series CPn and Series pH), each with five reaction systems. The pH values of the solutions were checked and adjusted to the desired values with NaOH (0.1 mol L−1) and HCl (0.1 mol L−1). The solutions were passed through 100 nm pore-size syringe filters (Minisart®, Sartorius Stedim, Germany) immediately before use.
Table 1 Working solutions for the two series of reaction systems (in 150 mmol L−1 NaCl)
Series |
System |
CaCl2 (mmol L−1) |
Na2HPO4 (mmol L−1) |
CPn |
3.0 |
6.00 |
|
6.00 |
|
3.5 |
7.00 |
|
7.00 |
|
n = 4.0 |
8.00 |
pH = 6.6–7.0 |
8.00 |
pH = 8.00 ± 0.05 |
4.5 |
9.00 |
|
9.00 |
|
5.0 |
10.0 |
|
10.0 |
|
|
pH |
7.6 |
8.00 |
|
8.00 |
7.60 |
8.0 |
8.00 |
|
8.00 |
8.00 |
pH = 8.5 |
8.00 |
pH = 6.6–7.0 |
8.00 |
pH = 8.50 ± 0.05 |
9.0 |
8.00 |
|
8.00 |
9.00 |
9.5 |
8.00 |
|
8.00 |
9.50 |
2.2 Measurement of pH drift
Working solutions were thermostated at 25 ± 0.2 °C in a water bath before use. The reaction was initiated by mixing equal volumes (40 mL) of the calcium and phosphate working solutions in a 100 mL beaker. The beaker was kept at 25 ± 0.2 °C in a water-jacketed Pyrex cell. The mixture in the beaker was magnetically stirred at a speed of 20% (instrument scale) and purged with nitrogen gas (N2 99.999%, Huanyu Jinghui Gas Co., Beijing, China) to reduce the effect of atmospheric CO2. The pH value was recorded at a frequency of 2 pts per min using a SevenExcellenceTM instrument (Mettler Toledo, Switzerland). A 3-point (pH 4.01, 7.00, 9.21) calibration for pH was performed immediately before a pH drift experiment.
2.3 Sample collection and chemical analyses
Samples were collected from the reaction system at the scheduled time points for the determination of Ca and P elements. Typically, we drew 2.5 mL of suspension from the middle of the beaker and centrifuged it immediately at 6000 × g for 4 min (HC-3018, Zhongjia Co., Anhui, China). From the upper part of the centrifuge tube, 0.8 mL of the supernatant was drawn and acidified with 0.2 mL of 3% HNO3, designated as sample A. An aliquot (0.1 mL) of sample A was diluted with 9.9 mL of 3% HNO3. The mixture was designated as sample B, which was used for the determination of the Ca content by inductively coupled plasma optical emission spectrometry (ICP-OES) on an ICAP-6300 instrument (Thermo Fisher, UK). The remaining sample A was used for the determination of P content by spectrophotometry on an AU5800® Chemistry Analyzer (Beckman Coulter, Inc.). The determined values of the standard solutions were used for calibrating the sample values. The phosphate content in the precipitate (SP) was calculated using the equation: SP = tPi (total concentration) − LPi (concentration in the supernatant). The Ca content in the precipitate was determined similarly: SCa = tCa − LCa.
3. Results and discussion
We are to adopt a pseudo-equilibrium approach to the solution chemistry of precipitation reactions and to reveal some aspects of the non-equilibrium thermodynamics. Notably, a reaction system at pseudo-equilibrium differs from that at equilibrium in some aspects. In the reaction of calcium phosphate precipitation, the only equilibrium state of a system is characterized by the unique “solubility product (Ksp)”, whose expression and value are independent of the initial concentrations of reactants. Whereas a system may have multiple pseudo-equilibrium states on the course toward the equilibrium, each with its specific expression of pseudo-equilibrium constant. Therefore, it is essential to collect samples from a series of reaction systems in a common pseudo-equilibrium state in the present study.
3.1 Two series of reaction systems
To meet the aforementioned requirement, we employed two series of reaction systems: Series CPn and Series pH. The pH drift curves of the two series display similar shapes (Fig. 1): a mild decrease (“induction period”) followed by a rapid change that concludes in about 10 minutes. Based on the shape of a pH drift curve, eight sampling times were set. The primary one was the completion of the rapid change (CRC, indicated with an arrow, Fig. 1a), which was readily recognized as the separating point of the tangent to the slow change period. During the induction period before the rapid change, we collected samples at its early, middle, and late stages, respectively (Ind-1, -2, and -3, indicated with asterisks, Fig. 1a). Among them the Ind-3 sample was collected at least 4 min before the onset of the rapid change period, to allow time for the manipulation of subsequent centrifugation. After CRC was a crystal ripening process,38,50 in which we collected samples at 1, 3, 6, and 12 hours after CRC (designated as 1H, 3H, 6H, and 12H, respectively). At some sampling times, the first and last systems made less and more reaction progress, respectively, than the others. Nevertheless, at least three systems were in a common pseudo-equilibrium state in such circumstance.
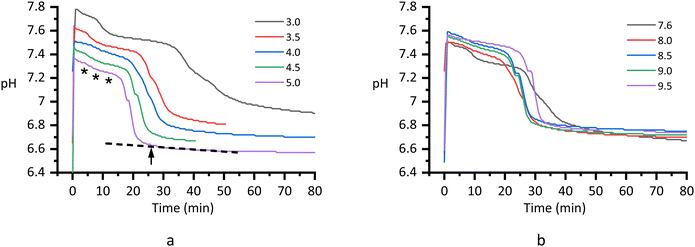 |
| Fig. 1 The pH drift curves of the two series of reaction systems. (a) Series CPn: a reaction was initiated by mixing equal volumes of calcium (pH 6.60–7.00) and phosphate (pH 8.00 ± 0.05) solutions. The initial concentrations in the mixture were [Ca2+]i = [Pi]i = n = 3.00, 3.50, 4.00, 4.50, and 5.00 mmol L−1, respectively. The arrow and asterisks signify some of the sampling times. (b) Series pH: the initial concentrations in the mixture were [Ca2+]i = [Pi]i = 4.00 mmol L−1, and the phosphate solutions were pre-adjusted to pH 7.60, 8.00, 8.50, 9.00, and 9.50 (±0.05), respectively. | |
Series CPn (Fig. 1a) is an improvement on series CnP5 investigated in our previous work ([Pi]i = 5.00 mmol L−1 and [Ca2+]i = 2.50, 3.00, 3.50, 4.00, 4.50 mmol L−1, respectively).38 In the current series, more significant differences were observed between systems in both the ionic product and the amount of the precipitate, yielding a higher signal/noise ratio of the solution chemistry data.
Series pH (Fig. 1b) differs from the above series in that the ionic product decreased as the amount of the precipitate increased from system to system. Besides, the pH level in the induction period varied non-monotonically. This fact reflects the dual effects of the initial pH on the reaction. In a phosphate solution, a higher pH corresponds to a higher HPO42−/H2PO4− ratio. On the one hand, the increase of HPO42− ions caused more CaHPO4 clusters to form and reduced the pH value. On the other hand, the higher concentration of the remaining HPO42− helped resist the reaction-induced pH drop. These two opposite effects resulted in the non-monotonic pH change in the induction period.
3.2 Two types of pseudo-equilibrium equations
Here we present a simplified model of the reaction (Scheme 1), to make the data analysis simpler while still retaining the critical aspects of the reaction. A more detailed explanation can be found in the ESI.‡
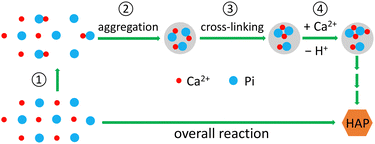 |
| Scheme 1 Calcium phosphate precipitation via aggregation-facilitated cross-linking. | |
Inorganic phosphate (Pi) is present mainly as H2PO4− and HPO42− in the near-neutral medium. They bind Ca2+ ions, forming CaH2PO4+ and CaHPO4 (step ①, Scheme 1), respectively. Given the H2O molecules in the coordination sphere of a Ca2+ ion,37,51 CaH2PO4+ and CaHPO4 could be called “calcium phosphate clusters”. These solution clusters form aggregate particles (step ②, Scheme 1), known as ACP. Because the clusters in an aggregate particle are closer to each other than those in solution, the cross-linking among them takes place more readily (step ③, Scheme 1). Some of the cross-linked clusters get additional Ca2+ ions, and the increased positive charges facilitate the proton release from a Ca2+-bound HPO42− ion, leading to the generation of Ca2+-bound PO43− ions (step ④, Scheme 1).
The overall reaction is shown in eqn (4), where x and (1 − x) represent the CaHPO4 proportions derived from H2PO4− and HPO42−, respectively; y and (1 − y) represent the PO43− and HPO42− proportions in the precipitate, respectively. Using the numbers of Ca and P atoms in the formula Ca(1−y)m0(HPO4)(1−y)m0Ca1.5ym0(PO4)ym0, we relate y with the experimentally determined Ca/P molar ratio (r, eqn (5)). Then the overall reaction can be written as eqn (6), where the formula Carm0(HPO4)(1−y)m0(PO4)ym0 represents a cross-linked cluster that exists as the structural unit of a precipitate particle, and m0 denotes the phosphate number in the cluster. The formula is equivalent to Carm0(H)(1−y)m0(PO4)m0 that becomes Ca10(OH)2(PO4)6 if m0 = 6, r = 5/3, and (H)−2 = (OH)2. Following the convention of presenting precipitation reactions, we express the dissociation constant in eqn (7), where (IP)m0 and [Sm0] represent the ionic product and the precipitate concentration in terms of the structural unit, respectively. To indicate the multiple structural units in a particle, we rewrite eqn (6) as eqn (8). For now, however, we focus on the reaction concerning the structural unit (eqn (6)). But one should be aware that the structural unit is within an aggregate particle, rather than a discrete one dispersed in solution.
|
rm0Ca2+ + (1 − x)m0HPO42− + xm0H2PO42− = (x + y)m0H+ + Ca(1−y)m0(HPO4)(1−y)m0Ca1.5ym0(PO4)ym0
| (4) |
|
rm0Ca2+ + (1 − x)m0HPO42− + xm0H2PO42− = (x + y)m0H+ + Carm0(HPO4)(1−y)m0(PO4)ym0
| (6) |
|
 | (7) |
|
 | (8) |
The concentration [Sm0] in eqn (7) can be expressed in either the P or Ca content ([SP] and [SCa], eqn (9)). The corresponding ionic product (IP)m0 is defined as the function of (IP)0 (eqn (10)), where the exponential term m0 is a constant to be determined from the solution chemistry data; the square brackets indicate (pseudo)equilibrium concentrations in molarity, where the ion charges are eliminated for clarity. In the presence of 150 mmol L−1 NaCl, the reaction-caused change in the ion strength of the solution could be neglected. Thus, we use the numerical values of molarity in place of the dimensionless activities.
|
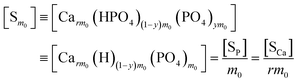 | (9) |
|
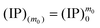 | (10a) |
|
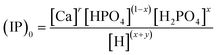 | (10b) |
By introducing eqn (9) and (10) into eqn (7), we obtain
|
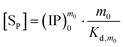 | (11) |
Taking the natural logarithm of eqn (11) gives
|
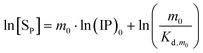 | (12) |
Similarly, we obtain the equations where [Sm0] is expressed in terms of [SCa]:
|
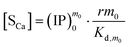 | (13) |
|
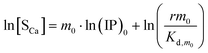 | (14) |
Shown in Fig. 2 are the ln[SP] vs. ln(IP)0 plots according to eqn (12), where a linear fitting is made to the data from at least three of the five reaction systems at a sampling time. These plots exhibit three prominent features. First, the presence of linearity indicates a correlation between the ionic product (IP)0 and the precipitate concentration [SP], different from the conventional solubility product (Ksp). We take the Ind-2 in Fig. 2a as an example: both (IP)0 and [SP] vary from system to system at the sampling time, but together they define a pseudo-equilibrium constant that can be determined from the intercept of the plot by eqn (12).
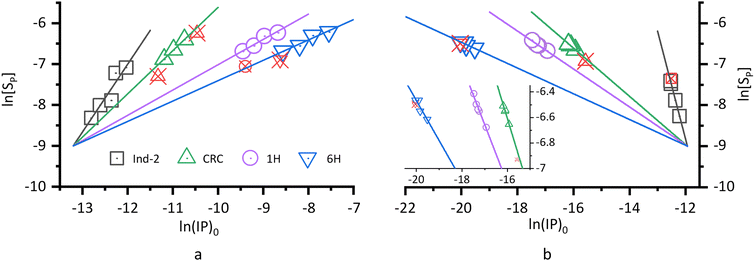 |
| Fig. 2 Correlation between the ionic product (IP)0 and the precipitate concentration [SP] at various reaction stages. (a) Series CPn: initial concentration [Ca2+]i = [Pi]i = n = 3.00, 3.50, 4.00, 4.50, and 5.00 mmol L−1, respectively; Pi pH = 8.00; (b) Series pH: initial concentration [Ca2+]i = [Pi]i = 4.0 mmol L−1; Pi pH = 7.60, 8.00, 8.50, 9.00, and 9.50, respectively. For comparison, the data of CRC, 1H, and 6H were shifted along the abscissa so that their fitted lines intersect that of Ind-2 at ln[SP] = −9.0. The aberrant points that deviated from the synchronicity (red and crossed) are excluded from the linear fitting. Inset in (b) shows the data from CRC onwards, where the differences are minimal from system to system at a sampling time. The fitted results of unshifted plots are filed in Table S1 of the ESI.‡ | |
Second, in either Fig. 2a or b, the absolute value of the slope of Ind-2 is greater than 1.5, whereas those from CRC onwards are mostly smaller than 1 and show a downward trend (column 5, Table 2). These results are consistent with our previous observations in another reaction series, and the downward trend has been considered characteristic of the crystal ripening process.38 In this process, the structural unit of a precipitate particle was a crystallographic cell. Because the phosphate number (m0) in a unit cell of HAP is an integer constant greater than 1, it does not match the decreasing value of the slope. On the other hand, the average number of surface phosphates per structural unit (m, eqn (15)) decreased with increasing structural units (u), as indicated by the particle-surface equation (eqn (16)38). Judging from the values and the downward trend, the surface parameter m matches the slope. Thus, the ionic product actually responded to the surface proportion per unit (eqn (17)), rather than to the complete structural unit (eqn (6)). This feature is different from the stoichiometry of the solubility product constant Ksp. Some differences between the equilibrium constant Ksp and a pseudo-equilibrium constant Kd,m are listed in Table 3.
|
 | (15) |
|
 | (16a) |
where
d = 3 is the dimension of the particle.
Table 2 Parameters relevant to the multi-unit particles in calcium phosphate precipitation
Stage |
r ± stdevb |
y |
y mod |
m ± stdeve |
m 0 |
rm 0 |
u ap |
Formulah |
K d,m ± stdevi |
1/Kd,m |
For the Ind data of series pH, the fitted results of an ln[SP] vs. ln(IP)0 plot are significantly different from those of the relevant ln[SCa] vs. ln(IP)0 plot (Table S1, ESI). The latter results are used here. Average of the Ca/P molar ratios of the precipitate separated from a series of reaction systems. The values during the induction period are assumed to be 1 (Section 3.2). P molar ratios eqn (5): y = 2(r – 1). Using the general formula Ca(1−y)m0(HPO4)(1−y)m0Ca1.5ym0(PO4)ym0 in eqn (4) and a modified value for y (i.e. ymod), one can determine the specific formula of the structural unit for the samples from CRC onwards (Section 3.5). We take the CRC in series CPn as an example: PO43− number = ymodm0 = 0.62 × 3 = 1.86 ≈ 2, HPO43− number = (1 − ymod)m0 = (1 − 0.62) × 3 = 1.14 ≈ 1, and Ca2+ number = [(1 − ymod) + 1.5ymod] m0 = (1 − 0.62 + 1.5 × 0.62) × 3 = 3.93 ≈ 4. Average of the m values obtained from the slopes of an ln[Sp] vs. ln(IP)0 plot and an ln[SCa] vs. ln(IP)0 plot. The determination of the apparent m0 value is discussed in Section 3.4 for ACP and in Section 3.5 for HAP nanoparticles. Calculated according to the particle-surface equation (eqn (16a)): u = (m0/m)d, where the particle dimension d = 3. Based on the general formula of the precipitate in eqn (8): {Carm0(HPO4)(1−y)m0(PO4)ym0}u. Average of the Kd,m values obtained from the intercepts of an ln[Sp] vs. ln(IP)0 plot and an ln[SCa] vs. ln(IP)0 plot. |
(a) Series CPn (n = 3.0, 3.5, 4.0, 4.5, 5.0) |
Ind-1 |
1 |
0 |
|
1.92 ± 0.05 |
2 |
2 |
1.1 |
Ca2(HPO4)2 |
(2.4 ± 1.4) × 10−7 |
4.09 × 106 |
Ind-2 |
1 |
0 |
|
1.64 ± 0.02 |
2 |
2 |
1.8 |
{Ca2(HPO4)2}2 |
(5.8 ± 0.7) × 10−6 |
1.72 × 105 |
Ind-3 |
1 |
0 |
|
1.97 ± 0.13 |
3 |
3 |
3.5 |
{Ca3(HPO4)3}3.5 |
(3.3 ± 3.1) × 10−7 |
3.03 × 106 |
CRC |
1.29 ± 0.06 |
0.58 |
0.62 |
1.05 ± 0.02 |
3 |
3.9 |
23 |
{Ca4(HPO4)(PO4)2}23 |
15.6 ± 0.8 |
6.43 × 10−2 |
1H |
1.34 ± 0.06 |
0.68 |
0.67 |
0.66 ± 0.04 |
3 |
4.0 |
94 |
{Ca4(HPO4)(PO4)2}94 |
189 ± 29 |
5.28 × 10−3 |
3H |
1.37 ± 0.04 |
0.74 |
0.67 |
0.65 ± 0.02 |
3 |
4.1 |
98 |
{Ca4(HPO4)(PO4)2}98 |
316 ± 19 |
3.16 × 10−3 |
6H |
1.35 ± 0.04 |
0.70 |
0.67 |
0.50 ± 0.00 |
3 |
4.1 |
216 |
{Ca4(HPO4)(PO4)2}216 |
410 ± 1 |
2.44 × 10−3 |
12H |
1.37 ± 0.07 |
0.74 |
0.76 |
0.57 ± 0.02 |
5 |
6.9 |
675 |
{Ca7(HPO4)(PO4)4}675 |
883 ± 39 |
1.13 × 10−3 |
|
(b) Series pH (pH = 7.60, 8.00, 8.50, 9.00, 9.50) |
Ind-1 |
1 |
0 |
|
2.72a |
3 |
3 |
1.3 |
Ca3(HPO4)3 |
4.02 × 10−19 a |
2.49 × 1018 |
Ind-2 |
1 |
0 |
|
2.42a |
3 |
3 |
1.9 |
{Ca3(HPO4)3}2 |
2.00 × 10−17 a |
4.99 × 1016 |
Ind-3 |
1 |
0 |
|
1.13a |
3 |
3 |
18.7 |
{Ca3(HPO4)3}19 |
2.13 × 10−10 a |
4.68 × 109 |
CRC |
1.36 ± 0.02 |
0.72 |
0.70 |
0.60 ± 0.01 |
3 |
4.1 |
125 |
{Ca4(HPO4)(PO4)2}125 |
(6.93 ± 0.20) × 10−5 |
1.44 × 104 |
1H |
1.35 ± 0.04 |
0.70 |
0.70 |
0.46 ± 0.00 |
3 |
4.1 |
277 |
{Ca4(HPO4)(PO4)2}277 |
(1.02 ± 0.02) × 10−4 |
9.83 × 103 |
3H |
1.36 ± 0.05 |
0.72 |
0.70 |
0.39 ± 0.02 |
3 |
4.1 |
455 |
{Ca4(HPO4)(PO4)2}455 |
(1.46 ± 0.09) × 10−4 |
6.84 × 103 |
6H |
1.36 ± 0.08 |
0.72 |
0.70 |
0.35 ± 0.08 |
3 |
4.1 |
630 |
{Ca4(HPO4)(PO4)2}630 |
(1.75 ± 0.45) × 10−4 |
5.70 × 103 |
12H |
1.40 ± 0.02 |
0.80 |
0.80 |
0.40 ± 0.01 |
5 |
7.0 |
1953 |
{Ca7(HPO4)(PO4)4}1953 |
(1.42 ± 0.02) × 10−4 |
7.06 × 103 |
Table 3 Comparison between the equilibrium constant Ksp and a pseudo-equilibrium constant Kd,m
Properties |
Equilibrium and Ksp |
Pseudo-equilibrium and Kd,m |
Unicity vs. multiplicity |
A reaction system has only one equilibrium state with its unique equilibrium constant Ksp. |
A reaction system may have multiple pseudo-equilibrium states on the course towards the equilibrium, each with its pseudo-equilibrium constant Kd,m. |
|
System dependency |
The expression of Ksp is determined by the formula of the precipitate, which is independent of the reaction systems employed. |
In an expression of Kd,m, the ionic product and the concentration of the precipitate take the form of product or quotient, depending on the reaction series employed. |
|
Precipitate |
The expression of Ksp takes the form of an ionic product, ignoring the contribution from the precipitate that, as a pure solid, has the activity of 1. |
An expression of Kd,m contains both the ionic product and the precipitate concentration. The precipitate cannot be taken as a pure solid. |
|
Whole vs. part (m) |
The expression of Ksp corresponds to the complete structural unit of a particle, as indicated by the precipitate formula. |
The ionic product in an expression of Kd,m responds to the surface proportion per structural unit in a multi-unit particle. |
|
Number of structural units in a particle (u) |
The expression and value of Ksp, seemingly not taking into account the number of the structural units in a precipitate particle though, implicitly assume that the number of the structural units in a particle is sufficiently large. |
The expression and value of Kd,m reflect the number of the structural units in a multi-unit particle, owing to the correlation between the ionic product and the surface proportion per structural unit. |
The third feature is the opposite signs of the slopes associated with the two series of reactions. The positive slope in Fig. 2a leads to Type I pseudo-equilibrium equation (eqn (18)), providing further evidence for our previous report.38 Whereas the negative slope in Fig. 2b leads to a Type II equation (eqn (19)), showing that the ionic product (IP)m decreased as the precipitate concentration [Sm] increased. The inverse relationship between [Sm] and (IP)m is a logical consequence for Series pH, given the fixed total concentrations of calcium and phosphate in the five reaction systems. However, the constant product of (IP)m × [Sm] is quite unexpected. After all, no equilibrium counterpart has been reported so far. As listed in Table 3, the dependency on the reaction series is one of the major differences between a pseudo-equilibrium constant (Kd,m) and the equilibrium constant (Ksp).
|
rmCa2+ + (1 − x)mHPO42− + xmH2PO4− = (x + y)mH+ + Carm(HPO4)(1−y)m(PO4)ym
| (17) |
|
 | (18) |
3.3 Reaction at the particle surface
In the two types of pseudo-equilibrium equations (eqn (18) and (19)), the subscript “m” indicates the surface proportion per structural unit in a multi-unit particle, as defined in eqn (15). Accordingly, the relevant precipitate concentration [Sm] should be used in the plots in Fig. 2. Why could the concentrations in terms of the complete unit ([Sm0]) make a plot linear? We now examine the relationship between [Sm] and [Sm0].
The ionic product and the precipitate concentration in terms of m are shown in eqn (20) and (21), respectively. Here [SP,surf] and [SCa,surf] represent the precipitate concentrations in terms of the P and Ca contents at the surface, respectively. According to the definitions of m (eqn (15)) and m0, the ratio of [SP,surf]/[SP] is equal to m/m0 (eqn (22)). We rearrange eqn (22) into eqn (23). Because the left- and right-hand sides of eqn (23) are equal to [Sm] and [Sm0] (eqn (21) and (9)), respectively, we obtain eqn (24).
|
 | (21) |
|
 | (22) |
|
 | (23) |
By substituting [Sm0] for the [Sm] in eqn (18), we then obtain eqn (25) for Series CPn, which shows that the concentration [Sm0] for the complete structural unit is indeed compatible with the (IP)m0 for the surface proportion. Taking the natural logarithm of eqn (25b) yields eqn (26), which is the exact equation governing the plots in Fig. 2a.
|
 | (25a) |
|
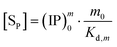 | (25b) |
|
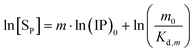 | (26) |
By substituting [Sm0] for the [Sm] in eqn (19), we obtain eqn (27) and (28) for Series pH, and the latter is the exact equation governing the plots in Fig. 2b.
|
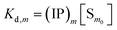 | (27a) |
|
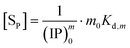 | (27b) |
|
ln[SP] = −m·ln(IP)0 + ln(m0Kd,m)
| (28) |
Similarly, we obtain the pseudo-equilibrium equations where the precipitate concentration is expressed in terms of its calcium content:
|
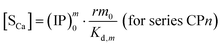 | (29) |
|
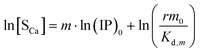 | (30) |
|
 | (31) |
|
 | (32) |
According to eqn (26) and (28), the slopes of ln[Sp] vs. ln(IP)0 plots give the m values (designated as mP) for Series CPn (Fig. 2a) and Series pH (Fig. 2b), respectively. Similarly, we obtain the m values (designated as mCa) from the ln[SCa] vs. ln(IP)0 plots according to eqn (30) and (32), respectively. Listed in Table 2 are the average values of mP and mCa at various reaction stages. Indeed, it is quite unexpected that the surface parameter m can be determined directly from the solution chemistry of the precipitation reaction, thus providing the evidence for Greenwald's conclusion drawn from crystal dissolution in 1942: “It is this surface and not the bulk of the precipitate that is believed to be in equilibrium with the solution.”52
Notably, the pseudo-equilibrium equations (eqn (25b), (27b), (29), and (31)) take the form of power laws (eqn (33)), where m is the magnitude of the characteristic exponent. As implied by the scale-invariant property of the function f(cx) ∝ f(x) (where c is a constant), a change in (IP)0 brought about a change in the quantity of the precipitate, while the composition and structure of the precipitate particles remained the same for the series of reaction systems at a common pseudo-equilibrium state. In the following sections, we will show how the experimentally determined surface parameter (m) is related to those of the complete structural unit (m0) and the whole particle (u, Kd,u).
3.4 Multi-unit particles: an amorphous phase
The determination of the m0 value for ACP is based on the surface parameter m. In the induction period, most of the m values were above 1.5 in both reaction series (column 5, Table 2), reflecting the characteristic composition and structure of ACP. In the aggregate particles, calcium phosphate clusters were highly hydrated.51 The hydration prevented them from getting closer to each other, resulting in less mutual occlusion. Therefore, we assume the m0 value to be the nearest integer greater than the m values, i.e. m0 = 2 for Series CPn and m0 = 3 for Series pH, respectively (column 6, Table 2). But a higher value (m0 = 3) is required for the Ind-3 in Series CPn, to match the greater extent of cross-linking as indicated by the increased m value.
Then, we calculate the apparent number of the structural units in a particle (uap, column 4 from right, Table 2) using eqn (16a). Here, by “apparent” we mean that there were likely more clusters in a particle, but a cluster was unable to fully “feel” the others as they were not close enough to each other. In this sense, the uap value is an indication of mutual occlusion. Using the m0 and u values, we arrive at the chemical formula of a multi-unit particle (column 3 from right, Table 2). In Series CPn, Ind-1 and Ind-2 have the same structural units, but Ind-2 has a higher uap value. Similarly, the uap value increases from Ind-1 through Ind-3 in Series pH. It is worth noting that, in addition to taking additional ions and clusters from the ambient solution, the dehydration-induced densification can also lead to an increase in uap. Such dehydration has been reported in the formation of a dense liquid phase, where the calcium and phosphate ions in the initial aggregates release their second shell H2O molecules.53
Next to the chemical formula is the pseudo-equilibrium constant Kd,m (column 2 from right, Table 2). With the m0 value known, a value of Kd,m can be derived from the intercept of an ln[S] vs. ln(IP)0 plot by eqn (26) and (30) for Series CPn and by eqn (28) and (32) for Series pH. Consistent with the parameter m, Kd,m also corresponds to the surface proportion of a structural unit. Nevertheless, it could be related to the pseudo-equilibrium constant for a whole multi-unit particle (Kd,u). Eqn (34) and (35) are the two types of pseudo-equilibrium equations for the overall reaction represented by eqn (8), where the precipitate concentration is expressed in terms of the multi-unit particle ([Su], eqn (36)). Alternatively, the concentration can be expressed in terms of a structural unit ([Sm0], eqn (37)). Dividing eqn (36) by eqn (37) gives eqn (38). According to eqn (24), we substitute [Sm] for the [Sm0] in eqn (38) and obtain eqn (39). Through the [Su] and [Sm] in eqn (39), we then introduce eqn (34) and (18), yielding eqn (40) that relates Kd,m to Kd,u for Series CPn.
|
 | (34) |
|
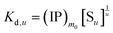 | (35) |
|
 | (36) |
|
 | (37) |
|
 | (38) |
|
 | (39) |
|
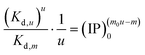 | (40) |
Here we take the Ind-2 in Series CPn as an example. Using eqn (40), we obtain Kd,u = 1.85 × 10−9 and Kd,u = 2.70 × 10−9 for the reaction systems n = 3.5 and 4.5 mmol L−1, respectively. These values are comparable to those calculated directly using eqn (34): Kd,u = 1.92 × 10−9 and Kd,u = 2.49 × 10−9 for the respective systems. Since Kd,m was determined from the data of five systems, the values from eqn (40) would be more representative than those from eqn (34). Therefore, the median of the former results, Kd,u = 2.3 × 10−9, could be taken as the dissociation constant of the ACP with the formula
, which represents one Ca2(HPO4)2 unit in a two-unit particle. Using the Kd,u value, we further calculate the free energy difference for the ACP formation by eqn (41): ΔGu = −49.3 kJ mol−1. Notably, the value is more than 3 times that of a discrete Ca2(HPO4)2 (−15.3 kJ mol−1) from a computer simulation,39 showing the additional stability conferred by the aggregation. For the whole particle with the formula {Ca2(HPO4)2}2, we obtain Kd,u = (2.3 × 10−9)2 = 5.3 × 10−18 and ΔGu = −98.5 kJ mol−1.
|
ΔG = −RT ln(1/Kd,u)
| (41) |
where
R = 8.31 J K
−1 mol
−1 and
T = 298 K.
The concepts of m0 and uap may shed new light on the formation and transformation of ACP. For example, as the values of m0 and uap increase, the sequential appearance of “solution clusters → dense liquid → amorphous solid” would be a natural consequence. The nascent dense liquid could be the droplets comprising aggregated, highly hydrated clusters, which do not create a tangible interface and are dispersed in the solution until being precipitated by centrifugation.49 Given the continuous change in the composition and structure of the precipitate,54 the term “amorphous calcium phosphate” is better used as a collective name for a class of materials rather than for a compound with a definite chemical formula. As such, the reported differences in the Ca/P ratio and the solubility of ACP could be attributed to the different initial solutions and/or the different stages of maturation.
3.5 Multi-unit particles: a crystalline phase
From CRC onwards, the values of the surface parameter m are significantly lower than those in the induction period (column 5, Table 2), consistent with the compactness of the structural units in a crystalline particle. For a HAP nanoparticle, the parameter m0 primarily represents the phosphate number in a crystallographic cell with the formula Ca10(OH)2(PO4)6, i.e. m0 = 6. However, the value is affected by the materials in the interfacial layer. At the crystal surface, the phosphate anions have some of their O atoms spread into the interfacial layer, and a proportion of them bind Ca2+ ions. Then reacting entities could develop from such sites at certain locations (e.g., steps, kinks) in the crystal ripening process. Hence, the precipitate formula {Carm0(HPO4)(1−y)m0(PO4)ym0}u in eqn (8) represents a mixture of the bulk crystal and the materials in the interfacial layer. We determined the value of the apparent m0 in the formula according to the following requirements: (1) to be an integer not less than m; (2) to make each of the numbers of Ca2+ (= rm0), PO43− (= ym0), and HPO42− (= (1 − y)m0) an integer or close to an integer; (3) to make the Ca2+ number obtained from rm0 equal or close to that obtained from the charge balance in the formula, i.e. 2rm0 = 3ym0 + 2(1 − y)m0. Since the value of y should not decrease in the crystal ripening process, a modified value (ymod, column 4, Table 2) is required in most cases.
The obtained value of m0 = 3 applies to the samples from CRC through 6H for both series, which leads to the formula Ca4(HPO4)(PO4)2 (column 3 from right, Table 2). Whereas the 12H samples demand a higher value of m0 = 5, which leads to the formula Ca7(HPO4)(PO4)4 with a lower HPO4/PO4 ratio than its predecessor. The two formulas appear in the same order as we reported previously.38 Note that the determination of the apparent m0 is strongly dependent on y, and the latter is derived from the Ca/P ratio r. Now we show how the r value is affected by the materials in the interfacial layer. As illustrated in eqn (42a) and (43a), each of the two formulas can be expressed as a mixture of HAP and the reacting entity 3Ca(HPO4)·2Ca3(PO4)2. To facilitate comparison, we have written the two formulas as 2Ca(HPO4)·2Ca3(PO4)2 and Ca(HPO4)·2Ca3(PO4)2, respectively. Two points can be drawn from these equations. First, the reacting entity has a lower r value than those of the precipitates. Second, the quantity of the reacting entity is smaller in eqn (43a) than in eqn (42a), indicating that the reaction was less active at 12H. If the reaction is close to the equilibrium and, thus, the concentrations of the reactants are sufficiently low, the quantity of the reacting entity would be negligible. In such circumstances, we use Ca(HPO4) to represent the material in the interfacial layer in eqn (42b) and (43b). In either case, the “surface proportion” includes the contributions from both the surface of the bulk crystal and the materials in the interfacial layer, and the higher m0 value for 12H is associated with the decreased quantity of the latter.
|
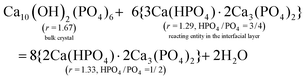 | (42a) |
|
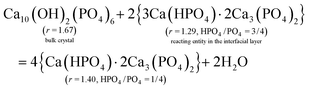 | (43a) |
|
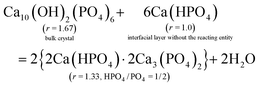 | (42b) |
|
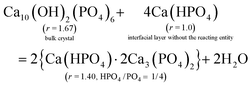 | (43b) |
With the m0 value known, we calculate the uap value according to eqn (16a). As shown in Table 2, the uap value is significantly higher for a set of samples in Series pH than the corresponding one in Series CPn, indicating that the higher pH values promoted the reaction more significantly than the higher reactant concentrations did. Whereas the substantial increase of uap from 6H to 12H in both series may arise from the increase in crystallinity more than in crystal size. As such, it would be the higher m0 value at 12H, rather than uap, that accounts for the slight rebound in m, thus terminating its monotonous downward trend.
The pseudo-equilibrium constant Kd,m is the other parameter derived from a ln[Sp] vs. ln(IP)0 plot in Fig. 2. Since the net reaction of the crystal ripening process38,50 led to the formation of HAP crystals, we use 1/Kd,m (column 1 from right, Table 2) to calculate the free energy difference (eqn (44)) for Series CPn. In the upper part of Fig. 3, Ind-2 data are depicted for comparison. The calculated value of the free energy difference between ACP (GA) and the reactants is ΔGA = GA − GInd-2 = −29.9 kJ mol−1. The negative sign indicates that the relative potential energy of the reactants was above that of ACP, producing a positive thermodynamic driving force for the spontaneous formation of ACP.
where
R = 8.31 J K
−1 mol
−1 and
T = 298 K.
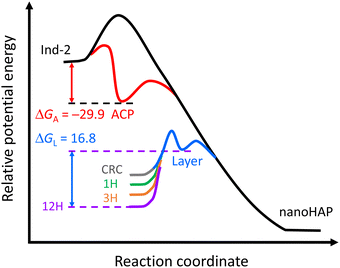 |
| Fig. 3 The potential energy profile for the reaction of calcium phosphate precipitation in Series CPn. The values of ΔGA and ΔGL (unit: kJ mol−1) correspond to the formation of amorphous calcium phosphate and the reacting entities at the crystal growth front in the interfacial layer of a hydroxyapatite nanoparticle, respectively. Other ΔGL values are 6.80 (CRC), 13.0 (1H), 14.3 (3H), and 14.9 (6H, not depicted) kJ mol−1. The ordinates of these states are not presented to scale. | |
Shown in the lower part of Fig. 3 are the relative potential energies concerning the crystal ripening process from CRC onwards, where GL indicates the reacting entities at the crystal growth front in the interfacial layer. Different from the circumstances of ACP, the positive values of ΔGL (ΔGL = GL − Gi, i = CRC, 1H, 3H, and 12H) indicate that the relative potential energies of the reactants were lower than that of the product (GL), and the gap widened over time. The reduced reactant concentrations account for the decrease in the thermodynamic driving force (ΔG = Gi − GnanoHAP), and the concomitant increase in the activation energy (>ΔGL) explains the observed slowdown of the crystal ripening process.
3.6 Special forms of pseudo-equilibrium equations
Eqn (34) and (35) are the two types of pseudo-equilibrium equations for the precipitation reaction with a whole multi-unit particle (eqn (8)). Using the two equations, we calculate the Kd,u values for Series CPn and Series pH, respectively. As shown in Fig. 4, the [Su]1/u value approach 1 from CRC onwards, making the Kd,u value close to (IP)m0. Accordingly, both equations become the common form shown in eqn (45). |
Kd,u = (IP)m0 (u → ∞)
| (45) |
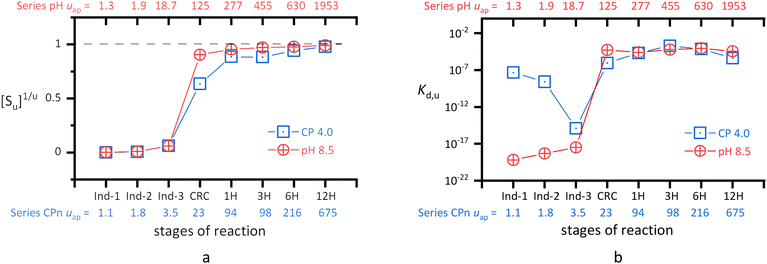 |
| Fig. 4 Trends in the precipitate concentration ([Su]1/u, a) and the pseudo-equilibrium constant (Kd,u in log10 scale, b). The apparent numbers of structural units (uap) in a particle are indicated along the lower (Series CPn) and upper (Series pH) abscissas. The [Su] values were calculated by eqn (36). The Kd,u values were calculated by eqn (34) for the CP4 system in Series CPn and by eqn (35) for the pH 8.5 system in Series pH. | |
However, the stoichiometry and magnitude of (IP)m0 differ from those of the solubility product of HAP (Ca10(OH)2(PO4)6, Ksp = 9.2 × 10−118 at 25 °C55), respectively. The discrepancy arises from the difference between certain lattice ions (PO43− and OH−) and their respective solution forms (HPO42− and H2O). The PO43− and OH− ions are generated at the crystal growth front (e.g., steps, kinks) in the interfacial layer. But the reacting entities did not obtain PO43− and OH− directly from the near-neutral solution; rather, they produced the PO43− and OH− anions in situ through the deprotonation of Ca-bound HPO42−
56 and H2O,37,57 respectively. Based on the Brønsted–Lowry concept of conjugate acids and bases, CaHPO4 (pKa = 8.558) is a better proton donor than a free HPO42− ion (pKa3 = 12.3659), and the latter is a better proton acceptor than an H2O molecule. Moreover, if a free HPO42− ion forms a hydrogen bond with the leaving proton in a Ca-bound HPO42− ion, it would further facilitate the proton transfer reaction (eqn (46)). Similar ways of proton transfer through the interfacial layer have been reported.25,60
|
HPO42−(Ca-bound) + HPO42−(H-bonding with leaving H) = PO43− (in ACP or HAP) + H2PO4− (free)
| (46) |
Although the OH− ion is absent in the formulas in Table 2, HAP nanoparticles did exist in the system from CRC onwards.38,61 The inconsistency is caused by the average nature of the r (= Ca/P) values. The presence of the OH− ion in the precipitate formula requires r > 1.5. But the HPO42− ions in the interfacial layer19,22,29,30 resulted in a lower r value than that in the crystalline core, as indicated in eqn (42) and (43). Given the large specific surface area of the nanoparticles, the influence of the interfacial layer was significant on the r values. Previously, a Ca/P molar ratio of 1.30–1.48 has been reported for HAP nanoparticles.24
And yet, the interfacial layer might be exactly where the OH− ion was generated. Since the acidity of H2O is much lower than that of HPO42−, its deprotonation demands a stronger proton acceptor. A feasible route of reaction is presented in eqn (47) and Scheme 2, where a Ca-bound PO43− anion acts as the proton acceptor: it can form a hydrogen bond with the leaving proton and, after capturing the proton, leaves the cluster as a free HPO42− ion.37,62 A recent study by 31P{1H} HETCOR NMR has revealed the close contact between the PO43− anion and the structure-bound H2O,63 indicating the possible involvement of PO43− in the deprotonation of H2O. Notably, the presence of HPO42− near a Ca-bound H2O molecule would reduce the deprotonation efficiency of the latter, owing to its higher acidity than the Ca-bound H2O molecule. The inverse relationship between the contents of OH− and HPO42− has been observed at the surface of hydroxy-carbonate apatite.24,64 Furthermore, to prevent the OH− anion from forming hydrogen bonds with H2O, several Ca2+ ions are required to compose the Ca2-triangle around the OH− anion.65–67 These Ca2+ ions, all in the form of calcium phosphate clusters, should become part of a crystal along with the OH− anion. This transformation, as the last step of crystal growth, must involve an event of bond-type transition (BTT), i.e. the weak covalent Ca–O (from OH− and PO43−) bonds in the aqueous medium become the ionic bonds in a crystal, followed by the adjustment of coordination numbers and the relative positions among these ions. Besides, a Ca1 ion is located between two PO43− triangles rotated 60° about the c-axis in HAP, and it is less likely that the multiple Ca1–O (from PO43−) ionic bonds are formed one by one. Therefore, the BTT event may occur synergistically in several calcium phosphate clusters, generating a BTT unit with a dimension comparable to the observed growth steps (0.5–3 nm) in apatite crystallites.68 At this stage, the HPO42−-containing “Posner-like clusters” (that are generated in molecular dynamics simulations)69 and Posner's cluster (that is proposed to be “an ultimate growth unit of HAP”70) could be developed successively and involved in BTT at the crystal growth front. An analogue to BTT, the bcc-to-fcc transition in crystal nucleation, has been observed in computer simulations.71
|
H2O (Ca-bound) + PO43− (Ca-bound) = OH− (embedding in a Ca-triangle) + HPO42− (free)
| (47) |
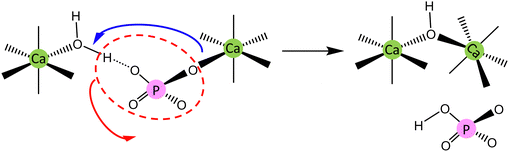 |
| Scheme 2 Generation of OH−via the proton transfer from a Ca-bound H2O molecule to a Ca-bound PO43− anion (the ion charges are omitted for clarity). | |
It can be anticipated that the amount of the reacting entities will be negligible when the reaction reaches equilibrium. If (IP)m0 has the same stoichiometry and magnitude as the solubility product of HAP then, eqn (45) becomes eqn (48) as the final special form of the two types of pseudo-equilibrium equations.
|
Ksp = solubility product
| (48) |
4. Conclusions
We have presented a pseudo-equilibrium approach to the solution chemistry of the precipitation reaction, which enables us to derive two types of pseudo-equilibrium equations. These equations have revealed some aspects of the non-equilibrium thermodynamics of reaction systems, indicating the solution chemistry route to the equilibrium state. Since ACP and HAP nanoparticles represent the materials in the non-equilibrium states far from and near the equilibrium state, respectively, the following conclusions drawn from them could apply to a broad range of precipitation reactions in laboratories, nature, and biology.
(1) There exist multiple structural units (u) in a precipitate particle.
(2) The ionic product responds to the surface proportion per structural unit (m), and the latter is related to the whole particle through a particle-surface equation.
(3) For a crystalline nanoparticle in a pseudo-equilibrium state, the “surface proportion” includes the contributions from both the surface of the bulk crystal and the materials in the interfacial layer, and the composition of the latter is dependent on the extent of reaction.
(4) The two types of pseudo-equilibrium equations approach the common specific form of “Kd,u = ionic product” as the number of structural units gets large enough.
Abbreviations
ACP | Amorphous calcium phosphate |
HAP | Hydroxyapatite |
ICP-OES | Inductively coupled plasma optical emission spectrometry |
CRC | Completion of the rapid change period |
IP | Ionic product |
LCa | Calcium concentration in the supernatant |
LPi | Phosphate concentration in the supernatant |
cCa | Complexed Ca2+ in the supernatant |
cPi | Ca-bound phosphate in the supernatant |
HETCOR NMR | Heteronuclear correlation nuclear magnetic resonance |
BTT | Bond-type transition |
Conflicts of interest
There are no conflicts to declare.
Acknowledgements
This work was jointly funded by the Natural Science Foundation of China and the Chinese Academy of Sciences (grant number U1632105). The author would like to thank Prof. B.-D. Gou for his help in the determination of Ca and P contents in the samples.
Notes and references
- J. B. Lian, G. S. Stein, E. Canalis, P. G. Robey and A. L. Boskey, in Primer on the Metabolic Bone Diseases and Disorders of Mineral Metabolism, ed. M. J. Favus, Lippincott Williams & Wilkins, Philadelphia, PA, USA, 4th edn, 1999, ch. 3, pp. 14–29 Search PubMed.
- E. Beniash, Wiley Interdiscip. Rev.: Nanomed. Nanobiotechnol., 2011, 3, 47–69 CrossRef CAS PubMed.
- E. D. Eanes, I. H. Gillessen and A. S. Posner, Nature, 1965, 208, 365–367 CrossRef CAS PubMed.
- R. A. Harper and A. S. Posner, Proc. Soc. Exp. Biol. Med., 1966, 122, 137–142 CrossRef CAS PubMed.
- J. D. Termine and A. S. Posner, Calcif. Tissue Res., 1967, 1, 8–23 CrossRef CAS PubMed.
- J. D. Termine and A. S. Posner, Science, 1966, 153, 1523–1525 CrossRef CAS PubMed.
- A. S. Posner, Physiol. Rev., 1969, 49, 760–792 CrossRef CAS PubMed.
- M. Grynpas, L. Bonar and M. Glimcher, Calcif. Tissue Int., 1984, 36, 291–301 CrossRef CAS PubMed.
- M. J. Glimcher, L. C. Bonar, M. D. Grynpas, W. J. Landis and A. H. Roufosse, J. Cryst. Growth, 1981, 53, 100–119 CrossRef CAS.
- N. J. Crane, V. Popescu, M. D. Morris, P. Steenhuis and M. A. Ignelzi, Bone, 2006, 39, 434–442 CrossRef CAS PubMed.
- S. Weiner, Bone, 2006, 39, 431–433 CrossRef CAS PubMed.
- M. D. Grynpas and S. Omelon, Bone, 2007, 41, 162–164 CrossRef CAS PubMed.
- J. Mahamid, A. Sharir, L. Addadi and S. Weiner, Proc. Natl. Acad. Sci. U. S. A., 2008, 105, 12748–12753 CrossRef CAS PubMed.
- C. Rey, C. Combes, C. Drouet and M. J. Glimcher, Osteoporosis Int., 2009, 20, 1013–1021 CrossRef CAS PubMed.
- J. Mahamid, B. Aichmayer, E. Shimoni, R. Ziblat, C. Li, S. Siegel, O. Paris, P. Fratzl, S. Weiner and L. Addadi, Proc. Natl. Acad. Sci. U. S. A., 2010, 107, 6316–6321 CrossRef CAS PubMed.
- J. Mahamid, A. Sharir, D. Gur, E. Zelzer, L. Addadi and S. Weiner, J. Struc. Biol., 2011, 174, 527–535 CrossRef CAS PubMed.
- A. Akiva, M. Kerschnitzki, I. Pinkas, W. Wagermaier, K. Yaniv, P. Fratzl, L. Addadi and S. Weiner, J. Am. Chem. Soc., 2016, 138, 14481–14487 CrossRef CAS PubMed.
- L. Bertinetti, A. Tampieri, E. Landi, C. Ducati, P. A. Midgley, S. Coluccia and G. Martra, J. Phys. Chem. C, 2007, 111, 4027–4035 CrossRef CAS.
- C. Jäger, T. Welzel, W. Meyer-Zaika and M. Epple, Magn. Reson. Chem., 2006, 44, 573–580 CrossRef PubMed.
- V. R. Deitz, H. M. Rootare and F. G. Carpenter, J. Colloid Sci., 1964, 19, 87–101 CrossRef CAS.
- Y. Sakhno, L. Bertinetti, M. Iafisco, A. Tampieri, N. Roveri and G. Martra, J. Phys. Chem. C, 2010, 114, 16640–16648 CrossRef CAS.
- V. Uskoković, Phys. Chem. Chem. Phys., 2020, 22, 5531–5547 RSC.
- N. T. K. Thanh, N. Maclean and S. Mahiddine, Chem. Rev., 2014, 114, 7610–7630 CrossRef CAS PubMed.
- N. Vandecandelaere, C. Rey and C. Drouet, J. Mater. Sci.: Mater. Med., 2012, 23, 2593–2606 CrossRef CAS PubMed.
- C. Drouet, M. Aufray, S. Rollin-Martinet, N. Vandecandelaère, D. Grossin, F. Rossignol, E. Champion, A. Navrotsky and C. Rey, Am. Mineral., 2018, 103, 550–564 CrossRef.
- J. B. Best, Biochim. Biophys. Acta, 1959, 32, 194–202 CrossRef CAS PubMed.
- M. Edén, Materialia, 2021, 17, 101107 CrossRef.
- G. M. L. Dalmônico, E. O. López, M. M. Longuinho, N. R. Checca, M. Farina, O. Ersen, A. M. Rossi and A. L. Rossi, Mater. Chem. Phys., 2019, 237, 121862 CrossRef.
- J. D. Termine and E. D. Eanes, Calcif. Tissue Res., 1972, 10, 171–197 CrossRef CAS PubMed.
- H. Chappell, M. Duer, N. Groom, C. Pickard and P. Bristowe, Phys. Chem. Chem. Phys., 2008, 10, 600–606 RSC.
- O. Söhnel and F. Grases, Urol. Res., 2011, 39, 429–436 CrossRef PubMed.
- J. L. Meyer and E. D. Eanes, Calcif. Tissue Res., 1978, 25, 59–68 CrossRef CAS PubMed.
- C.-G. Wang, J.-W. Liao, B.-D. Gou, J. Huang, R.-K. Tang, J.-H. Tao, T.-L. Zhang and K. Wang, Cryst. Growth Des., 2009, 9, 2620–2626 CrossRef CAS.
- A. S. Posner and F. Betts, Acc. Chem. Res., 1975, 8, 273–281 CrossRef CAS.
- V. Čadež, I. Erceg, A. Selmani, D. Domazet Jurašin, S. Šegota, D. Lyons, D. Kralj and M. Sikirić, Crystals, 2018, 8, 254 CrossRef.
- T. P. Feenstra and P. L. De Bruyn, J. Colloid Interface Sci., 1981, 84, 66–72 CrossRef CAS.
- L.-W. Du, S. Bian, B.-D. Gou, Y. Jiang, J. Huang, Y.-X. Gao, Y.-D. Zhao, W. Wen, T.-L. Zhang and K. Wang, Cryst. Growth Des., 2013, 13, 3103–3109 CrossRef CAS.
- H.-X. Fan, B.-D. Gou, Y.-X. Gao, G. Wu and T.-L. Zhang, Phys. Chem. Chem. Phys., 2019, 21, 22057–22066 RSC.
- N. A. Garcia, R. I. Malini, C. L. Freeman, R. Demichelis, P. Raiteri, N. A. J. M. Sommerdijk, J. H. Harding and J. D. Gale, Cryst. Growth Des., 2019, 19, 6422–6430 CrossRef CAS PubMed.
- B.-Q. Lu, N. A. Garcia, D. M. Chevrier, P. Zhang, P. Raiteri, J. D. Gale and D. Gebauer, Cryst. Growth Des., 2019, 19, 3030–3038 CrossRef CAS.
- A. J. Hoeher, S. T. Mergelsberg, O. J. Borkiewicz and F. M. Michel, Cryst. Growth Des., 2021, 21, 3736–3745 CrossRef CAS.
- N. Kanzaki, G. Treboux, K. Onuma, S. Tsutsumi and A. Ito, Biomaterials, 2001, 22, 2921–2929 CrossRef CAS PubMed.
- B. Xie, T. J. Halter, B. M. Borah and G. H. Nancollas, Cryst. Growth Des., 2014, 14, 1659–1665 CrossRef CAS PubMed.
- W. J. E. M. Habraken, J. Tao, L. J. Brylka, H. Friedrich, L. Bertinetti, A. S. Schenk, A. Verch, V. Dmitrovic, P. H. H. Bomans, P. M. Frederik, J. Laven, P. van der Schoot, B. Aichmayer, G. de With, J. J. DeYoreo and N. A. J. M. Sommerdijk, Nat. Commun., 2013, 4, 1507 CrossRef PubMed.
- Q. Zhang, Y. Jiang, B.-D. Gou, J. Huang, Y.-X. Gao, J.-T. Zhao, L. Zheng, Y.-D. Zhao, T.-L. Zhang and K. Wang, Cryst. Growth Des., 2015, 15, 2204–2210 CrossRef CAS.
- M. R. Christoffersen, J. Christoffersen and W. Kibalczyc, J. Cryst. Growth, 1990, 106, 349–354 CrossRef CAS.
- C. Holt, E. S. Sørensen and R. A. Clegg, FEBS J., 2009, 276, 2308–2323 CrossRef CAS PubMed.
- E. D. Eanes, J. D. Termine and M. U. Nylen, Calcif. Tissue Res., 1973, 12, 143–158 CrossRef CAS PubMed.
- Q. Zhang, Y. Liu, B.-D. Gou, L. Zheng, Y.-X. Gao and T.-L. Zhang, RSC Adv., 2016, 6, 102710–102723 RSC.
- J. Termine, R. Peckauskas and A. Posner, Arch. Biochem. Biophys., 1970, 140, 318–325 CrossRef CAS PubMed.
- J. R. Dorvee and A. Veis, J. Struct. Biol., 2013, 183, 278–303 CrossRef CAS PubMed.
- I. Greenwald, J. Biol. Chem., 1942, 143, 703–710 CrossRef CAS.
- A. Veis and J. Dorvee, Calcif. Tissue Int., 2013, 93, 307–315 CrossRef CAS PubMed.
- J. E. Roberts, M. Heughebaert, J. C. Heughebaert, L. C. Bonar, M. J. Glimcher and R. G. Griffin, Calcif. Tissue Int., 1991, 49, 378–382 CrossRef CAS PubMed.
- H. McDowell, T. M. Gregory and W. E. Brown, J. Res. Natl. Bur. Stand., Sect. A, 1977, 81A, 273–281 CrossRef CAS.
- D. Zahn, Z. Anorg. Allg. Chem., 2004, 630, 1507–1511 CrossRef.
- Z. Zyman, D. Rokhmistrov and V. Glushko, J. Cryst. Growth, 2012, 353, 5–11 CrossRef CAS.
- I. Greenwald, J. Phys. Chem., 1963, 67, 2853–2854 CrossRef CAS.
- D. R. Lide, CRC Handbook of Chemistry and Physics, CRC Press, 2008 Search PubMed.
- C. Rey, C. Combes, C. Drouet, S. Cazalbou, D. Grossin, F. Brouillet and S. Sarda, Prog. Cryst. Growth Charact. Mater., 2014, 60, 63–73 CrossRef CAS.
- J. D. Termine and A. S. Posner, Arch. Biochem. Biophys., 1970, 140, 307–317 CrossRef CAS PubMed.
- V. Uskoković, Cryst. Growth Des., 2019, 19, 4340–4357 CrossRef.
- O. F. Yasar, W.-C. Liao, B. Stevensson and M. Edén, J. Phys. Chem. C, 2021, 125, 4675–4693 CrossRef CAS.
- J. L. Meyer, Calcif. Tissue Int., 1979, 27, 153–160 CrossRef CAS PubMed.
- D. Zahn and O. Hochrein, Phys. Chem. Chem. Phys., 2003, 5, 4004–4007 RSC.
- M. I. Kay, R. A. Young and A. S. Posner, Nature, 1964, 204, 1050–1052 CrossRef CAS PubMed.
- K. Matsunaga, J. Am. Ceram. Soc., 2010, 93, 1–14 CrossRef CAS.
- A. Lotsari, A. K. Rajasekharan, M. Halvarsson and M. Andersson, Nat. Commun., 2018, 9, 4170 CrossRef PubMed.
- G. Mancardi, C. E. Hernandez Tamargo, D. Di Tommaso and N. H. de Leeuw, J. Mater. Chem. B, 2017, 5, 7274–7284 RSC.
- K. Onuma and A. Ito, Chem. Mater., 1998, 10, 3346–3351 CrossRef CAS.
- P. R. ten Wolde and D. Frenkel, Phys. Chem. Chem. Phys., 1999, 1, 2191–2196 RSC.
Footnotes |
† The paper is dedicated to Professor K. Wang who led the author to this field. |
‡ Electronic supplementary information (ESI) available. See DOI: https://doi.org/10.1039/d3cp03700b |
|
This journal is © the Owner Societies 2024 |
Click here to see how this site uses Cookies. View our privacy policy here.