Integrated fabrication of CMC@UiO-66–NH2@PEI composite adsorbents for efficient batch and dynamic phosphate capture†
Received
16th September 2023
, Accepted 6th November 2023
First published on 7th November 2023
Abstract
Adsorption technology has been regarded as an efficient method for removing low-concentration phosphate ions from water; however, the designed fabrication of expectable sorbents by integrating biomass components and MOFs remains a challenge. Herein, carboxymethylcellulose (CMC) and UiO-66–NH2 were mixed evenly and then dropped into a lanthanum chloride solution to form beads. Subsequently, the formed pellets underwent in situ polymerization using polyethyleneimine (PEI) to modify their surface. This surface modification with PEI aimed to enhance the mechanical stability of the pellets and improve their ability to remove phosphorus. This is an interesting approach to achieving enhanced performance in terms of both mechanical stability and phosphorus removal. We conducted an investigation into the impact of varying MOF contents and PEI concentrations on the adsorption performance. The optimal ratio adsorbent 0.2CUI has a maximum adsorption capacity of 293.27 mg P per g for phosphate. After investigating the impact of pH on the adsorption performance, we have discovered that 0.2CUI is highly effective in adsorbing phosphate across a broad pH spectrum. Adsorption investigations have indicated that the adsorption of phosphate follows the pseudo-second-order kinetic model and the Freundlich isotherm model. Furthermore, the dynamic adsorption performance tests revealed a significant correlation coefficient for the Thomas model. At the same time, cyclic experiments involving adsorption and desorption were conducted to assess the reusability of the prepared hydrogel. The advancement of phosphate adsorbents is approached from a new perspective in this study.
Water impact
To efficiently remove excessive phosphorus from water and mitigate its impact on human health and the environment, we developed 0.2CUI spheres by combining CMC with a MOF through ion cross-linking and modifying the surface with PEI. These spheres have a high adsorption capacity of 293.27 mg g−1 for phosphates, surpassing other adsorbents like cellulose. Moreover, the spheres address the issue of poor MOF recovery and offer a novel approach for MOF and biomass integration.
|
1. Introduction
Phosphorus is a crucial nutrient in ecosystems and a vital macronutrient for microorganisms in water systems. It serves as a limited and non-renewable resource, positively influencing the population of planktonic organisms. However, excessive phosphate content in water leads to eutrophication, causing uncontrolled algae growth, habitat deterioration for fish, and decreased dissolved oxygen levels, and ultimately harming biodiversity.1–3 Eutrophication is becoming one of the most prevalent global water pollution problems, with increased concentrations of nutrients such as nitrogen and phosphorus being the leading cause of eutrophication.4,5 In urban sewage treatment, it is of great significance to reduce phosphorus discharge. At present, there are many methods to remove phosphate in water, such as electrochemical methods,6 membrane separation,7 biological treatment,8 chemical precipitation,9,10 adsorption,11,12etc. Compared with other methods, the adsorption method has emerged as a highly promising and extensively employed technique owing to its adaptable design, straightforward operation, and cost-effectiveness.13
In recent years, the application of metal–organic frameworks (MOFs) in adsorption has sparked notable interest due to their versatility, porosity, adaptability, exceptional stability, and significant specific surface area.14 In general, following the principles of the hard and soft acid–base theory, the metal centres within MOFs have a tendency to selectively interact with phosphate, which acts as a typical hard Lewis base.15 UiO-66 has gained considerable recognition among the diverse spectrum of MOFs, primarily because of its remarkable thermal and chemical stability. It exhibits an exceptional ability to withstand boiling water for extended periods without undergoing any structural deterioration.16 UiO-66 is a metal organic framework comprising of zirconium as the metal ligand and terephthalic acid as the ligand. It exhibits stability in aqueous solutions and acidic environments, and possesses remarkable thermal stability. Furthermore, zirconium metal has a strong affinity for adsorbing phosphate.17 To enhance the adsorption efficiency of phosphate, the ligand was modified to 2-NH2-BDC, and subsequently, UiO-66–NH2 was synthesized to yield improved adsorption capabilities for subsequent experimental investigations. Zhang et al. successfully synthesized UiO-66–NH2 using a greener and more environmentally friendly approach, which demonstrates commendable adsorption capabilities for phosphate.18 The presence of protonated-NH2 within its structure facilitates electrostatic attraction towards phosphate, thereby enhancing its adsorption properties. Consequently, for this study, the composite was formulated using UIO-66–NH2 as the principal material. Nevertheless, the regeneration of MOFs poses an inevitable drawback, as the framework is susceptible to collapse under acidic or basic desorption conditions.19,20
Sodium carboxymethylcellulose (CMC) is a natural substrate that possesses a distinctive chemical structure, enabling it to form a solid film either through physical means such as hydrogen bonding or chemically by utilizing certain chemical crosslinking agents. At the same time, CMC exhibits an amorphous structure, making it a suitable material for diverse hydrogel applications. However, its mechanical strength is relatively weak and needs to be enhanced in order to improve its effectiveness in various applications.21 Simultaneously, CMC has the ability to undergo cross-linking with metal ions, resulting in the formation of a relatively stable hydrogel. Li et al. simply cross-linked CMC with aluminium ions to prepare adsorption beads with apparent adsorption capacity for heavy metal ions.22 At the same time, among many metals, lanthanum (La), as a rare earth metal, is easy to form stable La3+, and it has a specific recognition ability for phosphate. It is, therefore, often used to remove phosphate from water in adsorption experiments.1 Yang et al. successfully developed a novel remover by loading CGCS onto a magnesium–lanthanum bimetallic system. This innovative solution demonstrates exceptional efficiency in swiftly eliminating phosphate from water, with a maximum adsorption capacity of 39.22 mg g−1.23 Hence, CMC was cross-linked with lanthanum ions to produce beads specifically designed for phosphate adsorption.
Polyethyleneimine (PEI) is a polyvalent cationic surfactant which is rich in amino groups.24 As a result, we employed PEI as a modifier to enhance the previously formed spheres, ensuring that the composite material possesses ample adsorption active sites and improved mechanical stability. The modification of the microspheres with PEI resulted in the formation of wrinkles on their surface, leading to increased surface roughness. This enhanced surface roughness further strengthened the adsorption phenomenon.25
Yang et al. combined MIL-101(Cr) with CMC and used PVA for cross-linking in order to improve the adsorption performance for metal ions. They also provided a new way to combine MOF materials and CMC.26 On this basis, we modified CMC with PEI after combining it with a MOF in order to improve the material stability and adsorption performance. Consequently, this paper presents the preparation of an adsorbent with exceptional selectivity for phosphorus adsorption. Initially, UiO-66–NH2 was synthesized using hydrothermal methods. Subsequently, CMC and UiO-66–NH2 were thoroughly blended and added to a solution of lanthanum chloride, forming CMC/UiO-66–NH2/La (CLU) spheres. Finally, the material was modified using polyethyleneimine, ultimately yielding the CMC/UiO-66–NH2/La/PEI (0.2CUI) adsorbent. The preparation method is straightforward and user-friendly, and the resulting adsorbent beads offer easier recovery compared to powdered forms. This simplifies subsequent use processes and reduces complexity. It effectively tackles the challenge of problematic reutilization for MOF materials. After the combination of CMC and the MOF, the pore volume will relatively decrease, which affects the adsorption performance. The modification with PEI not only improves the specific surface area but also enhances stability, and the crosslinking mechanism better reflects the adsorption effect of 1 + 1 > 2.27 This study comprehensively examined the influence of reaction temperature, contact time, initial concentration, solution pH, and coexisting ions on phosphorus adsorption. In addition, the commonly used isothermal and kinetic models were used to fit the experimental data. To gain a more profound insight into the adsorption mechanism of the adsorbent, we conducted comprehensive characterization of the adsorbent beads, both before and after adsorption, using advanced techniques such as SEM, XRD, XPS, and FT-IR. The resultant characterization findings were then analysed alongside the adsorption mechanism to obtain a thorough understanding. In addition, dynamic column adsorption experiments were performed to evaluate the potential application of this solution in industry.
2. Experiment process
2.1 Materials
The materials applied to this work are presented in Text S1.†
2.2 Preparation of CUI
UiO-66–NH2 was synthesized using a hydrothermal method, following the subsequent procedure: dissolve 0.3065 g of 2-NH2–BDC and 0.4024 g of ZrCl4 in two beakers containing 20 ml of DMF, which were designated as solutions A and B, respectively. After reacting for 25 minutes, add 2.5 ml of CH3COOH to solution A, mix it evenly with solution B, pour it into 100 ml of polytetrafluoroethylene lining, and react the mixture hydrothermally at 120 °C for 24 hours. Following centrifugation and washing, dry it in an oven at 80 °C for 12 hours to obtain the final product UiO-66–NH2.
Dissolve 0.9 g of CMC in 30 ml of water, stir well, add 0.1 g, 0.15 g, 0.2 g, 0.25 g, and 0.3 g of UiO-66–NH2, respectively, stir continuously to mix the two thoroughly, and then drop 5% (w/v) mixture to LaCl3·6H2O solution, solidify for 6 hours, and then react in PEI (1.25%, 1.5%, 1.75%) solution for 6 hours to obtain the CMC/UiO-66–NH2/La/PEI (CUI) adsorbent for the final the adsorption test. Follow the steps above to prepare the corresponding CL (without UiO-66–NH2, drop the CMC solution directly into the LaCl3·6H2O solution) and CLU (the steps are the same but without PEI modification).
2.3 Characterization methods
The specific characterization methods and corresponding instruments are displayed in Text S2.†
2.4 Batch static adsorption study
The adsorption capability of 0.2CUI for phosphate was evaluated through a series of adsorption experiments. In each experiment, 20 mg of 0.2CUI was introduced into an Erlenmeyer flask containing a 20 mL phosphate solution. The reaction was allowed to fully proceed for 24 hours until adsorption equilibrium was achieved. The formula used for calculating the adsorption capacity is as follows: | 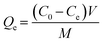 | (1.1) |
In the above formula, C0 (mg L−1) represents the concentration at the beginning of adsorption, and Ce (mg L−1) represents the phosphate concentration in solution at the equilibrium state of adsorption. M (mg) represents the mass of the adsorbent used and V (mL) represents the volume of phosphate solution in the experiment.
Different quantities of 0.2CUI (ranging from 0.25 to 1.25 g L−1) were added to a phosphate solution of fixed concentration and allowed to fully react to determine the optimal mass of the adsorbent. A series of hydrochloric acid and sodium hydroxide solutions with varying concentrations were prepared to adjust the initial pH (ranging from 3 to 11) of the phosphate solution, and the impact of pH on the adsorption performance was investigated.
By changing the initial concentration of phosphate solution, the adsorption isotherm of phosphate on 0.2CUI was studied. The concentration of the solution measured is set at 100 to 1000 mg L−1. Different isotherm models can be expressed as the following formulas:
Langmuir isotherm models:
|  | (1.2) |
Freundlich isotherm models:
Among them,
Qm (mg g
−1) represents the maximum adsorption capacity of 0.2CUI, and
Qe (mg g
−1) represents the equilibrium adsorption capacity of 0.2CUI.
Ce (mg L
−1) denotes the concentration of phosphate in the solution following the reaction.
KL represents a constant of the Langmuir model. Both
KF and
n are empirical constants of the Freundlich model.
The adsorption kinetics of phosphate on 0.2CUI was studied by changing the reaction time, and the interaction between phosphate and the adsorbent was analysed with different kinetic models. The formulas for its different models are as follows:
Pseudo-first-order model:
| ln(Qe − Qt) = ln Qe − K1t | (1.4) |
Pseudo-second-order model:
| 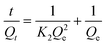 | (1.5) |
Intraparticle diffusion model:
where
Qe (mg g
−1) and
Qt (mg g
−1) represent the adsorption capacity at equilibrium and time
t (min), respectively.
K1 and
K2 are rate constants.
Ki and
θ are the diffusion coefficient and the intercept of the in-diffusion model, respectively.
Keeping other conditions constant, adsorption experiments were conducted at three temperatures, namely 298.15 K, 308.15 K, and 318.15 K, to investigate the impact of temperature on the phosphate adsorption process. Thermodynamic parameters including heat change, free energy change and entropy change can be obtained by the following formula. The calculation is derived from the following equation:
|  | (1.7) |
| 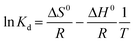 | (1.8) |
| ΔG0 = −RT ln Kd | (1.9) |
Among them,
T (K) refers to the Kelvin temperature, and
R (8.314 J K
−1 mol) represents the ideal gas constant.
Qe (mg L
−1) represents the adsorption capacity at adsorption equilibrium.
Kd is the thermodynamic equilibrium constant.
Ce (mg L
−1) refers to the residual concentration of phosphate in the solution when the adsorption process reaches saturation.
ΔG0 (KJ mol−1) indicates the change in free energy and can be calculated using eqn (1.9). ΔH0 and ΔS0 can be obtained from the intercept and slope of the linear relationship between ln
Kd and 1/T.
At the same time, the competitive adsorption effect of coexisting anions with different proportions of Cl−, NO3−, SO42− and phosphate was studied. Sodium hydroxide, hydrochloric acid, and sodium chloride were used as desorbents to elute phosphate from 0.2CUI for recycling.
2.5 Column adsorption study of phosphate removal by 0.2CUI
The practical effectiveness of 0.2CUI pellets in treating wastewater containing phosphate was assessed through bed column adsorption tests. We chose 0.277 g dry 0.2CUI pellets for adsorption studies on fixed bed columns. The concentration of phosphate solution used was 50 mg L−1 and the flow rates were set at 1 mL min−1 and 3 mL min−1, respectively. The effluent solution is collected in a graduated square container and the breakthrough point of the column is defined as Ct/C0 = 0.9 (where C0 and Ct represent the phosphate concentrations at the inlet and outlet ends in mg P per L).
The formulation of the model used is presented in Text S3.†
3. Results and discussion
3.1 Performance comparison of different adsorbents
Various quantities of UiO-66–NH2 were added during the preparation of the adsorbent, along with varying concentrations of the PEI solution used for modification. Consequently, a range of adsorbents were prepared for the purpose of phosphate adsorption. In order to assess the effectiveness, experiments were carried out under consistent conditions, and the most suitable adsorbent was thus identified. The findings from the experiments are depicted in Fig. S1(a).† It was observed that the adsorption performance of CLU was optimal when modified with a 1.75% PEI solution. During the adsorbent preparation process, varying quantities of UiO-66–NH2 were added, and the resulting pellets were modified using a 1.75% PEI solution to obtain five different adsorbents. Subsequent adsorption experiments were conducted for each of these adsorbents, as illustrated in Fig. S1(b).† Based on these results, the adsorbent 0.2CUI was chosen for further investigations.
3.2 Characterization of 0.2CUI
3.2.1 Morphology and composition analysis.
The surface morphology of the prepared 0.2CUI beads was examined using SEM and EDS, as depicted in Fig. 1. The micrographs revealed well-formed spherical shapes of different composite microbeads, along with cross-sectional and microstructure images. The surface of unprocessed CL microbeads appeared to be relatively dense and smooth, exhibiting a tighter network structure (Fig. 1(e)). However, with the inclusion of UiO-66–NH2, a relatively looser network structure was observed in the microstructure. This porous surface structure could potentially enhance the adsorption of phosphate, as it provides more collision opportunities between phosphate and adsorption sites, while also offering more exposed metal centers (Fig. 1f). Following the modification with PEI, it is evident that the adsorbent exhibits a distinct interconnected and distinctive porous network structure (Fig. 1g), and it is enriched with the nitrogen (N) element, which plays a vital role in enhancing the performance of phosphorus uptake. Following the adsorption of phosphorus, the P element was evenly distributed across the surface of the adsorbent, as observed in Fig. 1h. The main structure of the hydrogel adsorbent remained intact, demonstrating its stability. The successful loading of phosphate also resulted in a smoother and more compact hydrogel surface, as depicted in Fig. 1d. The EDS spectrum of 0.2CUI after phosphate adsorption (Fig. 2) confirmed the successful capture of the P element by 0.2CUI. Furthermore, it was demonstrated that the phosphorus element was evenly distributed throughout the adsorbent, thereby providing additional confirmation of the efficacy of the phosphate adsorption process.
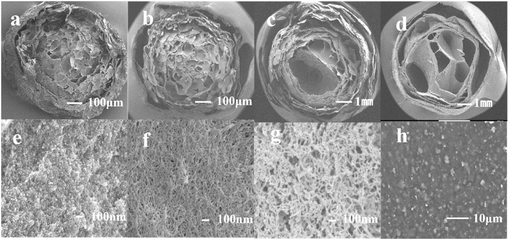 |
| Fig. 1 SEM images of CL (a and e), CLU (b and f), 0.2CUI (c and g), and 0.2CUI-P (d and h). | |
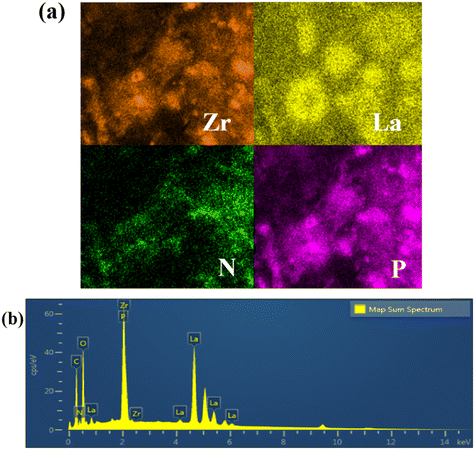 |
| Fig. 2 (a) EDS mapping images of 0.2CUI after phosphate adsorption; (b) EDS spectrum of 0.2CUI after phosphate adsorption. | |
3.2.2 Molecular analysis and characterisation.
In order to investigate the successful synthesis of 0.2CUI composites and their effective adsorption of phosphate, we characterised the functional groups of the materials before and after adsorption by FT-IR to observe the changes. As shown in Fig. 3, the IR spectra of UiO-66–NH2 (a), CMC (b), CL (c), CLU (d), 0.2CUI (e) and 0.2CUI-P (f) composites are shown. In Fig. 3a, the peak observed at 3447 cm−1 is regarded as the asymmetric stretching vibration of the amino group, the peak at 1574 cm−1 is attributed to the symmetric stretching vibration of the C
O bond on the aromatic ring and the peak at 1259 cm−1 is attributed to the C–N stretching vibration on the aromatic amine.11 The out-of-plane bending vibrations at 769 cm−1 and 665 cm−1 belong to the O–H bending group and Zr–O vibrations on the benzene ring.28 The broad and strong peaks at 3417, 3432, 3400, 3411, and 3428 cm−1 of the curves in Fig. 3b–f correspond to the O–H vibrational absorption peaks of CMC. In Fig. 3d–f, the peak at 1262 cm−1 is considered to be the C–N stretching vibration on the benzene ring in the MOF, which confirms the presence of the MOF in 0.2CUI.11 The peak width of the PEI-modified composites is more comprehensive than that of CMC, which may be due to the overlap of the N–H vibrational peaks of PEI and the O–H vibration absorption peaks of CMC.29 After PEI modification, it was observed in the spectrum of the 0.2CUI nanocomposite that the absorption bands of the asymmetric stretching vibration and symmetric stretching vibration of sodium carboxymethyl cellulose at 1613 cm−1 and 1423 cm−1 disappeared. It is the formation of intermolecular hydrogen bonds between –COOH and –NH2 of PEI which may cause the crosslinking of –COOH and La ions in CMC to be destroyed during the PEI modification process. The stability of the beads is enhanced after PEI modification, possibly attributed to the formation of hydrogen bonds between them, resulting in improved stability compared to the previous state.30 After the adsorption reaction, 0.2CUI-P has two distinct characteristic peaks at 613 cm−1 and 538 cm−1. They correspond to O
P–O and O–P–O, respectively, indicating that the prepared 0.2CUI composite successfully adsorbed phosphate.31
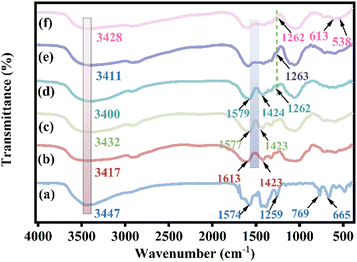 |
| Fig. 3 FTIR spectra of UiO-66–NH2 (a), CMC (b), CL (c), CLU (d), 0.2CUI (e) and 0.2CUI-P (f). | |
3.2.3 Surface properties and micro-structure of the membrane.
In order to gain a deeper understanding of the structural characteristics of the adsorbent, nitrogen adsorption–desorption isotherm analysis and pore size distribution analysis were carried out for the composites CL, CLU, 0.2CUI, and 0.2CUI-P, as shown in Fig. 4a. For detailed data, please refer to Table S1.† As can be seen from the curves shown in Fig. 4a, they represent typical type IV isotherms with H3 hysteresis loops, indicating the presence of mesoporous structures in these materials.32 As shown in Fig. S2,† the isotherm of the as-prepared sample UiO-66–NH2 conforms to the type I adsorption–desorption isotherm, and at high relative pressure above 0.8, no noticeable condensation and hysteresis are observed, indicating that the composite adsorbent has a wide range of microporous structures. The presence of micropores can augment the interaction between guest ions and the host pores, while simultaneously acting as ion reservoirs.33 After mixing with CMC, it is found that the specific surface area of the prepared adsorbent has decreased significantly. After the PEI modification, the specific surface area of the 0.2CUI adsorbent showed an increase, which can be potentially attributed to the PEI modification process. It is likely that the hydrogen bonds formed in the solution were disrupted, leading to alterations in the specific surface area and pore volume.26 Simultaneously, the data analysis reveals that following the adsorption experiment, the specific surface area and pore volume of 0.2CUI were significantly reduced, indicating the successful attachment of phosphate to the composite material. This finding is consistent with the previous SEM results. In conclusion, 0.2CUI has a high specific surface area and excellent pore structure, which is conducive to the rapid migration and capture of phosphate in the adsorbent pores.
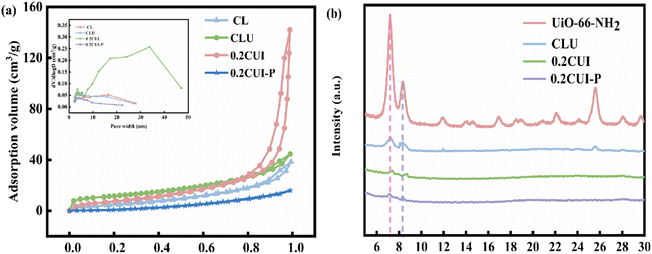 |
| Fig. 4 (a) N2 adsorption–desorption isotherms and pore size distributions; (b) XRD patterns of CLU, 0.2CUI, 0.2CUI-P and UiO-66–NH2. | |
3.2.4 Crystal structure analysis.
As shown in Fig. 4(b), the X-ray diffraction spectra of CLU, 0.2CUI, 0.2CUI-P, and UiO-66–NH2 were explored in the scanning range of 2° < 2θ < 30°. The characteristic peak of UiO-66–NH2 can be clearly seen, which is consistent with the description in the literature.34 Therefore, this may indicate that UiO-66–NH2 was successfully added to the adsorbent and maintained a good crystal structure. Then, in the PEI modification stage, it can be seen from the figure that the diffraction peak intensity of UiO-66–NH2 nanoparticles in 0.2CUI is relatively reduced, indicating that its structure has collapsed or chemically degraded during the modification process.
3.2.5 Thermal stability of the adsorbent.
The thermal properties of 0.2CUI pellets were studied by thermogravimetric analysis and differential thermal analysis. The thermogravimetric analysis curve of the composite material at 0.2CUI is shown in Fig. S3.† In a N2 atmosphere, heating from 35 °C to 800 °C, the three degradation stages of weightlessness can be seen from the curve in the figure. In the initial phase, there is a minor reduction in weight between 0 and 200, which is commonly attributed to the evaporation of physisorbed water (≤100) and the removal of functional groups (100–200).35 In the second stage, in the temperature range of 200–420 °C, the degradation rate of the adsorbent is about 36.64 wt%, which is attributed to the removal and dehydroxylation of DMF in the MOF, the polymer chain of CMC breakdown,36 and the decomposition of PEI.27 The last weight loss in the third stage may be related to the decomposition of the organic bridging ligands in the UiO-66–NH2 framework, leading to the collapse of the MOF structure,37 and its final remaining residue is zirconia.38 It can be seen from the thermal weight loss curve that when the temperature reaches 283 °C, the weight loss rate of the composite material is the largest, which indicates that the prepared 0.2CUI composite material has a specific thermal stability, which is conducive to its further practical application.
3.3 Batch experiments
3.3.1 Influence of dosage.
Before investigating the adsorption behavior, the effect of adsorbent dosage on the adsorption behavior was explored. We set the dosage range used, the concentration is 0.25–1.25 g L−1, and the initial concentration is 100 mg L−1. According to Fig. S4,† as the amount of adsorbent increases from 0.25 g L−1 to 1.25 g L−1, Qe decreases with the addition of the dosage. In contrast, the removal efficiency increases from 46.36% to 84.49%. This outcome suggests that when using higher amounts of sorbent, they offer a greater quantity of active sites in comparison to the phosphate content in the liquid solution. Aggregation of adsorbent particles reduces active site availability and surface area for phosphate adsorption, leading to a decreased ability to adsorb phosphates.46 Therefore, the adsorption efficiency and adsorption capacity of 0.02 g of adsorbent for phosphate were 81.3% and 81.29 mg g−1, respectively, which was the optimal dosage for further experiments.
3.3.2 Isotherms.
The adsorption behavior of materials is influenced by the equilibrium concentration of adsorbate and cannot be disregarded in adsorption studies. Therefore, the impact of altering the initial concentration of the phosphorus solution on the adsorption was investigated at a specific temperature, as illustrated in Fig. 5(a). When the temperature is constant, the maximum adsorption capacity of 0.2CUI for phosphate is 293.27 mg P per g. Compared with CL and CLU, the adsorption capacity of 0.2CUI is significantly increased. At the same time, compared with the other two adsorbents, the mechanical stability of 0.2CUI is better, and the adsorption test can be carried out stably. This is because when preparing the adsorption material, PEI is used to modify the initial material, which not only improves its mechanical stability, but also introduces –NH2, and the presence of –NH2 can undergo a protonation reaction with phosphate. The maximum adsorption performance of the adsorbent for phosphate was further evaluated by comparing the maximum adsorption capacity of 0.2CUI to that of other adsorbents documented in the literature (Table 1).
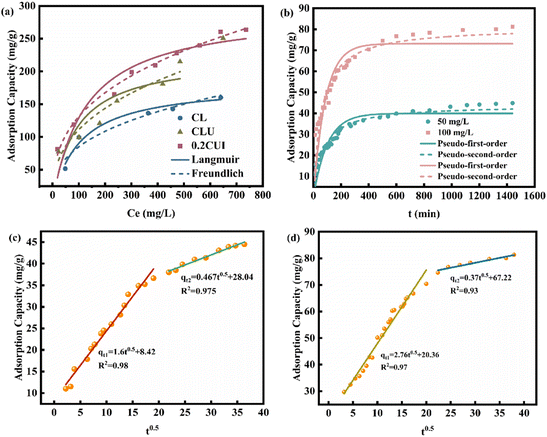 |
| Fig. 5 (a) Adsorption isotherms of phosphate onto CL, CLU, and 0.2CUI; (b) Adsorption kinetics of 0.2CUI at different initial concentrations of phosphate; intraparticle diffusion model of 0.2CUI at (c) 50 mg P per L and (d) 100 mg P per L in the adsorption process. | |
Table 1 Comparison of phosphate adsorption capacities of various adsorbents
Adsorbents |
Concentration range |
Dosage (g L−1) |
Adsorption capacity (mg P per g) |
Ref. |
BC@Ca + Zn |
10–300 mg L−1 |
1.0 |
52.96 |
39
|
ACL |
10–90 mg L−1 |
0.1 |
162 |
40
|
La-FACC |
10–200 mg L−1 |
5.0 |
24.90 |
41
|
Am-Zr/MgFe-LDH |
1–50 mg L−1 |
0.1 |
35.40 |
42
|
CMC/SA/Be/CaCO3 |
100–700 mg L−1 |
0.2 |
90.31 |
4
|
Fe3O4/UiO-66–NH2/CeO2 |
50–800 mg L−1 |
1.0 |
260.82 |
11
|
RB/MgAl2O4@CL+ |
5–500 mg L−1 |
1.0 |
89.65 |
43
|
Zr@MPN-PEI |
5–50 mg L−1 |
1.0 |
35.30 |
44
|
PEIMW@NCMW |
100–500 mg L−1 |
0.5 |
278.89 |
45
|
0.2CUI |
100–1000 mg L−1 |
1.0 |
293.27 |
This work |
The adsorption type of phosphate onto 0.2CUI was investigated using the Langmuir and Freundlich adsorption isotherm models (refer to Table 2 for specific details). Through analysis of the fitting data, it was determined that the Freundlich isotherm model provides a better description of the adsorption process. This model exhibited a higher regression coefficient (R2 > 0.98), indicating a good fit for the adsorption of phosphate onto 0.2CUI. As depicted in Fig. 5(a), while maintaining a constant reaction temperature, it can be observed that as the initial concentration of phosphate increases, the adsorption capacity initially experiences a rapid increase, followed by reaching equilibrium.
Table 2 Fitting parameters for the Langmuir and Freundlich isotherms for phosphate capture by CL, CLU, and 0.2CUI
|
Langmuir isotherm |
Freundlich isotherm |
q
m (mg g−1) |
K
L (L mg−1) |
R
2
|
K
F (mg g−1) |
n
|
R
2
|
CL |
162.4404 |
0.0024 |
0.9344 |
16.7618 |
2.8365 |
0.9046 |
CLU |
223.7873 |
0.0112 |
0.6911 |
20.1446 |
2.6973 |
0.9084 |
CUI |
293.2694 |
0.0079 |
0.8849 |
25.6573 |
2.8242 |
0.9877 |
This phenomenon can be attributed to the equilibrium between the active sites on the adsorbent and the phosphate molecules.
With an increase in phosphate concentration, the adsorption capacity demonstrates a rapid growth. However, the availability of active sites on the adsorbent is limited. Therefore, when the phosphate concentration reaches a certain threshold, the active centers become gradually fully occupied, resulting in a saturation point where the unit adsorption capacity tends to reach a balance.
3.3.3 Kinetics.
The study of adsorption kinetics is crucial in assessing phosphorus removal efficiency and application potential. In this study, we examined the process of phosphate adsorption using three composite materials CL, CLU, and 0.2CUI. Phosphate solutions with concentrations of 50 mg L−1 and 100 mg L−1 were selected, and the adsorption reactions were conducted at various time intervals. Fig. 5(b) illustrates the relationship between the adsorption capacity and contact time for different concentrations of phosphate solutions. As depicted in Fig. 5(b), we have plotted the correlation between the adsorption capacity and contact time for various concentrations of phosphate solutions.
The collected data were subsequently fitted using two traditional kinetic equations, and the fitting parameters are summarized in Table 3. Compared with PFO, PSO (R21 < R22) can better describe the phosphorus loading behavior on different samples, indicating that the adsorption process of phosphate is mainly controlled by chemisorption. In this process, it is likely that there is involvement of electron sharing or ion exchange, rather than a simple surface adsorption process.47 To further identify the adsorption rate-determining step, the diffusion mechanism was derived using the intraparticle diffusion equation. As shown in Fig. 5(c) and (d), 0.2CUI loaded phosphate has undergone two adsorption stages. It is evident that the intercept value C (representing the thickness of the boundary layer) is non-zero, indicating that the resistance posed by the boundary layer cannot be disregarded.48 The rate of phosphate adsorption onto the adsorbent surface is relatively rapid, leading to the occupation of a significant proportion of active sites. The subsequent stage entails the diffusion of phosphate into the adsorbent, displaying a lower slope compared to the initial stage. This suggests that particle effects influence the overall process of phosphate adsorption, encompassing the distribution and regulation of membrane diffusion mechanisms.
Table 3 The kinetic parameters of PFO and PSO models
|
Pseudo-first-order |
Pseudo-second-order |
q (mg g−1) |
K
1 (min−1) |
R
2
|
q (mg g−1) |
K
2 (mg min) |
R
2
|
50 mg L−1 |
39.9475 |
0.0099 |
0.8094 |
43.9552 |
0.0003 |
0.9113 |
100 mg L−1 |
73.2106 |
0.0115 |
0.7779 |
81.1339 |
0.0002 |
0.9011 |
3.3.4 Effect of temperature.
As depicted in Fig. S5,† the adsorption characteristics of 0.2CUI for phosphate were studied at different temperatures (298.15–318.15 K), and the computed thermodynamic data are presented in Table S3.† Analysis of the data shows that the adsorption performance of the adsorbent for phosphate decreases gradually in the temperature range of 298.15–318.15 K. ΔH0 is less than 0, suggesting that the adsorption process of phosphate is exothermic. The negative value of ΔS0 for the reaction indicates that during phosphorus adsorption, the degree of disarrangement at the solid solution interface decreases with increasing temperature.49 In addition, it was shown that removal of phosphate by 0.2CUI was a spontaneous process. Simultaneously, the decrease in the absolute value of ΔG0 with temperature indicates a gradual weakening of the spontaneous tendency of the reaction, and an increase in temperature will impact the positive movement of adsorption.
3.3.5 Comparative effects of 0.2CUI on adsorption selectivity and regeneration study.
It is well known that the presence of additional anions (usually higher than phosphate ion concentrations) in natural wastewater can seriously affect the adsorption behaviour of phosphate adsorbents. We selected Cl−, NO3− and SO42− these three anions for coexistence ion experiments. To examine their influence on the adsorption process of phosphate, we introduced four concentrations of competing ions (0, 0.1, 1, and 2 g L−1) to coexist with phosphate during the adsorption process. The results of the experiments are presented in Fig. 6a. The study revealed that the presence of Cl− and NO3− had a negligible impact on the adsorption of phosphate. This suggests that these competing ions primarily interacted with the outer surface of the adsorbent, thereby reducing the accessible adsorption sites for phosphate. Consequently, the adsorption of phosphate by the adsorbent remained unaffected. Although the adsorption performance is slightly reduced, it does not inhibit the adsorption of phosphate by forming complexes.50 The presence of SO42− ions has a more pronounced effect on the adsorption of phosphate, which can be attributed to the occurrence of a ligand exchange reaction between sulfate ions and the hydroxyl groups on the surface of the adsorbent. This reaction results in the formation of a surface complex that exhibits relative stability and is resistant to decomposition. As a result, it hinders the adsorption of phosphorus, dramatically reducing the adsorption performance of the adsorbent for phosphate.51 Overall, since each component in 0.2CUI has a certain affinity for phosphate, it shows good adsorption selectivity for phosphate. Cyclability is an essential indicator for applying adsorbents in practice, and good cycle performance can greatly reduce the cost consumption in the adsorption process. Therefore, we performed five cycles of adsorption experiments on this adsorbent in this work. 0.02 g adsorbent was dissolved in 100 mg L−1 phosphate solution in 100 ml, stirring continuously for 24 hours to conduct the adsorption experiment. Then, it was washed with deionized water, and sodium hydroxide, hydrochloric acid, and sodium chloride solution were used to perform desorption experiments for 3 hours. After desorption, it was washed to neutral, freeze-dried at −55 °C, and put into subsequent use. After five cycles of repeated experiments, the experimental findings are depicted in Fig. 6b. From the figure, it is evident that the 0.2CUI composite material exhibits a slight decrease in its phosphate removal capacity with each successive cycle. However, it still retains a certain level of adsorption capacity for phosphate. Therefore, the regenerated composite has the potential to be reused many times as a phosphate remover.
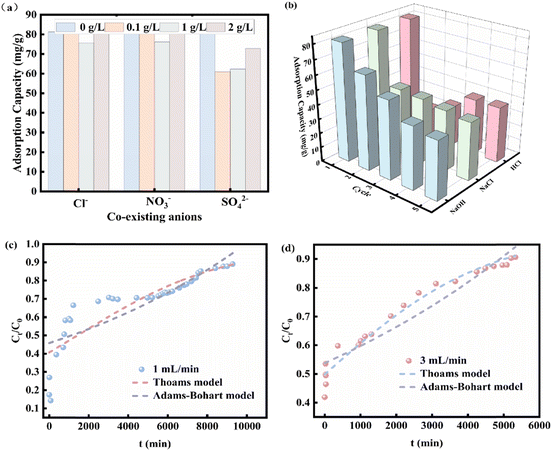 |
| Fig. 6 (a) Effects of co-existing anions; (b) reusability of 0.2CUI on phosphate capture; breakthrough curves of phosphate adsorption on 0.2CUI beads predicted by Thomas and Adams–Bohart models: (c) 1 mL min−1 and (d) 3 mL min−1. | |
3.3.6 Fixed-bed column studies.
Building upon the remarkable cycling performance of 0.2CUI beads in phosphate adsorption, we evaluated their dynamic adsorption capabilities and practical suitability in a continuous flow environment using a custom-designed fluid filter device. Two dynamic adsorption models were utilized to analyze the experimental data, generating breakthrough curves for phosphate in wastewater under different flow rates (1 mL min−1 and 3 mL min−1) with a concentration of 50 mg L−1. Fig. 6(c and d) and Table S2† show the breakthrough curves predicted by the two models, and the correlations of the parameters are given. The findings indicate that, at a concentration of 50 mg L−1, the saturation time of adsorption lengthens as the flow rate decreases. This is due to the shorter residence time of the fluid in the fixed bed column when the feed flow rate is higher. However, in such instances, the mass transfer resistance in the outer membrane of the adsorbent surface tends to decrease, resulting in a reduced time required to reach saturation.52 Based on Table S2,† we observed that the Thomas model provided a more accurate prediction of the breakthrough curve, with a higher correlation coefficient (R2).
3.4 Adsorption mechanism
3.4.1 Effect of pH.
The pH value in natural water bodies is influenced by various factors, including local geographical conditions and external factors. The fluctuation in pH value can potentially affect the efficiency of phosphorus removal by the adsorbent. Additionally, the pH value of the solution influences the potential of the adsorbent surface and determines the form in which the phosphate species is present.
Under different pH conditions, phosphate exists in various ion forms, as shown in Fig. S6.† When the pH < 2.12, it mainly exists in the form of H3PO4; when the pH gradually increases from 2.12 to 7.21, it exists in the form of H2PO4−; when the pH gradually increases from 7.21 to 12.31, the structure is HPO42−; when the pH > 12.31, the main form is PO43−.53
To investigate the influence of the pH value on the adsorption process, we adjusted the pH of 100 mg L−1 phosphate solution using sodium hydroxide or hydrochloric acid solution, ranging from 3 to 11. Subsequently, we conducted experiments. As shown in Fig. 7(a), when the pH value increased from 3 to 4, the adsorption performance of 0.2CUI for phosphate remarkably improved, reaching its maximum adsorption capacity of 81.29 mg g−1 at pH = 4. However, in general, the adsorption performance of 0.2CUI for phosphate is hardly affected within the pH range of 3–11. In other words, the adsorption process of the adsorbent in this study is not influenced by variations in pH. Additionally, we noticed a rise in the pH of the solution after adsorption. This can be attributed to the ligand exchange reaction taking place between phosphate on the adsorbent surface and the subsequent release of OH− into the solution. At the same time, the OH− released by the ligand exchange competes further with the phosphate, leading to a slight reduction in the adsorption capacity of 0.2CUI for phosphate. Therefore, the adsorption capacity of 0.2CUI for phosphate is slightly reduced. On the other hand, the existence form of phosphate changes through the change of pH. Its affinity for HPO42− is low for lanthanum ions, so its adsorption capacity will decrease.54 Under acidic conditions, the degree of protonation of the amine groups (–NH, –NH2) in 0.2CUI will increase, and the adsorption performance for phosphate can be improved through electrostatic attraction.
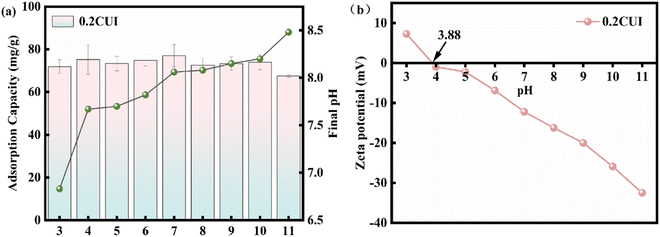 |
| Fig. 7 (a) Impact of initial pH on adsorption capacity and resulting pH in phosphate adsorption; (b) zeta potentials of 0.2CUI. | |
The zero-charge point of 0.2CUI was determined through zeta potential analysis, as shown in Fig. 7(b). The value of the zero potential point of 0.2CUI was found to be 3.88. It was observed that the zeta potential value gradually decreased as the pH value increased. When the pH value of the solution exceeds the pHPZC value, it hinders the adsorption of anionic pollutants due to deprotonation (excessive OH− in the solution). On the other hand, due to protonation (the solution contains excess H+), it is conducive to the loading of negative ion pollutants on its surface.55 Upon analyzing the experimental data, we made a fascinating discovery: the adsorption capacity did not decrease but rather exhibited a slight enhancement.
3.4.2 Elemental spectral analysis.
To investigate the adsorption process of 0.2CUI composites for phosphate, Fig. 8(a) shows the XPS spectrum of 0.2CUI before and after phosphate adsorption. The figure reveals that the P 2p spectrum appeared at 132.08 eV after the adsorption, providing evidence of effective phosphate adsorption onto 0.2CUI. Furthermore, we examined the changes in the N 1s, O 1s, Zr 3d, and La 3d bands before and after adsorption. The N 1s spectra of 0.2CUI before and after phosphate adsorption are depicted in Fig. 8(b). Through analysis, before the reaction, there are two peaks at 400.5 eV and 398.4 eV, which are mainly attributed to –NH2 and –N
;35 however, after loading phosphate, the binding energy of –NH2 decreased significantly from 400.5 eV to 400.34 eV, and a new peak appeared at 401.6 eV, which was attributed to –NH3+.56 After protonation of the amino group, there is a more obvious adsorption effect on phosphate, indicating that the adsorption of phosphate depends on electrostatic attraction. The spectra of La 3d before and after adsorption are depicted in Fig. 8(c), and the peaks of the La 3d3/2 and La 3d5/2 spectra of the 0.2CUI composite change from 854.43 eV, 850.82 eV, 837.6 eV, and 834.1 eV, respectively, to 854.6 eV, 850.98 eV, 837.8 eV, and 834.2 eV after the phosphate adsorption, shifting toward higher binding energies, indicating the formation of La–P complexes on the composites.5Fig. 8(d) shows the spectrum changes of Zr 3d before and after adsorption. The prominent peaks obtained from the 3d3/2 and 3d5/2 spectra can be decomposed into two extremes, 183.9 eV and 181.4 eV, in the spectrum of Zr 3d. The binding energy corresponds to the Zr–O bond, and the peaks at 183.1 eV and 180.08 eV correspond to the Zr–Zr bond, hence suggesting the presence of Zr4+.57,58 Upon analyzing the changes in the Zr 3d spectrum after the reaction, it was found that the binding energies belonging to the Zr–O bond changed to 184 eV and 181.9 eV, respectively. This indicates that electron transfer mainly occurs between the Zr–O bonds. The O 1s spectra are shown in Fig. 8(e), revealing characteristic peaks of La–O–P and Zr–O–P at 531.82 eV after adsorption. These peaks indicate that the phosphates and La and Zr are bonded together by inner-sphere covalent bonds. The mechanism of phosphate adsorption on 0.2CUI is illustrated in Fig. 9.
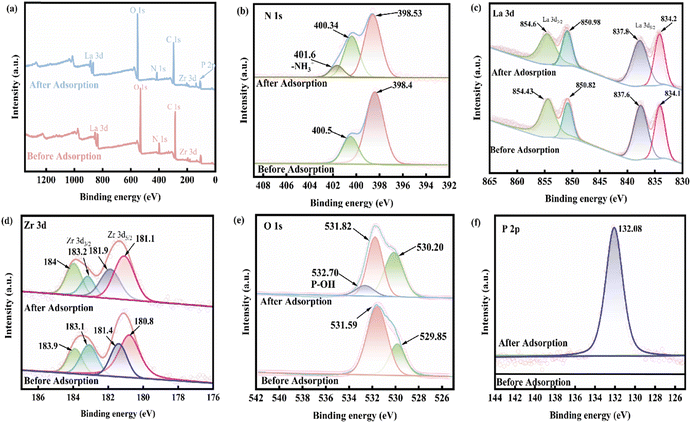 |
| Fig. 8 (a) The XPS total survey spectra of 0.2CUI and 0.2CUI-P and (b) N 1s, (c) La 3d, (d) Zr 3d, (e) O 1s and (f) P 2p spectra before and after 0.2CUI adsorption of phosphate. | |
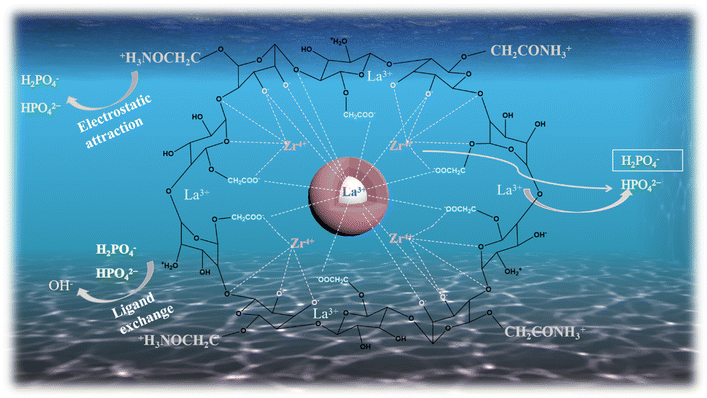 |
| Fig. 9 Schematic diagram of the phosphate adsorption mechanism on 0.2CUI. | |
4. Conclusion
In this study, we prepared an adsorbent with excellent adsorption properties and good recoverability. TGA analysis demonstrated that 0.2CUI exhibits commendable thermal stability. Furthermore, data from the reaction at different temperatures confirmed the spontaneous exothermic reaction during the phosphate adsorption reaction. The adsorption of phosphate by 0.2CUI follows the pseudo-second-order kinetic model and the Freundlich isotherm model. Furthermore, we determined its maximum adsorption capacity for phosphate to be 293.27 mg g−1, which is more excellent compared to other reported adsorbents. In the dynamic adsorption experiments, the adsorption behavior of phosphate on the 0.2CUI beads in the column can be more accurately described using the Thomas model, with a higher R2 compared to the Adams–Bohart model. The analysis of coexisting ions revealed that 0.2CUI exhibits favorable adsorption selectivity and can resist interference from other ions while adsorbing phosphate. After undergoing five cycles of adsorption testing, the adsorbent retained a significant capacity for adsorbing phosphate. Systematic experiments have proven that 0.2CUI is a promising material for treating solutions. This is mainly due to its notable mechanical stability, impressive adsorption properties, high selectivity towards phosphates, cost-effectiveness as a raw material, straightforward preparation process, and significant potential for recycling. Ligand exchange, electrostatic attraction, and ion exchange are the primary adsorption mechanisms that synergistically enhance the adsorption efficiency of 0.2CUI for phosphate. Therefore, it is a viable candidate material for remediating phosphates in water.
Author contributions
Yuyang Liu: conceptualization, methodology, software, formal analysis, writing – original draft, investigation; Shangru Zhai: writing – review & editing, supervision; Qingda An: investigation, visualization; Chang-sik Ha: supervision; Kairuo Zhu: supervision, project administration; Zuoyi Xiao: supervision, investigation; Jingai Hao: supervision; Xiaoling Dong: supervision.
Conflicts of interest
The authors declare that they have no known competing financial interests or personal relationships that could have appeared to influence the work reported in this paper.
Acknowledgements
Financial support from the National Natural Science Foundation of China (U22A20424 and 22178037) and the Liaoning Revitalization Talents Program (XLYC2002114) is highly appreciated.
Notes and references
- Y. Liu, D. Zhong, Y. Xu, H. Chang, L. Dong, Z. Han, J. Li and N. Zhong, Adsorption of phosphate in water by La/Al bimetallic-organic frameworks-chitosan composite with wide adaptable pH range, J. Environ. Chem. Eng., 2023, 11, 110309 CrossRef CAS.
- H. Mahadevan, K. A. Krishnan, R. R. Pillai and S. Sudhakaran, Assessment of urban river water quality and developing strategies for phosphate removal from water and wastewaters: Integrated monitoring and mitigation studies, SN Appl. Sci., 2020, 2, 14 CrossRef.
- H. Mahadevan, V. V. Dev, K. A. Krishnan, A. Abraham and O. C. Ershana, Optimization of retention of phosphate species onto a novel bentonite–alum adsorbent system, Environ. Technol. Innovation, 2018, 9, 1–15 CrossRef.
- J. Fu, J. X. Yap, C. P. Leo and C. K. Chang, Carboxymethyl cellulose/sodium alginate beads incorporated with calcium carbonate nanoparticles and bentonite for phosphate recovery, Int. J. Biol. Macromol., 2023, 234, 123642 CrossRef CAS PubMed.
- C. Zhang, X. Wang, X. Wang and B. Liu, Characterization of La–Mg-modified palygorskite and its adsorption of phosphate, J. Environ. Chem. Eng., 2022, 10, 107658 CrossRef CAS.
- S. Dong, X. Li, S. Wang, D. Zhang, Y. Chen, F. Xiao and Y. Wang, Adsorption-electrochemical mediated precipitation for phosphorus recovery from sludge filter wastewater with a lanthanum-modified cellulose sponge filter, Sci. Total Environ., 2023, 898, 165545 CrossRef CAS.
- R. Mohammadi, M. Hezarjaribi, D. L. Ramasamy, M. Sillanpää and A. Pihlajamäki, Application of a novel biochar adsorbent and membrane to the selective separation of phosphate from phosphate-rich wastewaters, Chem. Eng. J., 2021, 407, 126494 CrossRef CAS.
- L. Zhang, X. Huang, G. Fu and Z. Zhang, Aerobic electrotrophic denitrification coupled with biologically induced phosphate precipitation for nitrogen and phosphorus removal from high-salinity wastewater: Performance, mechanism, and microbial community, Bioresour. Technol., 2023, 372, 128696 CrossRef CAS.
- Y. Li, F. Fu, W. Cai and B. Tang, Synergistic effect of mesoporous feroxyhyte nanoparticles and Fe(II) on phosphate immobilization: Adsorption and chemical precipitation, Powder Technol., 2019, 345, 786–795 CrossRef CAS.
- H. Huang, J. Liu, P. Zhang, D. Zhang and F. Gao, Investigation on the simultaneous removal of fluoride, ammonia nitrogen and phosphate from semiconductor wastewater using chemical precipitation, Chem. Eng. J., 2017, 307, 696–706 CrossRef CAS.
- L. Yang, X. Shan, Y. Zhao, Z. Xiao, Q. An and S. Zhai, Efficient phosphate capture of Fe3O4/UiO-66-NH2/CeO2 in wide pH spectrum, Microporous Mesoporous Mater., 2022, 331, 111653 CrossRef CAS.
- H.-R. Yang, S.-S. Li, X.-C. Shan, C. Yang, Q.-D. An, S.-R. Zhai and Z.-Y. Xiao, Hollow polyethyleneimine/carboxymethyl cellulose beads with abundant and accessible sorption sites for ultra-efficient chromium (VI) and phosphate removal, Sep. Purif. Technol., 2021, 278, 119607 CrossRef.
- P. Cheng, Y. Liu, L. Yang, Q. Ren, X. Wang, Y. Chi, H. Yuan, S. Wang and Y.-X. Ren, Phosphate adsorption using calcium aluminate decahydrate to achieve low phosphate concentrations: Batch and fixed-bed column studies, J. Environ. Chem. Eng., 2023, 11, 109377 CrossRef CAS.
- N. M. Mahmoodi, M. Oveisi, M. Bakhtiari, B. Hayati, A. A. Shekarchi, A. Bagheri and S. Rahimi, Environmentally friendly ultrasound-assisted synthesis of magnetic zeolitic imidazolate framework – Graphene oxide nanocomposites and pollutant removal from water, J. Mol. Liq., 2019, 282, 115–130 CrossRef CAS.
- B. Zhang, N. Chen, C. Feng and Z. Zhang, Adsorption for phosphate by crosslinked/non-crosslinked-chitosan-Fe(III) complex sorbents: Characteristic and mechanism, Chem. Eng. J., 2018, 353, 361–372 CrossRef CAS.
- Z. Cui, J. Wu, Y. Xu, T. Wu, H. Li, J. Li, L. Kang, Y. Cai, J. Li and D. Tian, In-situ growth of polyoxometalate-based metal-organic frameworks on wood as a promising dual-function filter for effective hazardous dye and iodine capture, Chem. Eng. J., 2023, 451, 138371 CrossRef CAS.
- M. H. Hassan, R. Stanton, J. Secora, D. J. Trivedi and S. Andreescu, Ultrafast Removal of Phosphate from Eutrophic Waters Using a Cerium-Based Metal-Organic Framework, ACS Appl. Mater. Interfaces, 2020, 12, 52788–52796 CrossRef CAS.
- X. Zhang, M. Liu and R. Han, Adsorption of phosphate on UiO-66-NH2 prepared by a green synthesis method, J. Environ. Chem. Eng., 2021, 9, 106672 CrossRef CAS.
- Q. Tang, Z. Yin, R. Wang, W. Zhu, Z. Zhang, Y. Wang, Z. Yang, F. Liu and W. Yang, New strategy to remove phosphate from low concentration solution by MOFs-modified resin: High affinity and thermal desorption, Chem. Eng. J., 2023, 465, 142864 CrossRef CAS.
- D. Tang, M. Yin, X. Du, Y. Duan, J. Chen and T. Qiu, Wettability tunable conjugated microporous poly(aniline)s for long-term, rapid and ppb level sequestration of Hg(II), Chem. Eng. J., 2023, 474, 145527 CrossRef CAS.
- M. Pourmadadi, E. Rahmani, A. Shamsabadipour, A. Samadi, J. Esmaeili, R. Arshad, A. Rahdar, F. Tavangarian and S. Pandey, Novel carboxymethyl cellulose based nanocomposite: A promising biomaterial for biomedical applications, Process Biochem., 2023, 130, 211–226 CrossRef CAS.
- S. S. Li, Y. L. Song, H. R. Yang, Q. D. An, Z. Y. Xiao and S. R. Zhai, Carboxymethyl cellulose-based cryogels for efficient heavy metal capture: Aluminum-mediated assembly process and sorption mechanism, Int. J. Biol. Macromol., 2020, 164, 3275–3286 CrossRef CAS PubMed.
- B. Yang, F. Han, Y. Bai, Z. Xie, T. Shi, J. Wang and Y. Li, Phosphate removal performance and mechanism of magnesium–lanthanum-modified coal gasification coarse slag, Mater. Today Sustain., 2023, 22, 100357 CrossRef.
- L. L. Hu, B. Hu, L. M. Shen, D. D. Zhang, X. W. Chen and J. H. Wang, Polyethyleneimine-iron phosphate nanocomposite as a promising adsorbent for the isolation of DNA, Talanta, 2015, 132, 857–863 CrossRef CAS.
- V. V. Dev, B. Wilson, K. K. Nair, S. Antony and K. A. Krishnan, Response surface modeling of Orange-G adsorption onto surface tuned ragi husk, Colloid Interface Sci. Commun., 2021, 41, 100363 CrossRef CAS.
- C. Yang, H.-R. Yang, Q.-D. An, Z.-Y. Xiao and S.-R. Zhai, Recyclable CMC/PVA/MIL-101 aerogels with tailored network and affinity sites for efficient heavy metal ions capture, Chem. Eng. J., 2022, 447, 137483 CrossRef CAS.
- Y. Lei, X. Liu, J. Zhang, Z. Dai, X. Zhao and G. Liu, A novel composite (ZIF-8@PEI-CC) with enhanced adsorption capacity and kinetics of methyl orange, J. Solid State Chem., 2023, 318, 123758 CrossRef CAS.
- A. Dastneshan, S. Rahiminezhad, M. Naderi Mezajin, H. Nouri Jevinani, I. Akbarzadeh, M. Abdihaji, R. Qahremani, M. Jahanbakhshi, Z. Asghari Lalami, H. Heydari, H. Noorbazargan and E. Mostafavi, Cefazolin encapsulated UIO-66-NH2 nanoparticles enhance the antibacterial activity and biofilm inhibition against drug-resistant S. aureus: In vitro and in vivo studies, Chem. Eng. J., 2023, 455, 140544 CrossRef CAS.
- H.-R. Yang, S.-S. Li, Q.-D. An, S.-R. Zhai, Z.-Y. Xiao and L.-P. Zhang, Facile transformation of carboxymethyl cellulose beads into hollow composites for dye adsorption, Int. J. Biol. Macromol., 2021, 190, 919–926 CrossRef CAS.
- Z. Zhou, X. Chen, J. Worth, C. Ye, J. Chen and T. Qiu, Hydrogen bond induced acidic liquids for efficient biodiesel production, AIChE J., 2023, 69, 18098 CrossRef.
- H. Kong, J. Wang, G. Zhang, F. Shen, Q. Li and Z. Huang, Synthesis of three-dimensional porous lanthanum modified attapulgite chitosan hydrogel bead for phosphate removal: Performance, mechanism, cost-benefit analysis, Sep. Purif. Technol., 2023, 320, 124098 CrossRef CAS.
- D. Jiang, X. Wang, L. Feng, Y. Yu, J. Hu, X. Liu and H. Wu, Structural insight into the alginate derived nano-La(OH)3/porous carbon composites for highly selective adsorption of phosphate, Int. J. Biol. Macromol., 2022, 200, 172–181 CrossRef CAS PubMed.
- X. Lou, D. Tang, C. Ye, J. Chen and T. Qiu, Conjugated micro-mesoporous poly(aniline)s for ultrafast Hg (II) capture, Sep. Purif. Technol., 2023, 325, 124616 CrossRef CAS.
- X. Sun, J. Liu, H. Wang, Q. Li, J. Zhou, P. Li, K. Hu, C. Wang and B. Jiang, In-situ detection technique for charge transfer behavior of direct Z-scheme BiVO4/UiO-66-NH2 composites during photocatalytic thioanisole conversion, Chem. Eng. J., 2023, 472, 144750 CrossRef CAS.
- S. S. Li, X. L. Wang, Q. D. An, Z. Y. Xiao, S. R. Zhai, L. Cui and Z. C. Li, Upon designing carboxyl methylcellulose and chitosan-derived nanostructured sorbents for efficient removal of Cd(II) and Cr(VI) from water, Int. J. Biol. Macromol., 2020, 143, 640–650 CrossRef CAS PubMed.
- A. S. Khan, M. F. Nasir and A. Murtaza, Study of carboxymethyl cellulose (CMC) coated manganite as potential candidate for magnetic hyperthermia applications, Mater. Chem. Phys., 2022, 286, 126198 CrossRef CAS.
- Y. Cheng and J. Zhang, Facile design of UiO-66-NH2@La(OH)3 composite with enhanced efficiency for phosphate removal, J. Environ. Chem. Eng., 2021, 9, 104632 CrossRef CAS.
- K. F. Alshammari, A. Subaihi, A. Alharbi, M. A. Khalil and A. Shahat, Efficient Dual Sensor Based on Modified NH2-UiO-66(Zr) MOF for Sensitive and Rapid Monitoring of Ultra-trace Arsenic (III) in Aqueous Media, J. Mol. Liq., 2023, 389, 122787 CrossRef CAS.
- Z. Chen, Y. Wu, Y. Huang, L. Song, H. Chen, S. Zhu and C. Tang, Enhanced adsorption of phosphate on orange peel-based biochar activated by Ca/Zn composite: Adsorption efficiency and mechanisms, Colloids Surf., A, 2022, 651, 129728 CrossRef CAS.
- W.-X. Liang, Y. Wei, M. Qiao, J.-W. Fu and J.-X. Wang, High-gravity-assisted controlled synthesis of lanthanum carbonate for highly-efficient adsorption of phosphate, Sep. Purif. Technol., 2023, 307, 122696 CrossRef CAS.
- S. Asaoka, K. Kawakami, H. Saito, T. Ichinari, H. Nohara and T. Oikawa, Adsorption of phosphate onto lanthanum-doped coal fly ash—Blast furnace cement composite, J. Hazard. Mater., 2021, 406, 124780 CrossRef CAS PubMed.
- A. Nuryadin, T. Imai, A. Kanno, K. Yamamoto, M. Sekine and T. Higuchi, Phosphate adsorption and desorption on two-stage synthesized amorphous-ZrO2/Mg–Fe layered double hydroxide composite, Mater. Chem. Phys., 2021, 266, 124559 CrossRef CAS.
- X. Shan, L. Yang, Y. Zhao, H. Yang, Z. Xiao, Q. An and S. Zhai, Biochar/Mg-Al spinel carboxymethyl cellulose-La hydrogels with cationic polymeric layers for selective phosphate capture, J. Colloid Interface Sci., 2022, 606, 736–747 CrossRef CAS PubMed.
- A. A. Aryee, R. Han and L. Qu, Preparation of zirconium functionalized magnetic peanut husk crosslinked with polyethyleneimine and its application for the adsorption of phosphates in aqueous solution, J. Environ. Chem. Eng., 2023, 11, 111281 CrossRef CAS.
- J. Qu, F. Bi, S. Li, Z. Feng, Y. Li, G. Zhang, L. Wang, Y. Wang and Y. Zhang, Microwave-assisted synthesis of polyethylenimine-grafted nanocellulose with ultra-high adsorption capacity for lead and phosphate scavenging from water, Bioresour. Technol., 2022, 362, 127819 CrossRef CAS PubMed.
- S. Adil and J.-O. Kim, The effectiveness and adsorption mechanism of iron-carbon nanotube composites for removing phosphate from aqueous environments, Chemosphere, 2023, 313, 137629 CrossRef CAS PubMed.
- B. Wang, X. Hu, L. Li, H. Wang, H. Huang, R. Wang, D. Zhou, J. Yuan and L. Chen, Application and functionalization of toxic waste sludge-derived biochar for efficient phosphate separation from aqueous media: Toxicity diminution, robust adsorption, and inner mechanism, Chem. Eng. J., 2023, 468, 143745 CrossRef CAS.
- Y. Chang, Z. Wei, X. Chang, G. Ma, L. Meng, T. Liu, L. Yang, Y. Guo and X. Ma, Hollow hierarchically porous La2O3 with controllable multishells: A high-performance adsorbent for phosphate removal, Chem. Eng. J., 2021, 421, 127816 CrossRef CAS.
- J. Wang, G. Zhang, S. Qiao and J. Zhou, Comparative assessment of formation pathways and adsorption behavior reveals the role of NaOH of MgO-modified diatomite on phosphate recovery, Sci. Total Environ., 2023, 876, 162785 CrossRef CAS PubMed.
- P. Madhusudan, C. Lee and J.-O. Kim, Synthesis of Al2O3@Fe2O3 core–shell nanorods and its potential for fast phosphate recovery and adsorption of chromium (VI) ions from contaminated wastewater, Sep. Purif. Technol., 2023, 326, 124691 CrossRef CAS.
- J. Yan, M. Ma, K. Liu, Y. Bao and F. Li, Anchoring NH2-MIL-101(Fe/Ce) within Melamine Sponge Boosts Rapid Adsorption and Recovery of Phosphate from Water, ACS ES&T Engg, 2023, 3, 467–478 Search PubMed.
- V. Jurado-Davila, J. T. D. Oliveira, D. Estumano and L. A. Féris, Fixed-bed column for phosphate adsorption combining experimental observation, mathematical simulation, and statistics: Classical and Bayesian, Sep. Purif. Technol., 2023, 317, 123914 CrossRef CAS.
- J. Wang, G. Zhang, S. Qiao and J. Zhou, Magnetic Fe0/iron oxide-coated diatomite as a highly efficient adsorbent for recovering phosphorus from water, Chem. Eng. J., 2021, 412, 128696 CrossRef CAS.
- Z. Lan, Y. Lin and C. Yang, Lanthanum-iron incorporated chitosan beads for adsorption of phosphate and cadmium from aqueous solutions, Chem. Eng. J., 2022, 448, 137519 CrossRef CAS.
- M. Imran, M. M. Iqbal, J. Iqbal, N. S. Shah, Z. U. H. Khan, B. Murtaza, M. Amjad, S. Ali and M. Rizwan, Synthesis, characterization and application of novel MnO and CuO impregnated biochar composites to sequester arsenic (As) from water: Modeling, thermodynamics and reusability, J. Hazard. Mater., 2021, 401, 123338 CrossRef CAS PubMed.
- X. Liu, W. Cheng, Y. Yu, S. Jiang, Y. Xu and E. Zong, Magnetic ZrO2/PEI/Fe3O4 functionalized MWCNTs composite with enhanced phosphate removal performance and easy separability, Composites, Part B, 2022, 237, 109861 CrossRef CAS.
- R. Zhang, B. Du, Q. Li, Z. Cao, G. Feng and X. Wang, α-Fe2O3 nanoclusters confined into UiO-66 for efficient visible-light photodegradation performance, Appl. Surf. Sci., 2019, 466, 956–963 CrossRef CAS.
- D. Liu, Y. Li, C. Liu and Y. Zhou, Facile preparation of UiO-66@PPy nanostructures for rapid and efficient adsorption of fluoride: Adsorption characteristics and mechanisms, Chemosphere, 2022, 289, 133164 CrossRef CAS PubMed.
|
This journal is © The Royal Society of Chemistry 2024 |
Click here to see how this site uses Cookies. View our privacy policy here.