DOI:
10.1039/D3FO02740F
(Paper)
Food Funct., 2024,
15, 79-95
The amelioration of a purified Pleurotus abieticola polysaccharide on atherosclerosis in ApoE−/− mice†
Received
7th July 2023
, Accepted 12th November 2023
First published on 13th November 2023
Abstract
In this study, a polysaccharide known as PAPS2 was eluted from Pleurotus abieticola fruiting bodies using 0.1 M NaCl solutions. PAPS2 has a Mw of 19.64 kDa and its backbone is mainly composed of →6)-α-D-Galp-(1→, →6)-β-D-Glcp-(1→ and →2,6)-α-D-Galp-(1→ residues, and its branches mainly end with β-D-Manp-(1→, which is attached at C2 of →2,6)-α-D-Galp-(1→. PAPS2 elicited several effects in high-fat diet (HFD)-fed ApoE−/− mice. It significantly reduced the body weight, liver index, and serum levels of total cholesterol (TC) and triglycerides (TGs), and it alleviated lipid accumulation in the aorta. Intestinal microflora analysis showed that PAPS2 suppressed the abundances of Adlercreutzia, Turicibacter, and Helicobacter and enriched that of Roseburia. It also influenced lipid metabolism, suggesting that it reduced the levels of TGs, lysophosphatidylcholine (LPC), phosphatidylcholine (PC), and ceramide (Cer). Moreover, it suppressed oxidative response by increasing nuclear factor erythroid 2 (Nrf2)-related factor expression and activating the antioxidant enzymes superoxide dismutase (SOD) and glutathione peroxidase (GSH-Px) to reduce the level of reactive oxygen species (ROS). Meanwhile, it showed anti-inflammatory effects partially related to the inhibition of toll-like receptor 4 (TLR4)/nuclear factor kappa-B (NF-κB) signaling induced by lipopolysaccharide (LPS) in RAW 264.7 cells, as well as in the aorta of HFD-fed ApoE−/− mice. This study provides experimental evidence of the auxiliary applicability of PAPS2 in atherosclerosis treatment.
1 Introduction
Atherosclerotic disease is a lipid-driven multifocal immunoinflammatory disease of the arteries,1 and is divided into early and advanced lesions.2 Advanced lesions develop into atheromas with necrotic or lipid cores in the center covered by a fibrous cap and complicated by stenosis and calcification, which eventually leads to organ ischemia.3 Active cholesterol storage and innate and adaptive immune responses are accepted explanations for the formation of lesions in atherosclerosis.4 Many kinds of cells, such as endothelial cells, intimal smooth muscle cells, and leukocytes, are involved in the development of atherosclerosis.5
Monocytes differentiate into macrophages within the intima through the endothelial gap to mediate the oxidative modification of low-density lipoprotein (LDL). This leads to the accumulation of intracellular lipids and the formation of foam cells,6 which are important for the formation of atherosclerotic plaques. Moreover, an imbalance in oxidative stress causes macrophage polarization, promoting atherosclerosis.7 LDL at high levels tends to accumulate in the sub-endothelial space of the aorta wall and induce inflammatory responses by forming oxidized LDL following oxidative modifications, leading to atherosclerosis.8
The intestinal microflora and their host lipid metabolism play important roles in the progression of atherosclerosis.9 Unsaturated fatty acids, such as fish oil, modulate the intestinal microflora by enhancing the microbial production of short-chain fatty acids (SCFAs) and lowering the production of trimethylamine oxide (TMAO) to alleviate atherogenesis.10 Lipid metabolism disorders, such as fat production and decomposition, play a fundamental role in the pathogenesis of atherosclerosis.11 Mice with apolipoprotein E (ApoE) deficiency when fed a high-fat diet (HFD) showed a significant increase in plasma levels of total cholesterol (TC), which induced the rapid development of arterial plaques; hence, ApoE−/− mice are more widely used.12
3-Hydroxy-3-methylglutaryl-CoA reductase inhibitors (statins), such as pravastatin,13 simvastatin,14 and rosuvastatin,15 are used to slow the progression of atherosclerosis, but they only modestly reduce stenosis. Drug development for atherosclerosis is challenging, especially in developing compounds with novel underlying mechanisms with the potential to regulate immune responses and improve the balance between pro-inflammatory and pro-resolving processes in plaque inflammation.16 Hence, lifestyle modifications are another useful approach for reducing the progression of atherosclerosis. Fungi that are generally rich in nutrients, especially polysaccharides, have been reported to exert various pharmacological effects including antitumor,17 immunomodulatory,18 antioxidant,19 and hypolipidemic effects.20 Low-molecular-weight fucoidan ameliorated atherosclerotic areas and exerted a significant antioxidant effect that led to the mitigation of atherosclerosis in ApoE−/− mice.21
Pleurotus species are widely cultivated worldwide as food, and their production accounts for a quarter of the total output of commercial mushrooms.22P. eryngii showed an anti-atherosclerotic effect in ApoE-deficient mice.23P. abieticola is distributed in northeastern and northwestern China and Russia and has been successfully cultivated;24 few studies have reported its biological activity. Earlier, we extracted a water-soluble polysaccharide from the fruiting bodies of P. abieticola, known as PAPS1, which exhibits immune-enhancing activity by promoting cellular immune response.25
In this study, we extracted another polysaccharide PAPS2 from the fruiting bodies of P. abieticola through elution with 0.1 M NaCl solutions. Its molecular weight (Mw), monosaccharide composition, and glycosidic linkage were systemically analyzed. One-dimensional and two-dimensional Nuclear Magnetic Resonance (NMR) was further performed to confirm the structural characterization. The amelioration of the atherosclerotic effects of PAPS2 was investigated in HFD-fed ApoE−/− mice, which may be related to the regulation of the intestinal microflora and lipidomics. Our study provides a reference for the use of P. abieticola in the adjuvant treatment for atherosclerosis.
2 Materials and methods
2.1 Isolation and purification of PAPS2
As in our previous study,25 the fruiting bodies of P. abieticola were cultured at Jilin Agricultural University (Changchun, Jilin Province, China), dried, crushed, and subjected to extraction using double-distilled (D.D.) water. Crude polysaccharides were collected after alcohol precipitation, deproteinization, centrifugation, and dialysis.
The lyophilizate was dissolved in D.D. water, passed through a diethylaminoethylcellulose-52 (DEAE-52) anion exchange column, and eluted first with distilled water and then with 0.1, 0.3, and 0.5 M NaCl solutions at 3.0 mL min−1. HiPrep 26/60 Sephacryl S-400 High Resolution (HR) and HiLoad 16/600 Superdex 200 preparative columns were used for further purification of the polysaccharide eluted with the 0.1 M NaCl solution. Finally, the PAPS2 polysaccharide was obtained for further experiments. All batch numbers of reagents and instrument parameters were the same as those in our previous study.25
2.2 Structural and composition analyses of PAPS2
As in our previous studies, the total sugar and protein contents in PAPS2 were detected using phenol–sulfuric acid colorimetry and bicinchoninic acid (BCA) assay (23225, Thermo Fisher Scientific, Carlsbad, USA), respectively.26 The Mw and homogeneity of PAPS2 were determined using a gel permeation chromatography system coupled online to a multi-angle laser light scattering system.27 The monosaccharide components of the hydrolyzed PAPS2 were detected using a high-performance liquid chromatography system.25 After derivatization including methylation and acetylation, gas chromatography-mass spectrometry (GC-MS) was performed for glycosidic linkage information analysis of PAPS2.25
As in our previous study,28 PAPS2 was dissolved in D2O, followed by filtration and transfer to an NMR tube. 1H and 13C NMR spectra were recorded using a Bruker Avance AV600 NMR spectrometer (600 MHz for 1H, 151 MHz for 13C; Bruker, Germany). NMR data were processed using MestReNova-14.0.0-23239 (Mestrelab Research, Santiago de Compostela, Spain).
2.3 Cell culture and treatment
The murine macrophage cells RAW 264.7 (CL-0190) were obtained from Procell Life Science & Technology Co., Ltd (Wuhan, China). RAW 264.7 cells were cultured in Dulbecco's modified Eagle's medium (DMEM) (Thermo Fisher Scientific), supplemented with 15% fetal bovine serum, 100 μg mL−1 streptomycin, and 100 units per mL penicillin at 37 °C in a 5% CO2 incubator.
RAW 264.7 cells were pretreated with PAPS2 (50 μg mL−1) for 3 h at 37 °C under 5% CO2 and then co-incubated with lipopolysaccharide (LPS, 1 μg mL−1) for another 15 h.
2.4 Animals and treatment
The animal experimental procedures in this study were approved by the Institutional Animal Ethics Committee of Jilin Agricultural University (approval no. 2021-06-03-002). They complied with the ARRIVE guidelines and were performed according to the National Research Council's Guide for the Care and Use of Laboratory Animals (8th edition).
Six male C57BL/6 and 18 male ApoE−/− mice (8 weeks old, 18–22 g, specific pathogen-free grade) were purchased from Liaoning Changsheng Biotechnology Co., Ltd (license no.: SCXK (Liao) 2020-0001; Liaoning, China). All mice were maintained under the following conditions: temperature, 23 ± 1 °C; humidity, 50 ± 10%; and 12 h light/dark cycle (7:00 a.m. to 7:00 p.m.). After acclimatization for 7 days, the ApoE−/− mice were fed an HFD (21% fat and 0.5% cholesterol) for the next 8 weeks, whereas the six C57BL/6 mice were fed a normal chow diet for the same period. The mice were provided food and water ad libitum throughout the experimental period.
The six C57BL/6 mice were orally administered normal saline (n = 6) (serving as the control group, Ctrl). The 18 ApoE−/− mice were randomly divided into three groups (n = 6), and each day for the next 4 weeks each group orally received normal saline (serving as the HFD group), 100 mg kg−1 PAPS2 dissolved in D.D. water (serving as the HFD + PAPS2 group), and 3 mg kg−1 rosuvastatin calcium (RC; 147098-20-2; Beijing Solarbio Science & Technology Co., Ltd., Beijing, China) dissolved in D.D. water (serving as the HFD + RC group), respectively. The body weight of the mice was monitored weekly (Fig. 2A).
After the last administration, all the mice were fasted for 12 h with free access to water. Peripheral blood was obtained by sampling the retro-orbital venous plexus and stored in a refrigerator at −80 °C. The mice were anesthetized by an intraperitoneal injection of 3% pentobarbital and received a 15–20 mL cardiac perfusion with normal saline. Cecal samples were collected and immediately snap-frozen in liquid nitrogen. The organs (liver, spleen, lungs, kidneys, and aorta) were dissected and weighed. The tissues were divided into two parts: one part was snap-frozen in liquid nitrogen and stored at −80 °C for further biochemical analysis, and the other part was fixed in 4% paraformaldehyde solution for histological analysis.
2.5 Histopathological analysis
The fixed tissues (n = 3 in each group) from the liver, spleen, lungs, kidneys, and aorta were dehydrated, embedded in paraffin, and cut into 5 μm sections. Hematoxylin and eosin (H&E) and Oil Red O staining was performed as described in our previous study.29,30
2.6 Biochemical analysis
The serum was collected twice by centrifugation at 3500 rpm for 5 min. Aortic tissues were homogenized in normal saline, and the protein concentrations of the samples were detected using a BCA kit (23225, Thermo Fisher Scientific).
The levels of low-density lipoprotein (LDL) (A113-1-1), triglycerides (TGs) (A110-1-1), high-density lipoprotein (HDL) (A112-1-1), and TC (A111-1-1) in the serum, and the levels of malondialdehyde (MDA) (A003-1), superoxide dismutase (SOD) (A001-3-2), and glutathione peroxidase (GSH-Px) (A005-1-2) in the serum and aorta tissue (n = 6 in each group) were measured using commercially available assay kits purchased from Nanjing Jiancheng Biotechnology Co., Ltd (Jiangsu, China).
The levels of reactive oxygen species (ROS) (ml 009876-1), interleukin 1β (IL-1β) (ml 301814), IL-18 (ml 002294), tumor necrosis factor α (TNF-α) (ml 002095), NOD-like receptor thermal protein domain associated protein 3 (NLRP3) (ml 037234), IL-6 (ml 063160), ceramide (Cer) (ml 037499), and lysophosphatidylcholine (LPC) (ml 001938) in the mouse serum and aorta (n = 6 in each group) were analyzed using enzyme-linked immunosorbent assay (ELISA) kits purchased from Shanghai Enzyme Link Biotechnology Co., Ltd (Shanghai, China).
2.7 Intestinal microflora analysis
Samples were randomly selected from the Ctrl, HFD, and HFD + PAPS2 groups (n = 4 in each group). Intestinal microflora analysis was performed using 16S rDNA sequencing with an Illumina MiSeq platform at Shanghai Personalbio Technology Co., Ltd (Shanghai, China), as previously published.31 Sequences with ≥97% similarity were considered to be the same operational taxonomic units (OTUs). Various analyses, including alpha and beta diversity, were performed using the QIIME software package. Abundance data from each group of microbiota were used to generate a species composition heatmap and for linear discriminant analysis (LDA) effect size (LEfSe) analysis. The LEfSe analysis was considered more reliable when the LDA score was >2.0.
2.8 Lipidomics analysis
Serum samples were randomly selected from the Ctrl, HFD, and HFD + PAPS2 groups (n = 4 in each group). As in the procedure of our previous study,27 lipidomics analysis was performed using liquid chromatography-mass spectrometry at Shanghai Personalbio Technology Co., Ltd (Shanghai, China). The processed data were subjected to orthogonal partial least squares discriminant analysis (OPLS-DA) for metabolite variation analysis.
2.9 Immunohistochemical staining analysis
As per the procedure in our previous study,32 aortic paraffin sections from the Ctrl, HFD, HFD + PAPS2, and HFD + RC groups (n = 3 in each group) were dewaxed, hydrated, washed, sealed (3% H2O2; 10 min), washed again (PBS; 3 times) at 4 °C, treated with primary antibodies including anti-nuclear factor erythroid 2-related factor (Nrf2) (ab31163; dilution, 1
:
100; Abcam (Shanghai) Trading Co., Ltd, Shanghai, China), anti-IL-1β (ab283818; dilution, 1
:
500; Abcam), and anti-nuclear factor kappa-B (NF-κB) p65 (phosphor Ser536) (p-NF-κB) (ab76302; dilution, 1
:
250; Abcam), anti-toll-like receptor 4 (TLR4) (ab22048; dilution, 1
:
100; Abcam), and anti-NLRP3 (ab263899; dilution, 1
:
200; Abcam), incubated overnight, and re-stained with hematoxylin and HRP-labeled goat anti-rabbit (H + L) secondary antibodies (E-AB-1003; dilution, 1
:
2000; Elabscience, Houston, TX, USA) at 4 °C. All the slides were observed under an optical microscope (BX51; Olympus, Tokyo, Japan).
2.10 Western blotting
The collected RAW 264.7 cells were homogenized and lysed in ice-cold RIPA lysis buffer (PC101; Epizyme, Shanghai, China), containing a cocktail of protease and phosphatase inhibitors (P002; NCM Biotech, Jiangsu, China). Protein concentrations of the samples were detected using a BCA kit (23225, Thermo Fisher Scientific).
As per the procedure in a previous study of western blotting33 a total of 40 μg lysates (n = 3 in each group) were separated using 10% (for a range of 30–110 kDa) and 12.5% (for a range of 10–50 kDa) dodecyl sulfate, sodium salt polyvinylidene fluoride membranes (0.45 μm; Merck Millipore, MA, USA). After blocking in 5% BSA for 3 h at room temperature, the bands were incubated with primary antibodies (detailed information shown in Table S1†) overnight at 4 °C, and horseradish peroxidase-conjugated secondary antibodies (Table S1†) for 2.5 h at 4 °C. Protein bands were imaged using ultra-high sensitivity ECL kits (GK10008; GlpBio, CA, USA) via a chemiluminescence image analysis system (QuickChemi 5200; Monad (Wuhan) Biotech Co., Ltd, Wuhan, China). Quantitation of protein bands was performed using ImageJ 6.0 software (National Institutes of Health, Bethesda, Maryland, USA).
2.11 Statistical analysis
All biochemical indices were processed using SPSS Statistics 26 (IBM, Armonk, New York, USA) and GraphPad Prism 9.0 (GraphPad Software, USA) was used to draw the graphs. Data are presented as mean ± standard deviation (S.D.). More than two different groups (three or four) were compared using one-way analysis of variance (ANOVA), followed by Tukey's post hoc test. p < 0.05 was considered statistically significant for all analyses. Significance boundaries are indicated as ns (not significant), *p < 0.05, **p < 0.01, and ***p < 0.001.
In intestinal microflora analysis (n = 4 in each group), alpha diversity was analyzed using Quantitative Insights into Microbial Ecology version 2 (2019.4) using the Kruskal–Wallis rank-sum test and Dunn's test as post hoc tests. Beta diversity was calculated using the Bray–Curtis method.
In lipidomics analysis (n = 4 in each group), statistically significant differences were analyzed by a t-test (p < 0.05) of metabolite levels, and a fold change ≥1.5 or ≤0.667 was regarded as the standard for differential lipids to draw heatmaps and correlation plots.
3 Results
3.1 Purification and composition analysis of PAPS2
PAPS-B was isolated and eluted using a DEAE-52 column with 0.1 M NaCl solution (Fig. 1A) and further purified using Sephacryl S-400 HR (Fig. 1B). The collected PAPS-B2 was purified using Superdex 200 to obtain PAPS2 (Fig. 1C). PAPS2 had few UV absorption peaks in the range of 260–280 nm (Fig. S1A†), indicating low levels of proteins and nucleic acids. PAPS2 was 92.7% carbohydrate and 0.31% protein in composition.
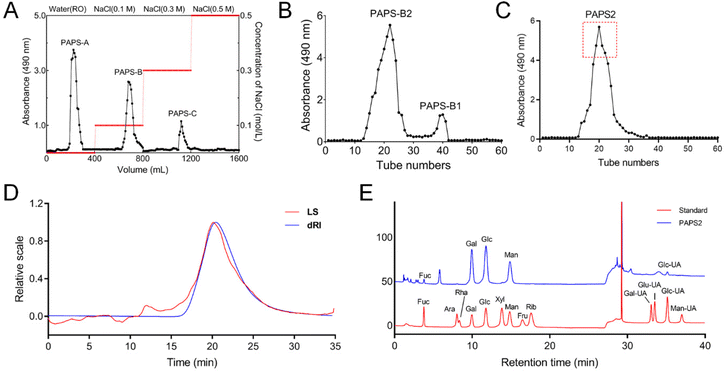 |
| Fig. 1 Structural and composition analyses of PAPS2. Isolation and purification of PAPS2 using (A) DEAE-52 with distilled water and then with 0.1, 0.3, and 0.5 M NaCl solutions at 3.0 mL min−1, (B) Sephacryl S-400 column, and (C) Superdex 200 prep grade column. (D) Molecular weight analysis of PAPS2. The blue line represents the response of the refractive index detector (dRI), and the red line represents the response of laser light scattering (LS). (E) The monosaccharide composition of PAPS2. The red line represents the standard monosaccharide mixture, and the blue line represents PAPS2. Fuc, fucose; Ara, arabinose; Rha, rhamnose; Gal, galactose; Glc, glucose; Xyl, xylose; Man, mannose; Fru, fructose; Rib, ribose; Gal-UA, galacturonic acid; Gul-UA, guluronic acid; Glc-UA, glucuronic acid; Man-UA, mannuronic acid. | |
The Mw/number-average molecular weight (Mn) was 1.750, and the Mw of PAPS2 was estimated to be 19.64 kDa (Fig. 1D and Table S2†). An FT-IR analysis was performed to identify the characteristic functional groups of PAPS2 (Fig. S1B†). The broad peaks at 3419.32 cm−1 belonged to the stretching vibration of –OH, which is also regarded as the characteristic peak of sugars. The signal at 2926.35 cm−1 represented asymmetric C single bond H stretching and bending vibrations in the molecules.34 The broad peaks at 1646.17 cm−1 and 1246.19 cm−1 belonged to the stretching vibrations of the C double bond O and C single bond O, respectively.
PAPS2 was composed of the monosaccharides Fuc (2.50%), Gal (37.98%), Glc (31.45%), Man (26.20%), and Glc-UA (1.8%) (Fig. 1E and Table S3†). Based on the retention times and standard data in the Complex Carbohydrate Research Center spectral database for partially methylated alditol acetate, PAPS2 was mainly composed of four glycosidic residues: 1,5-di-O-acetyl-2,3,4,6-tetra-O-methyl mannitol (t-Man(p), 27.20%), 1,5,6-tri-O-acetyl-2,3,4-tri-O-methyl glucitol (6-Glc(p), 13.53%), 1,5,6-tri-O-acetyl-2,3,4-tri-O-methyl galactitol (6-Gal(p), 26.16%), and 1,2,5,6-tetra-O-acetyl-3,4-di-O-methyl galactitol (2,6-Gal(p), 23.33%) (Table 1 and Fig. S2†).
Table 1 The methylation analysis of PAPS2
Retention time (min) |
Linkage pattern |
Methylated sugar |
Characteristic ions (m/z) |
Relative mole percentage (%) |
6.744 |
t-Fuc(p) |
1,5-Di-O-acetyl-6-deoxy-2,3,4-tri-O-methyl fucitol |
59, 72, 89, 102, 115, 118, 131, 162, 175 |
1.55 |
8.567 |
t-Man(p) |
1,5-Di-O-acetyl-2,3,4,6-tetra-O-methyl mannitol |
59, 71, 87, 102, 113, 118, 129, 145, 161, 162, 205 |
27.20 |
11.858 |
3-Glc(p) |
1,3,5-Tri-O-acetyl-2,4,6-tri-O-methyl glucitol |
59, 71, 87, 101, 118, 129, 143, 161, 174, 203, 217, 234, 277 |
1.65 |
12.080 |
2-Glc(p) |
1,2,5-Tri-O-acetyl-3,4,6-tri-O-methyl glucitol |
59, 74, 87, 101, 113, 129, 145, 161, 170, 190, 215 |
3.85 |
13.404 |
6-Glc(p) |
1,5,6-Tri-O-acetyl-2,3,4-tri-O-methyl glucitol |
59, 71, 87, 99, 102, 118, 129, 143, 162, 189, 203, 233 |
13.53 |
15.145 |
6-Gal(p) |
1,5,6-Tri-O-acetyl-2,3,4-tri-O-methyl galactitol |
59, 71, 87, 99, 102, 118, 129, 143, 159, 162, 189, 203, 233 |
26.16 |
17.417 |
3,6-Glc(p) |
1,3,5,6-Tetra-O-acetyl-2,4-di-O-methyl glucitol |
59, 74, 87, 101, 118, 129, 139, 160, 174, 189, 202, 234, 305 |
2.73 |
19.311 |
2,6-Gal(p) |
1,2,5,6-Tetra-O-acetyl-3,4-di-O-methyl galactitol |
60, 74, 87, 100, 114, 130, 143, 160, 174, 190, 204, 234 |
23.33 |
The one-dimensional 1H and 13C NMR spectra of PAPS2 were identified (Fig. 2). The 1H spectrum showed that anomeric hydrogen signals were mainly between 3.3 and 5.3 ppm (Fig. 2A). Four main signals were found at 5.06, 4.92, 4.72, and 4.43 ppm, respectively, indicating PAPS2 has both α- and β-anomeric configurations.35
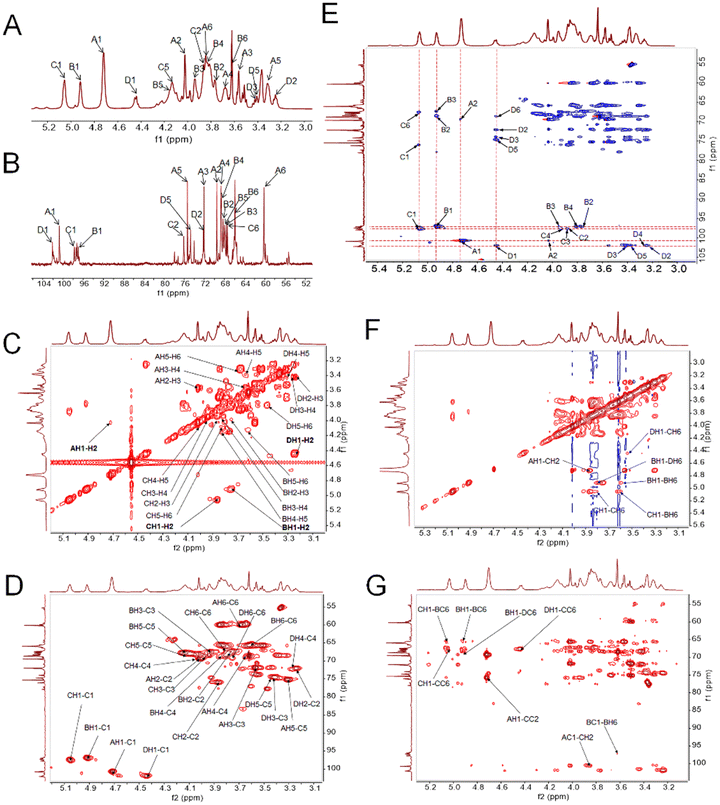 |
| Fig. 2 Structural characterization of PAPS2 using NMR. One-dimensional NMR atlas of (A) 1H (600 MHz), and (B) 13C NMR (151 MHz). Two-dimensional NMR atlas of (C) COSY, (D) HSQC, (E) HSQC-TOCSY, (F) NOESY, and (G) HMBC. | |
The 13C NMR spectra of PAPS2 were carried out in a region ranging from 55–103 ppm (Fig. 2B). According to the literature data as well as the results of methylation and monosaccharide composition, four main anomeric protons could be labelled as A–D, which were identified as β-D-Manp-(1→ (4.72/100.73 ppm),37 →6)-α-D-Galp-(1→ (4.92/97.24 ppm),42 →2,6)-α-D-Galp-(1→ (5.06/97.76 ppm)42 and →6)-β-D-Glcp-(1→ (4.43/102.06 ppm),43 respectively.
Two-dimensional NMR atlas were further analyzed to elucidate the PAPS2 structural features. In the 1H–1H correlation spectroscopy (COSY) spectrum, the signals at 4.72/4.03, 4.03/3.56, 3.56/3.67, 3.67/3.31, 3.31/3.84 and 3.31/3.70 ppm were observed and assigned to residue A (Fig. 2C and Table 2). Cross peaks at 4.92/3.76, 3.76/3.94, 3.94/3.85, 3.85/4.15, and 4.15/3.62 ppm belonged to the H–H signals of residue B (Fig. 2C and Table 2). Similarly, signals at 3.87, 3.98, 4.02, 4.10, 3.82 and 3.54 ppm, and 4.43, 3.24, 3.43, 3.26, 3.42 and 3.76 ppm were assigned to H2–H6 of residues B and C, respectively (Fig. 2C and Table 2). Combined with the literature reports and heteronuclear single quantum correlation (HSQC) spectrum, the cross peaks at 4.72/100.73, 4.03/69.51, 3.56/72.03, 3.67/68.68, 3.31/75.32 and 3.70/60.19 ppm were assigned to H1/C1–H6/C6 of residue A, respectively (Fig. 2D and Table 2). Similar to residue A, the signals of residues B, C, and D were also assigned (Fig. 2D and Table 2), which were consistent with the cross peaks in the HSQC – total correlation spectroscopy (TOCSY) spectrum (Fig. 2E). According to the spectra of the heteronuclear multiple bond correlation (HMBC) and nuclear Overhauser effect spectroscopy (NOESY), the proton–proton and proton–carbon signal bond correlations were analyzed. The signals at 4.72/3.87 ppm in NOESY (Fig. 2F) and 4.72/76.06 ppm in the HMBC (Fig. 2F) proved the presence of A1–C2, indicating that β-D-Manp-(1→ was attached at C2 of →2,6)-α-D-Galp-(1→. Similarly, BH1 showed proton–proton signals with its own H6 and DH6, indicating the presence of –B–B– and –B–D– linkages (Fig. 2F). DH1–CH6 (4.43/3.54 ppm), CH1–CH6 (5.06/3.82 ppm), and CH1–BH6 (5.06/3.62 ppm) were observed in NOESY (Fig. 2F), respectively. The same proton–carbon signal bonds were also observed in the HMBC, indicating the presence of –D–C–, –C–C–, and –C–B– linkages (Fig. 2G).
Table 2
1H and 13C NMR chemical shifts for PAPS2
|
Glycosyl residues |
H1/C1 |
H2/C2 |
H3/C3 |
H4/C4 |
H5/C5 |
H6/C6 |
Ref. |
A |
β-D-Manp-(1→ |
4.72 |
4.03 |
3.56 |
3.67 |
3.31 |
3.70/3.84 |
36 and 37 |
100.73 |
69.51 |
72.03 |
68.68 |
75.32 |
60.19 |
B |
→6)-α-D-Galp-(1→ |
4.92 |
3.76 |
3.94 |
3.85 |
4.15 |
3.62 |
38 and 39 |
97.24 |
68.28 |
67.62 |
68.6 |
67.98 |
65.86 |
C |
→2,6)-α-D-Galp-(1→ |
5.06 |
3.87 |
3.98 |
4.02 |
4.10 |
3.82/3.54 |
38 and 40 |
97.76 |
76.06 |
69.14 |
69.21 |
68.85 |
67.44 |
D |
→6)-β-D-Glcp-(1→ |
4.43 |
3.24 |
3.43 |
3.26 |
3.42 |
3.76 |
39 and 41 |
102.06 |
72.17 |
74.67 |
71.85 |
74.75 |
69.39 |
In conclusion, the backbone of PAPS2 mainly contains →6)-α-D-Galp-(1→, →6)-β-D-Glcp-(1→ and →2,6)-α-D-Galp-(1→ residues, and its branches mainly end with β-D-Manp-(1→, which was attached at C2 of →2,6)-α-D-Galp-(1→.
3.2 Alleviation of atherosclerosis by PAPS2 in HFD-fed ApoE−/− mice
A schematic representation of the animal experimental protocol is shown in Fig. 3A. After a 4-week treatment, the body weights of HFD-fed ApoE−/− mice increased by 10.9% (p < 0.001) (Fig. 3B) compared with that of the Ctrl mice. PAPS2 and RC significantly reduced the body weights by 14.3% (p < 0.001) and 12.4% (p < 0.001), respectively (Fig. 3B). PAPS2 also reversed the increase of the liver index (p < 0.05) (Fig. 3C) in the mice.
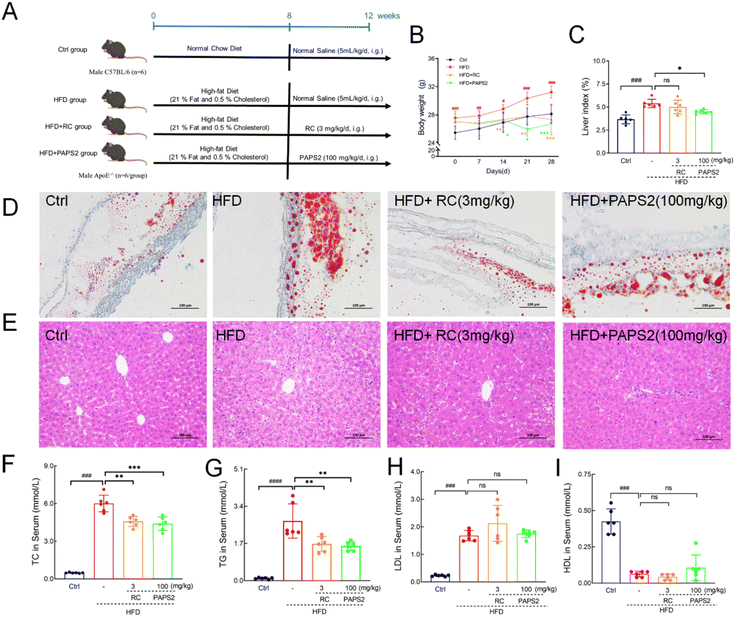 |
| Fig. 3 PAPS2 relieved dyslipidemia in HFD-fed ApoE−/−mice. (A) A schematic of the experimental protocol. PAPS2 suppressed the enhancement of (B) body weight and (C) the liver index in HFD-fed ApoE−/− mice (n = 6). Histopathological analysis of (D) the aorta using Oil Red O staining (n = 3; ×200; scale bar: 100 μm) and (E) the liver using H&E staining (n = 3; ×200; scale bar: 100 μm). PAPS2 suppressed the increased levels of (F) TC and (G) TGs, but not that of (H) LDL (n = 6). It failed to modulate the low levels of (I) HDL in the serum (n = 6) of HFD-fed ApoE−/− mice. Data are presented as mean ± S.D., and were analyzed using one-way ANOVA, followed by Tukey's post hoc test. ns, not significant, #p < 0.05, ##p < 0.01, and ###p < 0.001, compared with the Ctrl mice, *p < 0.05, **p < 0.01, and ***p < 0.001, compared with the vehicle-treated HFD-fed ApoE−/− mice. | |
Compared with that in the Ctrl mice, a significant increase in lipid accumulation was observed in HFD-fed ApoE−/− mice, which was strongly alleviated by PAPS2 (Fig. 3D). PAPS2 and RC reduced lipid accumulation (Fig. 3D) and vacuoles in the liver (Fig. 3E) induced by the HFD. No significant changes were noted in the spleen and kidneys among the experimental groups (Fig. S3A and C†). PAPS2 and RC prevented inflammatory infiltration in the lungs (Fig. S3B†).
Compared with that in the Ctrl group, HFD-fed ApoE−/− mice showed 12.0-fold enhanced TC (p < 0.001) (Fig. 3F), 24.8-fold enhanced TGs (p < 0.001) (Fig. 3G), and 6.2-fold enhanced LDL (p < 0.001) (Fig. 3H); HDL decreased by 85% (p < 0.001) (Fig. 3I). In contrast, RC and PAPS2 reduced the TC levels by 20.5% (p < 0.01) (Fig. 3F) and 25.8% (p < 0.001) (Fig. 3F), respectively, and the TG levels by 38.5% (p < 0.01) (Fig. 3G) and 41.9% (p < 0.01) (Fig. 3G), respectively; however, they failed to regulate the serum LDL (p > 0.05) (Fig. 3H) and HDL (p > 0.05) (Fig. 3I) levels.
3.3 PAPS2 regulates the intestinal microflora in HFD-fed ApoE−/− mice
The 16S rRNA sequences that had ≥97% similarity were clustered into OTUs. At the phylum level, the main composition of the intestinal microflora consisted of Firmicutes (76.36%) and Bacteroidetes (10.05%) in vehicle-treated HFD-fed ApoE−/− mice (Fig. 3A). PAPS2 decreased Firmicutes abundance to 65.12% and increased that of Bacteroidetes to 23.41% (Fig. 4A), reversing the imbalance in the intestinal microflora caused by the HFD. According to the Venn diagram, among the 9929 detected OTUs, 715 OTUs were shared by the Ctrl, HFD, and HFD + PAPS2 groups (Fig. 4B). In contrast, 2564 (25.8%), 2554 (25.7%), and 2910 (29.3%) unique OTUs were found in the Ctrl, HFD, and HFD + PAPS2 groups, respectively (Fig. 4B).
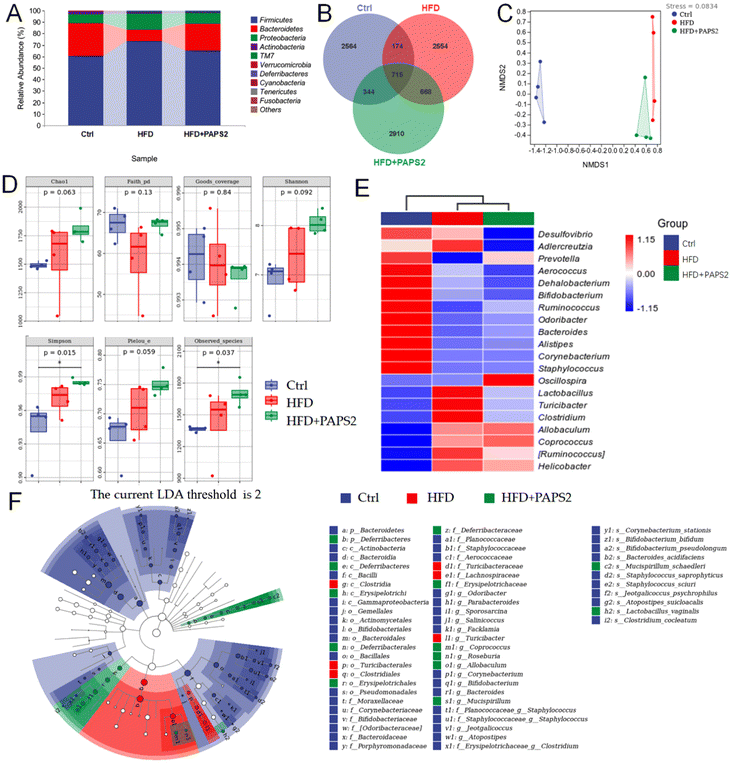 |
| Fig. 4 PAPS2 regulated the intestinal microflora in HFD-fed ApoE−/− mice. (A) The relative abundance of species at the phylum level. (B) Venn diagram. (C) NMDS of unweighted UniFrac distance from the beta diversity analysis. Beta diversity was calculated using the Bray–Curtis method. (D) Chao1, Faith's phylogenetic diversity (Faith PD), Good's coverage, Shannon's and Simpson's indices, Pielou's evenness, and the observed species index values from the alpha diversity analysis. Data were analyzed using the Kruskal–Wallis rank-sum test and Dunn's test as post hoc tests. ns, not significant, *p < 0.05, compared with the Ctrl mice. (E) Heatmap of 20 bacterial genera with the most significantly different abundance, calculated from the unweighted UniFrac distance of the cecal content samples. The levels of (F) the taxonomic cladogram of distribution of the LDA values of significantly different species according to the LEfSe analysis. Data are presented as mean ± S.D. (n = 4). | |
This indicates a relatively large difference in the intestinal microflora composition among the groups. Consistent with the results of the Venn diagram, the beta diversity results showed a specific separation of the microbial composition among the three groups (stress = 0.0834) (Fig. 4C). Compared with that in the vehicle-treated HFD-fed ApoE−/− mice, PAPS2 failed to regulate the indices of alpha diversity such as Chao1, Faith's phylogenetic diversity, Good's coverage, Shannon's index, Simpson's index, Pielou's evenness, and the observed species index (Fig. 4D). A heatmap of the top 20 bacterial genera that presented the most significantly different abundances is listed in Fig. 4E.
In HFD-fed ApoE−/− mice, PAPS2 strongly reduced the relative abundances of Adlercreutzia, Turicibacter, and Helicobacter, and enhanced those of Prevotella and Bacteroides (Fig. 4E). The microbial taxa with significant changes in abundance were identified using LEfSe analysis. Among the three groups, Ruminococcus gnavus was one of the most specific dominant nodes in vehicle-treated HFD-fed ApoE−/− mice (p < 0.05, LDA > 2.0) (Fig. 4F and Table S4†). PAPS2-treated HFD-fed ApoE−/− mice showed an enrichment in Roseburia (p < 0.01, LDA > 2.0) (Fig. 4F and Table S4†).
3.4 PAPS2 regulates lipid metabolism in HFD-fed ApoE−/− mice
In total, 10 subclasses and 2049 individual lipids were detected. More than half of these lipids belonged to TGs (30.9%) and phosphatidylcholine (PC) (22.7%) (Fig. 5A). According to OPLS-DA, the metabolite variation in the serum showed significant differences among the three groups (Fig. 5B).
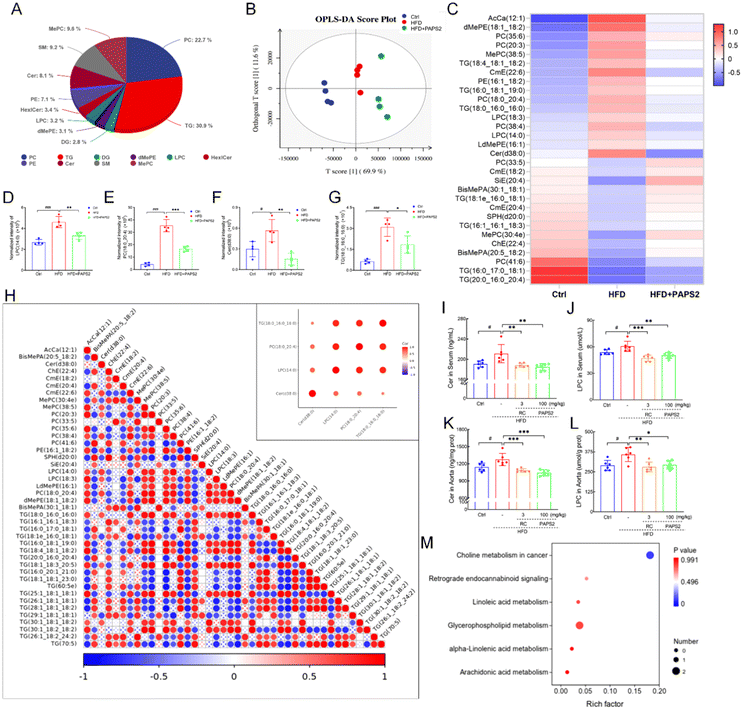 |
| Fig. 5 PAPS2 regulated lipid metabolism in HFD-fed ApoE−/− mice. (A) The pie plot of the detected lipids in total. (B) OPLS-DA score plot (n = 4). (C) Heatmap of significantly altered metabolites. The normalized intensity of the representative lipids, (D) LPC (14:0), (E) PC (18:0_20:4), (F) Cer (d38:0), and (G) TG (18:0_16:0_16:0) affected by PAPS2 (n = 4). Data are presented as mean ± S.D., and were analyzed using a t-test. (H) Correlation analysis on the association with the mentioned lipids. The levels of (I and K) Cer and (J and L) LPC in the serum and aorta of each group (n = 6). Data are presented as mean ± S.D., and were analyzed using one-way ANOVA, followed by Tukey's post hoc test. (M) KEGG pathway enrichment analysis. #p < 0.05 and ###p < 0.001, compared with the Ctrl mice, *p < 0.05, **p < 0.01, and ***p < 0.001, compared with the vehicle-treated HFD-fed ApoE−/− mice. | |
The top 30 lipids that were significantly regulated are shown in Fig. 5C. The normalized intensities of LPC (14:0) (p < 0.001) (Fig. 5D), PC (18:0_20:4) (p < 0.001) (Fig. 5E), Cer (d38:0) (p < 0.05) (Fig. 5F), and TGs (18:0_16:0_16:0) (p < 0.001) (Fig. 5G) were increased in the vehicle-treated HFD-fed ApoE−/− mice, but were significantly reversed by PAPS2 (p < 0.05) (Fig. 5D–G). The correlation between the associated lipids in the heatmap is shown in Fig. 5H. Four lipids were significantly positively correlated (Fig. 5H). The levels of Cer and LPC in the serum and aorta were measured using ELISA. Consistent with the results of the lipid metabolism analysis in HFD-fed ApoE−/− mice, PAPS2 reduced the levels of Cer and LPC by 12.4% (p < 0.01) (Fig. 5I) and 16.7% (p < 0.01) (Fig. 5J), respectively, in the serum and by 18.2% (p < 0.001) (Fig. 5K) and 18.0% (p < 0.05) (Fig. 5L) in the aorta, respectively. RC showed regulatory effects similar to those of PAPS2 (p < 0.05) (Fig. 5I–L). PAPS2 affects pathways related to lipid metabolism, particularly choline metabolism, in HFD-fed ApoE−/− mice (Fig. 5M).
3.5 PAPS2 suppressed oxidative response in HFD-fed ApoE−/− mice
In HFD-fed ApoE−/− mice, PAPS2 significantly suppressed the levels of pro-oxidative factors such as ROS by 19.1% in the serum (p < 0.001) (Fig. 6A) and 13.0% in the aorta (p < 0.05) (Fig. 6E) and MDA by 57.5% in the serum (p < 0.05) (Fig. 6B) and 70.7% in the aorta (p < 0.001) (Fig. 6F). It enhanced the levels of anti-oxidative factors such as SOD by 53.5% in the serum (p < 0.001) (Fig. 6C) and 67.4% in the aorta (p < 0.05) (Fig. 6G) and GSH-Px by 43.7% in the serum (p < 0.05) (Fig. 6D) and 125.7% in the aorta (p < 0.05) (Fig. 6H). RC showed similar effects to those of PAPS2, except that it failed to suppress the serum ROS (p > 0.05) (Fig. 6A) and MDA (p > 0.05) (Fig. 6B) levels in HFD-fed ApoE−/− mice.
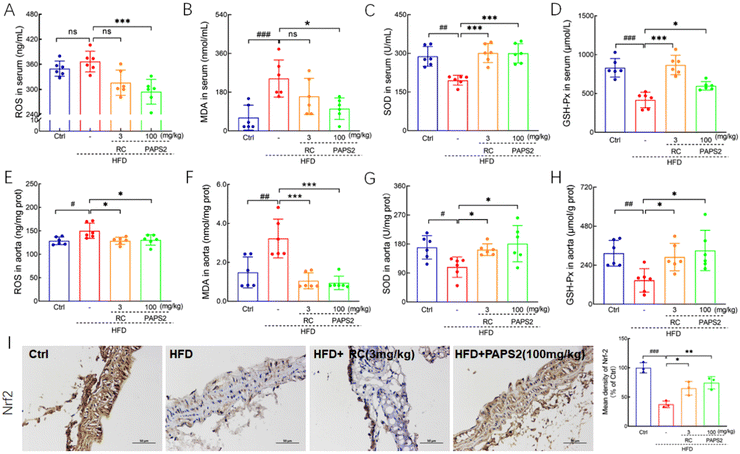 |
| Fig. 6 PAPS2 regulated oxidative stress-related factors in HFD-fed ApoE−/− mice. PAPS2 strongly influenced the levels of (A) ROS, (B) MDA, (C) SOD, and (D) GSH-Px in the serum and (E) ROS, (F) MDA, (G) SOD, and (H) GSH-Px in the aorta (n = 6). (I) PAPS2 enhanced the expression of Nrf2 in the aorta, analyzed by immunohistochemical staining (n = 3; ×100; scale bar: 50 μm). Data are presented as mean ± S.D., and were analyzed using one-way ANOVA, followed by Tukey's post hoc test. ns, not significant, #p < 0.05, ##p < 0.01, and ###p < 0.001, compared with the Ctrl mice, *p < 0.05, **p < 0.01 and ***p < 0.001, compared with the vehicle-treated HFD-fed ApoE−/− mice. | |
The multifunctional regulator Nrf2 is a cytoprotective factor that regulates the expression of genes and their proteins, including Heme Oxygenase 1 (HO-1) and SOD. It exerts beneficial effects by protecting against oxidative injury and modulating atherosclerosis.44 Both RC and PAPS2 significantly enhanced the levels of Nrf2 in the aorta of HFD-fed ApoE−/− mice (p < 0.05) (Fig. 6I).
3.6 PAPS2 suppressed inflammation by modulating TLR4/NF-κB signaling in HFD-fed ApoE−/− mice
Immunohistochemical analysis showed that in HFD-fed ApoE−/− mice, PAPS2 and RC reduced the levels of TLR4 by 51.6% (p < 0.01) and 56.4% (p < 0.001) (Fig. 7A), NLRP3 by 46.0% (p < 0.01) and 52.0% (p < 0.001) (Fig. 7B), IL-1β by 61.5% (p < 0.001) and 54.5% (p < 0.001) (Fig. 7C), and p-NF-κB by 62.1% (p < 0.001) and 55.5% (p < 0.001) (Fig. 7D), respectively. PAPS2 reduced the level of IL-6 by 9.9% (p < 0.05) (Fig. 7F) in the aorta and that of TNF-α by 17.1% (p < 0.001) (Fig. 7G) in the serum and 11.1% (p < 0.01) (Fig. 7H) in the aorta of HFD-fed ApoE−/− mice, whereas RC failed to modulate the TNF-α level (p > 0.05) (Fig. 7G) in the serum. No obvious changes in the IL-6 and TNF-α levels were found between the Ctrl and HFD-fed ApoE−/− groups in the serum (p > 0.05) (Fig. 7E and G). Consistent with the immunohistochemical results, PAPS2 reduced the levels of NLRP3 by 18.4% (p < 0.001) (Fig. 7I) and 10.8% (p < 0.001) (Fig. 7J), IL-1β by 9.9% (p < 0.05) (Fig. 7K) and 11.4% (p < 0.05) (Fig. 7L) and IL-18 by 14.0% (p < 0.001) (Fig. 7M) and 15.6% (p < 0.01) (Fig. 7N), in the serum and aorta of HFD-fed ApoE−/− mice, respectively. Similar effects were exerted by RC (p < 0.05) (Fig. 7), except on the IL-1β level in the serum (p > 0.05) (Fig. 7K).
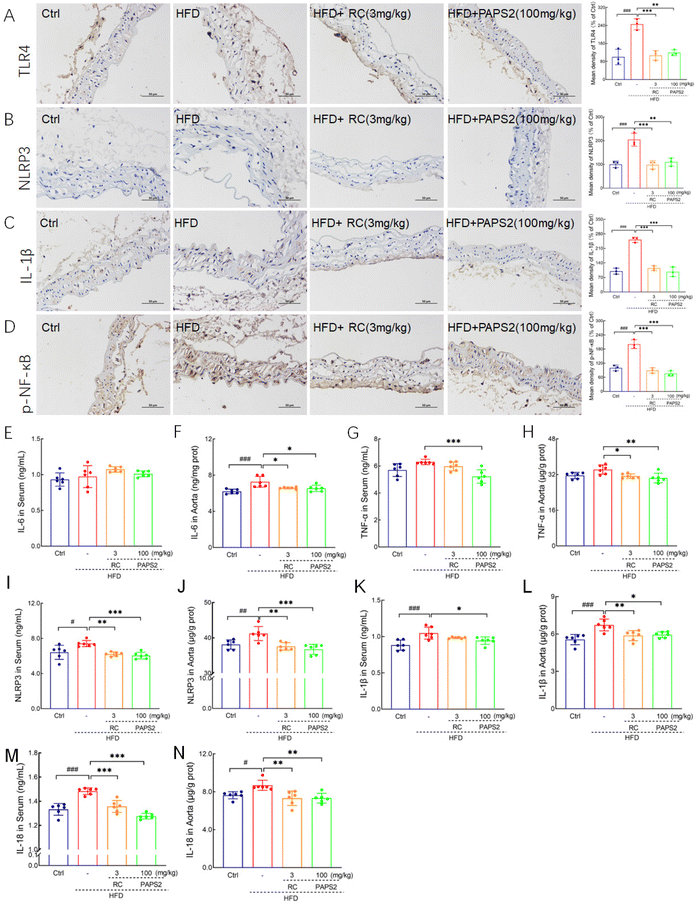 |
| Fig. 7 PAPS2 alleviated NLRP3-induced inflammation related to its suppression in TLR4/NF-κB signaling. PAPS2 strongly suppressed the expressions of (A) TLR4, (B) NLRP3, (C) IL-1β and (D) p-NF-κB in the aorta, analyzed by immunohistochemical staining (n = 3; ×100; scale bar: 50 μm). PAPS2 significantly reduced the levels of (E and F) IL-6, (G and H) TNF-α, (I and J) NLRP3, (K and L) IL-1β, and (M and N) IL-18 in the serum and aorta, analyzed using ELISA (n = 6). Data are presented as mean ± S.D., and were analyzed using one-way ANOVA, followed by Tukey's post hoc test. #p < 0.05, ##p < 0.01 and ###p < 0.001, compared with the Ctrl mice, *p < 0.05, **p < 0.01, and ***p < 0.001, compared with the vehicle-treated HFD-fed ApoE−/− mice. | |
3.7 PAPS2 regulated Nrf2 and TLR4/NF-κB signaling in LPS-exposed RAW 264.7 cells
LPSs can trigger inflammation in atherosclerosis by acting on macrophages.45 RAW 264.7 cells were exposed to LPSs to promote the inflammation response. LPSs reduced the expressions of Nrf2 by 73.6% (p < 0.001), HO-1 by 43.5% (p < 0.001), and SOD1 by 41.1% (p < 0.01), respectively, compared with the non-treated cells (Fig. 8). PAPS2 significantly enhanced the expressions of Nrf2 by 172.1% (p < 0.001), HO-1 by 46.6% (p < 0.01), and SOD1 by 83.9% (p < 0.01), respectively (Fig. 8). Moreover, compared with the non-treated cells, LPS increased the levels of TLR4 by 130.5% (p < 0.01), p-NF-κB/NF-κB by 87.4% (p < 0.01), IL-6 by 274.0% (p < 0.001), TNF-α by 65.1% (p < 0.01), NLRP3 by 80.2% (p < 0.01), IL-1β by 289.5% (p < 0.001), and IL-18 by 159.1% (p < 0.001), which were all strongly reversed by PAPS2 treatment (p < 0.01) (Fig. 8), indicating the alleviation of PAPS2 on oxidative response and inflammation in macrophages.
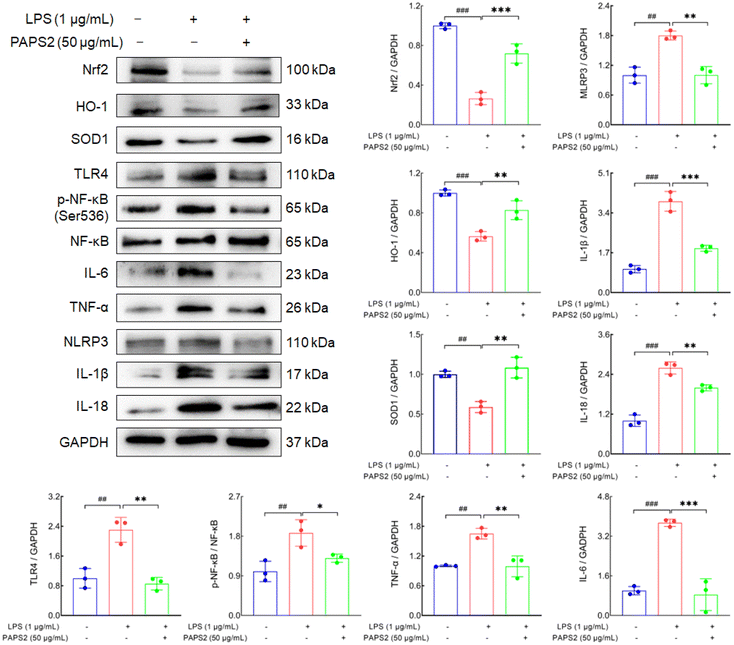 |
| Fig. 8 PAPS2 regulated Nrf2 and TLR4/NF-κB signaling in LPS-exposed RAW 264.7 cells. In LPS (1 μg mL−1)-exposed RAW 264.7 cells, PAPS2 enhanced the expression levels of Nrf2, HO-1, and SOD1, and suppressed the expression levels of TLR4, p-NF-κB, IL-6, TNF-α, NLRP3, IL-1β, and IL-18. Data are presented as mean ± S.D. (n = 3), and were analyzed using one-way ANOVA, followed by Tukey's post hoc test. Quantification data were normalized by GAPDH (n = 3). ##p < 0.01 and ###p < 0.001, compared with the non-treated cells; **p < 0.01 and ***p < 0.001, compared with the LPS-treated cells. | |
4 Discussion
In this study, PAPS2, a polysaccharide with a Mw of 19.64 kDa, was first purified from P. abieticola, and it mainly contains →6)-α-D-Galp-(1→, →6)-β-D-Glcp-(1→ and →2,6)-α-D-Galp-(1→ residues, and its branches mainly end with β-D-Manp-(1→, which is attached at C2 of →2,6)-α-D-Galp-(1→. Accordingly, enzymatic-extractable polysaccharides from Cordyceps militaris consisting of →2,6)-α-D-Galp-(1→ and →6)-β-D-Galp-(1→ showed hepatoprotective effects via regulation of Nrf2/ROS/NF-κB in CCl4-induced mice.46Saussurea involucrate polysaccharides containing →1)-β-D-Galp-(6→ exhibited anti-inflammatory activity by inhibiting pro-inflammatory cytokine expressions via regulation of NF-κB.47 In this study, PAPS2 significantly ameliorated atherosclerotic symptoms in HFD-fed ApoE−/− mice.
ApoE−/− mice fed an HFD (21% fat and 0.5% cholesterol) developed hypercholesterolemia that presented as foam cell lesions and developed into early fibrous lesions by 15 weeks of age.48 Cholesterol and lipids metabolized by the intestinal microflora affect the formation of atherosclerotic plaques. For example, TMAO, which is derived mainly from the oxidation of trimethylamine that is abundant in red meat is involved in atherosclerotic plaque formation.49 Dietary components such as SCFAs are metabolized by the intestinal microflora to produce important signaling molecules to regulate metabolism-associated diseases.50 SCFAs (acetate, propionate, and butyrate) inhibit the activity of histone deacetylases and the production of IL-6 and IL-8 to attenuate the endothelial activation induced by TNF-α or LPS.51 Propionate significantly inhibits TNF-α- and IL-1-induced NF-κB and increases the expression of peroxisome proliferator-activated receptor alpha, which are important steps in the development of atherosclerosis.52 The ratio of Bacteroidetes to Firmicutes—the two most abundant bacteria in healthy mice—is an indicator of the ecological imbalance parameter for diseases.53 The reduced ratio of Bacteroidetes to Firmicutes in HFD-fed ApoE−/− mice was strongly reversed by the PAPS2 treatment. According to the results of the LEfSe analysis, R. gnavus was one of the most specific dominant nodes in vehicle-treated HFD-fed ApoE−/− mice. The abundance of Ruminococcus, which produces SCFAs by fermenting polysaccharides, was found to increase in HFD-induced obese rodent models, which may contribute to the development of obesity by promoting insulin resistance and systemic inflammation.54 PAPS2 increases the abundance of Roseburia, which inhibits microglial activation and reduces the levels of IL-1α, interferon (INF)-γ, and monocyte chemoattractant protein (MCP)-1 by producing propionate and butyrate to reduce inflammation in the brain.55 Colonization with Roseburia inhibited the development of atherosclerotic plaque formation in gnotobiotic mice that were fed a plant polysaccharide diet.56 Thus, PAPS2 might reduce inflammation to alleviate atherosclerosis by regulating the intestinal microflora.
Disturbance of the intestinal microflora usually plays an important role in the occurrence and development of hyperlipidemia and cholesterol metabolic disorder.57 The intestinal microflora may regulate the lipid metabolism of the host. According to lipid metabolism analysis, PAPS2 reduced the increase in serum TG levels in HFD-fed ApoE−/− mice. High TG levels are well-known biomarkers of atherosclerosis due to their association with apolipoprotein C3, which can activate the NLRP3 inflammasome to induce inflammation and promote the development of atherosclerosis.58 A recent study reported that a high level of plasma TG is a major risk for the health of patients with cardiovascular disease, even when LDL-cholesterol levels are low.59 Hence, the reduction in serum TG levels is of great significance in cardiovascular diseases. LPC, which is mainly derived from the turnover of PC in the circulation by phospholipase A2, increases oxidative stress, inhibits endothelial cell migration and proliferation in the vascular system, and promotes inflammation and the development of atherosclerosis.60 The production of Cer stimulated by cytokines such as TNF-α and ROS causes increased uptake of lipoprotein, accumulation of cholesterol within macrophages, endothelial dysfunction, and increased inflammation, which ultimately promote the lesions and plaques of atherosclerosis.61 PAPS2 significantly reduced the levels of PC, LPC, and Cer, and ameliorated atherosclerosis in HFD-fed ApoE−/− mice.
The role of oxidative stress in atherosclerosis progression has been widely described.62 In response to excessive oxidative stress, increasing levels of ROS in endothelial cells cause tissue infiltration and damage the structure and function of endothelial cells.63 Endothelial injury, which is considered the early stage of atherosclerosis, leads to the transfer of lipids and monocytes into the intima of blood vessels, inducing the formation of foam cells and atherosclerotic plaques.44 Nrf2 promotes downstream gene expression and activates antioxidant enzymes such as SOD and GSH-Px, which remove ROS and lipid peroxide products, such as MDA.64 PAPS2 not only decreased the levels of ROS and MDA by increasing Nrf2 expression in the aorta, but also improved expressions of Nrf2, SOD1 and HO-1 in RAW 264.7 cells, confirming its antioxidant effect. Consequently, the enhanced levels and activities of SOD and GSH-Px can prevent the formation of foam cells and atherosclerotic plaques. Furthermore, Nrf2 reduces ROS levels, which alleviates the activation of NF-κB and stimulation of the NLRP3 inflammasome; thus, inflammation is reduced.65,66
Inhibitions of IL-1β and NLRP3 alleviate vascular inflammation and progression of atherosclerosis.67 IL-1β and IL-18 are two cytokines that are regulated by NLRP3 inflammasomes and associated with an increased risk of cardiovascular diseases.68 Initial signals, such as ox-LDL and cholesterol crystals, activate the NF-κB pathway by triggering TLRs to increase the transcription of pro-inflammatory cytokines and NLRP3.69 Activation of the NLRP3 inflammasome recruits macrophages to the aortic wall lesions, which in return induces the formation of foam cells and the development of plaques.70 In RAW 264.7 cells, PAPS2 suppressed the expression levels of TLR4/NF-κB signaling induced by LPS. The NLRP3 inhibitor MCC950 reduces IL-1β and IL-18 production in the aorta and attenuates inflammation and pyroptosis in macrophages to hinder atherosclerosis development.71 Atorvastatin decreases cholesterol production by suppressing the TLR4/myeloid differentiation factor 88 (MyD88)/NF-κB signaling pathway, which reduces the formation of the NLRP3 complex.72,73 PAPS2 reduced the expression levels of TLR4, p-NF-κB, and NLRP3 to alleviate inflammation for ameliorating atherosclerosis in ApoE−/− mice.
There exist limitations to this study. The relationship between the structure and bioactivity, as well as the specific target of PAPS2 is not clear enough and requires further investigation.
5 Conclusion
In this study, we identified that PAPS2 has a Mw of 19.64 kDa and is mainly composed of →6)-α-D-Galp-(1→, →6)-β-D-Glcp-(1→ and →2,6)-α-D-Galp-(1→ residues, and its branches mainly end with β-D-Manp-(1→, which is attached at C2 of →2,6)-α-D-Galp-(1→. PAPS2 ameliorated atherosclerosis by reducing oxidative stress and inflammation, which may be associated with the regulation of the intestinal microflora. PAPS2 is a potential auxiliary anti-atherosclerotic agent that targets lipid metabolism and inflammatory responses.
Author contributions
Fange Kong: methodology, data curation, visualization, methodology, and writing – original draft preparation. Lei Xing: methodology and investigation. Di Wang: conceptualization, writing – review and editing, resources, project administration, and funding acquisition. Changtian Li: conceptualization, resources and funding acquisition. Lanzhou Li: data curation, supervision and methodology. Chunxia Wang: data curation and visualization. Shichao Peng: investigation and methodology.
Conflicts of interest
There are no conflicts to declare.
Acknowledgements
This work was supported by the Special Fund for Modern Agricultural Industrial Technology System [CARS-20 and CARS-20-08B], and the Science and Technology Development Project in Jilin Province, China (20200708068YY and 20210401169YY).
References
- E. Falk, Pathogenesis of Atherosclerosis, J. Am. Coll. Cardiol., 2006, 47, C7–C12 CrossRef CAS PubMed.
- H. C. Stary, A. B. Chandler, S. Glagov, J. R. Guyton, W. Insull, Jr., M. E. Rosenfeld, S. A. Schaffer, C. J. Schwartz, W. D. Wagner and R. W. Wissler, A definition of initial, fatty streak, and intermediate lesions of atherosclerosis. A report from the Committee on Vascular Lesions of the Council on Arteriosclerosis, American Heart Association, Circulation, 1994, 89, 2462–2478 CrossRef CAS PubMed.
- J. Fan and T. Watanabe, Atherosclerosis: Known and unknown, Pathol. Int., 2022, 72, 151–160 CrossRef.
- J. Pedro-Botet, E. Climent and D. Benaiges, Atherosclerosis and inflammation. New therapeutic approaches, Med. Clin., 2020, 155, 256–262 CrossRef CAS.
- C. K. Glass and J. L. Witztum, Atherosclerosis: The Road Ahead, Cell, 2001, 104, 503–516 CrossRef CAS.
- P. Libby, J. E. Buring, L. Badimon, G. K. Hansson, J. Deanfield, M. S. Bittencourt, L. Tokgözoğlu and E. F. Lewis, Atherosclerosis, Nat. Rev. Dis. Primers, 2019, 5, 56 CrossRef.
- M. Khosravi, A. Poursaleh, G. Ghasempour, S. Farhad and M. Najafi, The effects of oxidative stress on the development of atherosclerosis, Biol. Chem., 2019, 400, 711–732 CrossRef CAS.
- I. Tabas, G. García-Cardeña and G. K. Owens, Recent insights into the cellular biology of atherosclerosis, J. Cell Biol., 2015, 209, 13–22 CrossRef CAS PubMed.
- T. Le Roy, E. Lécuyer, B. Chassaing, M. Rhimi, M. Lhomme, S. Boudebbouze, F. Ichou, J. Haro Barceló, T. Huby, M. Guerin, P. Giral, E. Maguin, N. Kapel, P. Gérard, K. Clément and P. Lesnik, The intestinal microbiota regulates host cholesterol homeostasis, BMC Biol., 2019, 17, 94 CrossRef CAS PubMed.
- Z. He, W. Hao, E. Kwek, L. Lei, J. Liu, H. Zhu, K. Y. Ma, Y. Zhao, H. M. Ho, W. S. He and Z. Y. Chen, Fish Oil Is More Potent than Flaxseed Oil in Modulating Gut Microbiota and Reducing Trimethylamine-N-oxide-Exacerbated Atherogenesis, J. Agric. Food Chem., 2019, 67, 13635–13647 CrossRef CAS PubMed.
- S. Zhang, F. Hong, C. Ma and S. Yang, Hepatic Lipid Metabolism Disorder and Atherosclerosis, Endocr., Metab. Immune Disord.: Drug Targets, 2022, 22, 590–600 CrossRef CAS PubMed.
- B. Emini Veseli, P. Perrotta, G. R. A. De Meyer, L. Roth, C. Van der Donckt, W. Martinet and G. R. Y. De Meyer, Animal models of atherosclerosis, Eur. J. Pharmacol., 2017, 816, 3–13 CrossRef CAS PubMed.
- X. X. Zhou, P. J. Gao and B. G. Sun, Pravastatin attenuates interferon-gamma action via modulation of STAT1 to prevent aortic atherosclerosis in apolipoprotein E-knockout mice, Clin. Exp. Pharmacol. Physiol., 2009, 36, 373–379 CrossRef CAS.
- H. Jiang, Z. Ruan, Z. Wang, B. Wu, Z. Gao, X. Ye and X. Lu, Simvastatin reduces atherosclerotic plaques and endothelial inflammatory response in atherosclerosis rats through TGF-β/Smad pathway, Minerva Med., 2020, 111, 504–507 Search PubMed.
- H. R. Underhill, C. Yuan, X. Q. Zhao, L. W. Kraiss, D. L. Parker, T. Saam, B. Chu, N. Takaya, F. Liu, N. L. Polissar, B. Neradilek, J. S. Raichlen, V. A. Cain, J. C. Waterton, W. Hamar and T. S. Hatsukami, Effect of rosuvastatin therapy on carotid plaque morphology and composition in moderately hypercholesterolemic patients: a high-resolution magnetic resonance imaging trial, Am. Heart J., 2008, 155, 584 CrossRef .e581–e588.
- A. Ehlgen, A. Bylock, J. Kreuzer, M. Koslowski, F. Gantner and H. G. Niessen, Clinical imaging in anti-atherosclerosis drug development, Drug Discovery Today, 2015, 20, 1317–1327 CrossRef.
- L. Ren, C. Perera and Y. Hemar, Antitumor activity of mushroom polysaccharides: a review, Food Funct., 2012, 3, 1118–1130 RSC.
- D. Akramiene, A. Kondrotas, J. Didziapetriene and E. Kevelaitis, Effects of beta-glucans on the immune system, Medicina (Kaunas), 2007, 43, 597–606 CrossRef.
- K. Arunachalam, P. S. Sreeja and X. Yang, The Antioxidant Properties of Mushroom Polysaccharides can Potentially Mitigate Oxidative Stress, Beta-Cell Dysfunction and Insulin Resistance, Front. Pharmacol., 2022, 13, 874474 CrossRef CAS.
- P. Kalita, A. B. Ahmed, S. Sen and R. Chakraborty, A comprehensive review on polysaccharides with hypolipidemic activity: Occurrence, chemistry and molecular mechanism, Int. J. Biol. Macromol., 2022, 206, 681–698 CrossRef CAS PubMed.
- Y. Xu, W. Zhu, T. Wang, L. Jin, T. Liu, X. Li, Z. Guan, Z. Jiang, X. Meng, J. Wang and Y. Guo, Low molecule weight fucoidan mitigates atherosclerosis in ApoE (−/−) mouse model through activating multiple signal pathway, Carbohydr. Polym., 2019, 206, 110–120 CrossRef CAS PubMed.
- J. Raman, K. Y. Jang, Y. L. Oh, M. Oh, J. H. Im, H. Lakshmanan and V. Sabaratnam, Cultivation and Nutritional Value of Prominent Pleurotus spp.: An Overview, Mycobiology, 2020, 49, 1–14 CrossRef.
- K. Mori, C. Kobayashi, T. Tomita, S. Inatomi and M. Ikeda, Antiatherosclerotic effect of the edible mushrooms Pleurotus eryngii, (Eringi), Grifola frondosa (Maitake), and Hypsizygus marmoreus (Bunashimeji) in apolipoprotein E-deficient mice, Nutr. Res., 2008, 28, 335–342 CrossRef CAS.
- X. Guo, L. Sun, C. Li, Y. Fu, B. Song and Y. Li, The yield and quality of Pleurotus abieticola grown on nematode-infected Pinus massoniana chips, RSC Adv., 2020, 11, 883–890 RSC.
- M. Pan, F. Kong, L. Xing, L. Yao, Y. Li, Y. Liu, C. Li and L. Li, The Structural Characterization and Immunomodulatory Activity of Polysaccharides from Pleurotus abieticola, Fruiting Bodies, Nutrients, 2022, 14(20), 4410 CrossRef CAS.
- W. Hu, M. Song, C. Wang, Z. Guo, Y. Li and D. Wang, Structural characterization of polysaccharide purified from Hericium erinaceus fermented mycelium and its pharmacological basis for application in Alzheimer's disease: Oxidative stress related calcium homeostasis, Int. J. Biol. Macromol., 2021, 193, 358–369 CrossRef CAS PubMed.
- X. Jiang, J. Hao, Y. Zhu, Z. Liu, L. Li, Y. Zhou, Y. Li, L. Teng and D. Wang, The anti-obesity effects of a water-soluble glucan from Grifola frondosa via the modulation of chronic inflammation, Front. Immunol., 2022, 13, 962341 CrossRef CAS.
- J. Song, Y. Zhang, Y. Zhu, X. Jin, L. Li, C. Wang, Y. Zhou, Y. Li, D. Wang and M. Hu, Structural characterization and anti-osteoporosis effects of polysaccharide purified from Eucommia ulmoides Oliver cortex based on its modulation on bone metabolism, Carbohydr. Polym., 2023, 306, 120601 CrossRef CAS.
- X. Jiang, J. Hao, Z. Liu, X. Ma, Y. Feng, L. Teng, Y. Li and D. Wang, Anti-obesity effects of Grifola frondosa, through the modulation
of lipid metabolism via ceramide in mice fed a high-fat diet, Food Funct., 2021, 12, 6725–6739 RSC.
- Y. Zhang, J. Hao, Z. Liu, Z. Li, L. Teng and D. Wang,
Inonotus hispidus, Protects against Hyperlipidemia by Inhibiting Oxidative Stress and Inflammation through Nrf2/NF-κB Signaling in High Fat Diet Fed Mice, Nutrients, 2022, 14, 3477 CrossRef CAS PubMed.
- M. Dong, H. Liu, T. Cao, L. Li, Z. Sun, Y. Qiu and D. Wang, Huoxiang Zhengqi alleviates azoxymethane/dextran sulfate sodium-induced colitis-associated cancer by regulating Nrf2/NF-κB/NLRP3 signaling, Front. Pharmacol., 2022, 13, 1002269 CrossRef CAS PubMed.
- C. Wang, X. Cai, R. Wang, S. Zhai, Y. Zhang, W. Hu, Y. Zhang and D. Wang, Neuroprotective effects of verbascoside against Alzheimer's disease via the relief of endoplasmic reticulum stress in Aβ-exposed U251 cells and APP/PS1 mice, J. Neuroinflammation, 2020, 17, 309 CrossRef CAS PubMed.
- C. Wang, S. Chen, H. Guo, H. Jiang, H. Liu, H. Fu and D. Wang, Forsythoside A Mitigates Alzheimer's-like Pathology by Inhibiting Ferroptosis-mediated Neuroinflammation via Nrf2/GPX4 Axis Activation, Int. J. Biol. Sci., 2022, 18, 2075–2090 CrossRef CAS PubMed.
- S. Teng, Y. Zhang, X. Jin, Y. Zhu, L. Li, X. Huang, D. Wang and Z. Lin, Structure and hepatoprotective activity of Usp10/NF-κB/Nrf2 pathway-related Morchella esculenta, polysaccharide, Carbohydr. Polym., 2023, 303, 120453 CrossRef CAS.
- F. Huang, R. Zhang, Y. Liu, J. Xiao, D. Su, Y. Yi, G. Wang, Z. Wei and M. Zhang, Characterization and mesenteric lymph node cells-mediated immunomodulatory activity of litchi pulp polysaccharide fractions, Carbohydr. Polym., 2016, 152, 496–503 CrossRef CAS.
- M.-T. Kuang, J.-Y. Li, X.-B. Yang, L. Yang, J.-Y. Xu, S. Yan, Y.-F. Lv, F.-C. Ren, J.-M. Hu and J. Zhou, Structural characterization and hypoglycemic effect via stimulating glucagon-like peptide-1 secretion of two polysaccharides from Dendrobium officinale, Carbohydr. Polym., 2020, 241, 116326 CrossRef CAS PubMed.
- C. F. Ellefsen, C. W. Wold, A. L. Wilkins, F. Rise and A. B. C. Samuelsen, Water-soluble polysaccharides from Pleurotus eryngii, fruiting bodies, their activity and affinity for Toll-like receptor 2 and dectin-1, Carbohydr. Polym., 2021, 264, 117991 CrossRef CAS.
- Y. Cheng, Y. Xie, J.-c. Ge, L. Wang, D.-y. Peng, N.-j. Yu, Y. Zhang, Y.-h. Jiang, J.-p. Luo and W.-d. Chen, Structural characterization and hepatoprotective activity of a galactoglucan from Poria cocos, Carbohydr. Polym., 2021, 263, 117979 CrossRef CAS.
- Y.-X. Wang, T. Zhang, Y. Xin, X.-J. Huang, J.-Y. Yin and S.-P. Nie, Comprehensive evaluation of alkali-extracted polysaccharides from Agrocybe cylindracea: Comparison on structural characterization, Carbohydr. Polym., 2021, 255, 117502 CrossRef CAS.
- Y. Chen, Y. Liu, M. M. R. Sarker, X. Yan, C. Yang, L. Zhao, X. Lv, B. Liu and C. Zhao, Structural characterization and antidiabetic potential of a novel heteropolysaccharide from Grifola frondosa via IRS1/PI3K-JNK signaling pathways, Carbohydr. Polym., 2018, 198, 452–461 CrossRef CAS.
- W. Zhang, B. Hu, M. Han, Y. Guo, Y. Cheng and H. Qian, Purification, structural characterization and neuroprotective effect of a neutral polysaccharide from Sparassis crispa, Int. J. Biol. Macromol., 2022, 201, 389–399 CrossRef CAS PubMed.
- M. Zavadinack, D. de Lima Bellan, J. L. da Rocha Bertage, S. da Silva Milhorini, E. da Silva Trindade, F. F. Simas, G. L. Sassaki, L. M. C. Cordeiro and M. Iacomini, An α-D-galactan and a β-D-glucan from the mushroom Amanita muscaria: Structural characterization and antitumor activity against melanoma, Carbohydr. Polym., 2021, 274, 118647 CrossRef CAS PubMed.
- C. W. Reid, E. Vinogradov, J. Li, H. C. Jarrell, S. M. Logan and J. R. Brisson, Structural characterization of surface glycans from Clostridium difficile, Carbohydr. Res., 2012, 354, 65–73 CrossRef CAS PubMed.
- Q. Zhang, J. Liu, H. Duan, R. Li, W. Peng and C. Wu, Activation of Nrf2/HO-1 signaling: An important molecular mechanism of herbal medicine in the treatment of atherosclerosis via the protection of vascular endothelial cells from oxidative stress, J. Adv. Res., 2021, 34, 43–63 CrossRef CAS.
- Y. Tan, H. Zhang, D. Guo, J. Wang, X. Yuan and Z. Yuan, Adipophilin Involved in Lipopolysaccharide-Induced Inflammation in RAW264.7 Cell via Extracellular Signal-Regulated Kinase 1/2-Peroxisome Proliferator-Activated Receptor Gamma Pathway, DNA Cell Biol., 2017, 36, 1159–1167 CrossRef CAS.
- H. Zhao, B. Deng, D. Li, L. Jia and F. Yang, Enzymatic-extractable polysaccharides from Cordyceps militaris alleviate carbon tetrachloride-induced liver injury via Nrf2/ROS/NF-κB signaling pathway, J. Funct. Foods, 2022, 95, 105152 CrossRef CAS.
- G. Liu, M. Kamilijiang, A. Abuduwaili, D. Zang, N. Abudukelimu, G. Liu, A. Yili and H. A. Aisa, Isolation, structure elucidation, and biological activity of polysaccharides from Saussurea involucrata, Int. J. Biol. Macromol., 2022, 222, 154–166 CrossRef CAS PubMed.
- Y. Zhao, H. Qu, Y. Wang, W. Xiao, Y. Zhang and D. Shi, Small rodent models of atherosclerosis, Biomed. Pharmacother., 2020, 129, 110426 CrossRef CAS.
- A. L. Jonsson and F. Bäckhed, Role of gut microbiota in atherosclerosis, Nat. Rev. Cardiol., 2017, 14, 79–87 CrossRef CAS PubMed.
- N. Alhajri, R. Khursheed, M. T. Ali, T. Abu Izneid, O. Al-Kabbani, M. B. Al-Haidar, F. Al-Hemeiri, M. Alhashmi and F. H. Pottoo, Cardiovascular Health and The Intestinal Microbial Ecosystem: The Impact of Cardiovascular Therapies on The Gut Microbiota, Microorganisms, 2021, 9, 2013 CrossRef CAS PubMed.
- M. Li, W. Chen and T. Zhang, A novel seizure diagnostic model based on kernel density estimation and least squares support vector machine, Biomed. Signal Process. Control, 2018, 41, 233–241 CrossRef.
- D. Zapolska-Downar and M. Naruszewicz, Propionate reduces the cytokine-induced VCAM-1 and ICAM-1 expression by inhibiting nuclear factor-kappa B (NF-kappaB) activation, J. Physiol. Pharmacol., 2009, 60, 123–131 CAS.
- T. Xia, B. Zhang, S. Li, B. Fang, W. Duan, J. Zhang, J. Song and M. Wang, Vinegar extract ameliorates alcohol-induced liver damage associated with the modulation of gut microbiota in mice, Food Funct., 2020, 11, 2898–2909 RSC.
- N. Jiao, S. S. Baker, C. A. Nugent, M. Tsompana, L. Cai, Y. Wang, M. J. Buck, R. J. Genco, R. D. Baker, R. Zhu and L. Zhu, Gut microbiome may contribute to insulin resistance and systemic inflammation in obese rodents: a meta-analysis, Physiol. Genomics, 2018, 50, 244–254 CrossRef CAS PubMed.
- L. Song, Q. Sun, H. Zheng, Y. Zhang, Y. Wang, S. Liu and L. Duan, Roseburia hominis Alleviates Neuroinflammation via Short-Chain Fatty Acids through Histone Deacetylase Inhibition, Mol. Nutr. Food Res., 2022, 66, e2200164 CrossRef.
- K. Kasahara, K. A. Krautkramer, E. Org, K. A. Romano, R. L. Kerby, E. I. Vivas, M. Mehrabian, J. M. Denu, F. Bäckhed, A. J. Lusis and F. E. Rey, Interactions between Roseburia, intestinalis and diet modulate atherogenesis in a murine model, Nat. Microbiol., 2018, 3, 1461–1471 CrossRef CAS PubMed.
- M. J. Villanueva-Millán, P. Pérez-Matute and J. A. Oteo, Gut microbiota: a key player in health and disease. A review focused on obesity, J. Physiol. Biochem., 2015, 71, 509–525 CrossRef PubMed.
- B. G. Talayero and F. M. Sacks, The role of triglycerides in atherosclerosis, Curr. Cardiol. Rep., 2011, 13, 544–552 CrossRef PubMed.
- S. Raposeiras-Roubin, X. Rosselló, B. Oliva, L. Fernández-Friera, J. M. Mendiguren, V. Andrés, H. Bueno, J. Sanz, V. Martínez de Vega, E. Abu-Assi, A. Iñiguez, A. Fernández-Ortiz, B. Ibáñez and V. Fuster, Triglycerides and Residual Atherosclerotic Risk, J. Am. Coll. Cardiol., 2021, 77, 3031–3041 CrossRef CAS.
- S. H. Law, M. L. Chan, G. K. Marathe, F. Parveen, C. H. Chen and L. Y. Ke, An Updated Review of Lysophosphatidylcholine Metabolism in Human Diseases, Int. J. Mol. Sci., 2019, 20, 1149 CrossRef CAS PubMed.
- J. Bismuth, P. Lin, Q. Yao and C. Chen, Ceramide: a common pathway for atherosclerosis?, Atherosclerosis, 2008, 196, 497–504 CrossRef CAS.
- J. Loscalzo, Nitric Oxide Signaling and Atherothrombosis Redux: Evidence From Experiments of Nature and Implications for Therapy, Circulation, 2018, 137, 233–236 CrossRef.
- B. R. Clapp, A. D. Hingorani, R. K. Kharbanda, V. Mohamed-Ali, J. W. Stephens, P. Vallance and R. J. MacAllister, Inflammation-induced endothelial dysfunction involves reduced nitric oxide bioavailability and increased oxidant stress, Cardiovasc. Res., 2004, 64, 172–178 CrossRef CAS PubMed.
- C. Zhao, Y. Zhang, H. Liu, P. Li, H. Zhang and G. Cheng, Fortunellin protects against high fructose-induced diabetic heart injury in mice by suppressing inflammation and oxidative stress via AMPK/Nrf-2 pathway regulation, Biochem. Biophys. Res. Commun., 2017, 490, 552–559 CrossRef CAS PubMed.
- F. Sivandzade, S. Prasad, A. Bhalerao and L. Cucullo, NRF2 and NF-κB interplay in cerebrovascular and neurodegenerative disorders: Molecular mechanisms and possible therapeutic approaches, Redox Biol., 2019, 21, 101059 CrossRef CAS.
- X. Huang, Y. Liu, H. Shen, T. Fu, Y. Guo and S. Qiu, Chlorogenic acid attenuates inflammation in LPS-induced Human gingival fibroblasts via CysLT1R/Nrf2/NLRP3 signaling, Int. Immunopharmacol., 2022, 107, 108706 CrossRef CAS PubMed.
- J. Hettwer, J. Hinterdobler, B. Miritsch, M. A. Deutsch, X. Li, C. Mauersberger, A. Moggio, Q. Braster, H. Gram, A. A. B. Robertson, M. A. Cooper, O. Groß, M. Krane, C. Weber, W. Koenig, O. Soehnlein, N. H. Adamstein, P. Ridker, H. Schunkert, P. Libby, T. Kessler and H. B. Sager, Interleukin-1β suppression dampens inflammatory leucocyte production and uptake in atherosclerosis, Cardiovasc. Res., 2022, 118, 2778–2791 CrossRef CAS PubMed.
- P. M. Ridker, J. G. MacFadyen, T. Thuren and P. Libby, Residual inflammatory risk associated with interleukin-18 and interleukin-6 after successful interleukin-1β inhibition with canakinumab: further rationale for the development of targeted anti-cytokine therapies for the treatment of atherothrombosis, Eur. Heart J., 2020, 41, 2153–2163 CrossRef CAS.
- K. Koushki, S. K. Shahbaz, K. Mashayekhi, M. Sadeghi, Z. D. Zayeri, M. Y. Taba, M. Banach, K. Al-Rasadi, T. P. Johnston and A. Sahebkar, Anti-inflammatory Action of Statins in Cardiovascular Disease: the Role of Inflammasome and Toll-Like Receptor Pathways, Clin. Rev. Allergy Immunol., 2021, 60, 175–199 CrossRef CAS PubMed.
- M. Westerterp, P. Fotakis, M. Ouimet, A. E. Bochem, H. Zhang, M. M. Molusky, W. Wang, S. Abramowicz, S. la Bastide-van Gemert, N. Wang, C. L. Welch, M. P. Reilly, E. S. Stroes, K. J. Moore and A. R. Tall, Cholesterol Efflux Pathways Suppress Inflammasome Activation, NETosis, and Atherogenesis, Circulation, 2018, 138, 898–912 CrossRef CAS PubMed.
- W. Zeng, D. Wu, Y. Sun, Y. Suo, Q. Yu, M. Zeng, Q. Gao, B. Yu, X. Jiang and Y. Wang, The selective NLRP3 inhibitor MCC950 hinders atherosclerosis development by attenuating inflammation and pyroptosis in macrophages, Sci. Rep., 2021, 11, 19305 CrossRef CAS.
- M. Ortego, C. Bustos, M. A. Hernández-Presa, J. Tuñón, C. Díaz, G. Hernández and J. Egido, Atorvastatin reduces NF-kappaB activation and chemokine expression in vascular smooth muscle cells and mononuclear cells, Atherosclerosis, 1999, 147, 253–261 CrossRef CAS.
- D. Z. Shen, S. L. Xin, C. Chen and T. Liu, Effect of atorvastatin on expression of TLR4 and NF-κB p65 in atherosclerotic rabbits, Asian Pac. J. Trop. Med., 2013, 6, 493–496 CrossRef CAS.
|
This journal is © The Royal Society of Chemistry 2024 |
Click here to see how this site uses Cookies. View our privacy policy here.