DOI:
10.1039/D3FO02826G
(Paper)
Food Funct., 2024,
15, 183-195
Chemopreventive effects of α-tocopherol and its long-chain metabolites α-13′-hydroxy- and α-13′-carboxychromanol in LT97 colon adenoma cells
Received
12th July 2023
, Accepted 25th November 2023
First published on 27th November 2023
Abstract
Anticancer effects of vitamin E (tocopherols) have been studied extensively. While in vitro and animal studies showed promising results regarding anticancer effects of tocopherols, human intervention studies failed to reproduce these results. In vivo, α-tocopherol (α-TOH) is metabolized to the long-chain metabolites (LCM) 13′-hydroxychromanol (α-13′-OH) and 13′-carboxychromanol (α-13′-COOH), which likely reach the large intestine. The LCM showed antiproliferative effects in different colon cancer cell lines, but the exact mechanism of action remains unclear. To further clarify the chemopreventive action of the LCM, premalignant LT97 colon adenoma cells were treated with α-TOH, α-13′-OH and α-13′-COOH to study their impact on growth, apoptosis, antigenotoxicity, and ROS-scavenging capacity as well as expression of selected genes involved in detoxification and the cell cycle. Growth inhibitory potential was observed for α-13′-OH (IC50: 37.4 μM) and α-13′-COOH (IC50: 5.8 μM) but not for α-TOH in the tested concentrations. Levels of caspase-3 activity and expression of genes regulating the cell cycle and detoxification remained unchanged. However, α-TOH, α-13′-OH and α-13′-COOH exhibited antigenotoxic and partly ROS-scavenging capacity. The results indicate that the LCM exert chemopreventive effects via ROS-scavenging capacity, the protection against DNA damage and the induction of cell death via caspase-independent mechanisms in premalignant colon cells.
1. Introduction
Since the discovery of vitamin E (tocopherols) in 1922 as an essential factor for the fertility of rats,1 there is still a lot of research interest regarding the effects of vitamin E on human health. Vitamin E is a fat-soluble vitamin, which is widely considered to consist of four isomers (α, β, γ, δ) of each tocopherol and tocotrienol.2,3 Vitamin E exhibits antioxidant and ROS- (reactive oxygen species) scavenging activity, which is mediated by its hydroxy chromanol structure.2 Of all vitamin E congeners, α-tocopherol (α-TOH) is the most active antioxidant.2,4 It is important to note that studies also indicated that vitamin E acts via non-antioxidant mechanisms such as modulating signaling pathways by affecting gene expression or enzyme activities.5,6
In this regard, several studies have investigated potential health-related effects and particularly, in vitro-based studies showed promising results regarding different health-relevant endpoints such as cardiovascular disease and cancer.3,4,7,8 Results from epidemiological studies also showed an association of a low vitamin E status and an enhanced risk for cancer indicating that vitamin E might be important for modulating cancer incidence. However, results from human intervention studies provided inconsistent or contradictory results, as reviewed.9,10 For example, in the selenium and vitamin E cancer prevention trial (SELECT), no cancer preventive effects of vitamin E intake were observed. In contrast, the supplementation with vitamin E (400 IU per day) in adequately supplied men was even associated with an insignificant increase in the risk for prostate cancer.11 The still unclear data situation about the potential health effects of vitamin E and the mechanisms involved make further investigations necessary. As reviewed,12 clear anticancer effects of vitamin E have been demonstrated in vitro in several cancer cell types such as human prostate cancer cells LNCaP and PC-3 for e.g. γ-TOH, whereas α-TOH had no impact. Furthermore, the growth of healthy prostate cells was not inhibited.13 Similar effects were observed in HCT-116 colon cancer14 and breast cancer cells15 and mechanistic studies indicated that anticancer effects of γ-TOH are mediated by accumulation of certain ceramides and sphingolipids12–15 and by inhibition of eicosanoid synthesis by blocking cyclooxygenase (COX) and lipoxygenase (LOX).12 Chemopreventive effects of γ-TOH have also been investigated in several cancer models (e.g. prostate, colon, esophageal and breast cancer models) in animal studies.12
The different results obtained from in vitro as well as in vivo studies in chemically induced cancer models and in vivo studies in humans may indicate that mechanisms of vitamin E in vitro might differ from its mode of action in vivo. In vivo, all forms of vitamin E are metabolized in the liver resulting in bioactive intermediates such as the long-chain metabolites (LCM) as reviewed.3,4 Consequently, recent research not only focused on vitamin E but also on its metabolites as bioactive compounds with potential health-related effects since the LCM are proposed to mediate the vitamin function of vitamin E (α-TOH) at least in part.4,8 Vitamin E metabolism comprises intestinal absorption, vascular transport, and hepatic sorting by transport proteins such as the α-tocopherol transfer protein (α-TTP). α-TTP preferentially binds to α-TOH so that a discrimination of the different vitamin E congeners in favor of α-TOH occurs in the liver. Here, α-TOH is metabolized via ω-hydroxylation and ω-oxidation to the LCM 13′-hydroxychromanol (α-13′-OH) and 13′-carboxychromanol (α-13′-COOH), respectively. These LCM are mainly secreted via bile acids into the intestine and excreted via feces, while the short-chain metabolites such as carboxyethyl-hydroxychromanol (CEHC) are eliminated via urine.4,6,16 Thus, the LCM of tocopherol could exert bioactive and maybe chemopreventive effects on intestinal epithelial cells. Anticancer effects of tocopherol and its LCM, particularly α-13′-OH and α-13′-COOH, have been demonstrated in in vitro studies using different cancer cell lines such as HepG2 cells,17 HT29 cells18 or Caco-2 cells19 as reviewed by Wallert et al.3 Nevertheless, the antiproliferative actions of α-TOH metabolites vary in different types of cells and the exact mechanisms remain to be examined. Previous in vitro studies mainly focused on investigating chemopreventive effects of vitamin E congeners and their metabolites in colon cancer cells. To provide new and further mechanistic insights into the chemopreventive potential of α-TOH and its LCM α-13′-OH and α-13′-COOH, the present study aims at investigating antiproliferative, antigenotoxic and antioxidant effects using premalignant LT97 colon adenoma cells as reflection of an early stage of colon carcinogenesis. Therefore, this study contributes to the understanding of the role of vitamin E and its LCM as potential chemopreventive agents in early colon cancer development and prevention.
2. Materials and methods
2.1 α-TOH and LCM
α-TOH was purchased from Carl Roth GmbH & Co. KG (Karlsruhe, Germany). The LCM α-13′-OH and α-13′-COOH were semi-synthesized from garcinoic acid (δ-tocotrienoloic acid) which was isolated from the African bitter nut Garcinia kola es described.17,20
2.2 Cell culture
LT97 colon adenoma cells (a kind gift from Professor B. Marian, Institute for Cancer Research, University of Vienna, Austria) were used as model of an early and premalignant stage of tumour development. The cell line was prepared from colon microadenoma of a patient suffering from hereditary familiar polyposis coli21 and culture conditions were described in detail previously.22 The cells were regularly checked by mycoplasma tests (MycoAlert mycoplasma detection kit, Lonza, Switzerland) to exclude contamination.
2.3 Growth inhibition – DAPI assay
Growth inhibitory effects were analyzed using the DAPI (4′,6-diamidino-2-phenylindol) assay as described previously.22 In brief, LT97 cells were seeded into 96-well plates, grown to a confluence of 30–40% and treated with α-TOH (10 μM, 25 μM, 50 μM, 100 μM), α-13′-OH (10 μM, 25 μM, 50 μM, 100 μM) or α-13′-COOH (0.5 μM, 1 μM, 2.5 μM, 5 μM and 10 μM) for either 24 h or 48 h. After treatment, the incubation medium was removed, and cells were fixed with 100 μl methanol (Carl Roth GmbH & Co. KG, Karlsruhe, Germany). After 5 min, methanol was removed and evaporated. Next, cells were stained with 100 μl of DAPI solution (20 μM in PBS, phosphate buffered saline, 30 min, 37 °C, Sigma-Aldrich Inc., St Louis, MO, USA). The fluorescence was recorded at Ex/Em of λ = 360/465 nm (SpectraFluor Plus, Tecan Germany, Crailsheim, Germany) and relative cell numbers were calculated from the blank-corrected results based on the vehicle control (DMSO, Carl Roth GmbH & Co. KG, Karlsruhe, Germany), which was set to 100%. IC50 and IC25 values were calculated via nonlinear regression/one phase exponential decay (GraphPad Prism®, GraphPad Software, San Diego, CA, USA). IC50 and IC25 values for α-TOH, α-13′-OH and α-13′-COOH are presented in Table 1. These were used to estimate sub-toxic concentrations of α-TOH (100 μM), α-13′-OH (10 μM) and α-13′-COOH (5 μM) which were used for further cell culture experiments.
Table 1 IC50 and IC25 values (μM) of α-tocopherol and long-chain metabolites in LT97 cells
Treatment |
24 h |
48 h |
IC50 |
IC25 |
IC50 |
IC25 |
α-TOH: α-tocopherol, α-13′-OH: α-13′-hydroxychromanol, α-13′-COOH: α-13′-carboxychromanol, IC: inhibitory concentration, n. d.: not detectable. |
α-TOH |
n. d. |
n. d. |
n. d. |
97.8 |
α-13′-OH |
37.4 |
13.4 |
12.4 |
4.8 |
α-13′-COOH |
5.8 |
2.5 |
7.5 |
3.7 |
2.4 Apoptosis – measurement of caspase-3 activity
The activity of caspase-3 as a marker of apoptosis was determined as described in detail previously.23 In brief, LT97 cells were grown in 6-well plates to a confluence of 50–60% and treated with α-TOH (100 μM), α-13′-OH (10 μM) or α-13′-COOH (5 μM) or controls (medium and DMSO as negative and vehicle control, respectively) for 24 h or 48 h. Cells treated with sodium-butyrate (4 mM, Merck KGaA, Darmstadt, Germany) served as positive control. After treatment, cell lysis and measurement of caspase-3 activity in cell lysates was performed as described23 using 50 nM caspase-3 inhibitor (Ac-DEVD-CHO, Enzo Life Science, Lörrach, Germany) and 25 μM caspase-3 substrate (Ac-DEVD-AMC, Enzo Life Science, Lörrach, Germany). Fluorescence intensity was measured at Ex/Em λ = 380/465 nm (SpectraFluor Plus, Tecan Germany, Crailsheim, Germany) and modulation of caspase activity was calculated relative to the vehicle control (DMSO), which was set to 1.
2.5 Genotoxicity and antigenotoxicity – Comet assay
To examine genotoxic and antigenotoxic effects of α-TOH and the LCM in LT97 cells the alkaline modification of the Comet assay was used as described earlier24 with minor modifications. To determine short-term genotoxic and antigenotoxic effects, LT97 cells were harvested from a 75 cm2 cell culture flasks at a confluence of 80–90% as described.22 The cell number was adjusted to 0.2 × 106 and cells were treated with either 100 μM α-TOH, 10 μM α-13′-OH or 5 μM α-13′-COOH in PBS (pH 7.4) for 1 h at 37 °C to determine genotoxic effects. Antigenotoxic effects were examined by treatment of cells with α-TOH or LCM for 45 min and 15 min co-incubation with 75 μM H2O2 (Merck KGaA, Darmstadt, Germany) at 37 °C.
Rather long-term genotoxic effects were examined in LT97 cells grown in 6-well plates (50–60% confluence) after treatment with α-TOH (100 μM), α-13′-OH (10 μM) or α-13′-COOH (5 μM) in cell culture medium for 24 h at 37 °C. Cells were washed with PBS and, after harvesting, the cell number was adjusted to 0.2 × 106. For examination of antigenotoxic effects, 0.2 × 106 cells were treated with 75 μM H2O2 for 15 min at 37 °C and washed with PBS. In all conditions, cell pellets were obtained by centrifugation (425g, 5 min, 4 °C) and were stored on ice until they were mixed with 45 μl 0.7% low-melting agarose (Biozym, Hessisch Oldendorf, Germany) and spread onto pre-coated (0.5% normal melting agarose, Biozym, Hessisch Oldendorf, Germany) microscopic slides. Cells treated with PBS and H2O2 (75 μM, 15 min) served as negative and positive control, respectively. A detailed description of the procedure of the alkaline modification of the Comet assay and the detection of DNA damage (% TI, tail intensity; means of 100 counted cells) is presented in Glei et al.24,25
2.6 ROS formation – DCF assay
ROS formation as a marker for oxidative stress was measured using the DCF assay as described by Pelka et al.26 with slight modifications. In brief, LT97 cells were seeded into black clear-bottom 96-well plates and grown to a confluence of 60% as described.22 Cells were washed twice with PBS++ (containing 0.9 mM CaCl2·2H2O and 0.5 mM MgCl2·6H2O) and incubated with 100 μl of a 20 μM DCFH-DA solution (2′,7′-dichlorofluorescin-diacetate, in PBS++, Sigma-Aldrich, St Louis, MO, USA) for 30 min at 37 °C. The DCFH-DA solution was removed, and cells were washed again twice with PBS++. Subsequently, cells were treated with α-TOH (25 μM, 50 μM, 100 μM), α-13′-OH or α-13′-COOH (2,5 μM, 5 μM and 10 μM) as well as controls (2 mM H2O2 served as positive control, and medium as well as DMSO served as negative and vehicle control, respectively) for up to 24 h at 37 °C. In addition, cells were co-incubated with α-TOH and the LCM together with 2 mM H2O2 to detect their ROS-scavenging capacity. The fluorescence intensity was measured at Ex/Em of λ = 485/535 nm (Synergy 2, Biotek Instruments GmbH, Bad Friedrichshall, Germany) in intervals of 30 min up to 3 h and after 6 h and 24 h.
2.7 Gene expression – RT-qPCR
The expression of the antioxidant relevant genes catalase, SOD2 (superoxide dismutase 2), GSTP1 (glutathione S-transferase P1), GPX1 (glutathione peroxidase 1) and of the cell cycle relevant genes p21 and cyclin D2 were analyzed in LT97 cells after treatment with 100 μM α-TOH, 10 μM α-13′-OH or 5 μM α-13′-COOH for 24 h.
RNA isolation and cDNA synthesis.
Sample preparation of LT97 cells for RNA isolation was conducted after the removal of cell culture supernatants. Total RNA was extracted using the RNeasy Mini kit (Qiagen, Hilden, Germany) as described.27 Genomic DNA was removed by on-column DNase I digestion (Qiagen, Hilden, Germany). cDNA synthesis was carried out using revert aid first strand cDNA synthesis kit (ThermoFisher Scientific, Karlsruhe, Germany) with 5 μg total RNA, 500 ng μl−1 oligo(dT) primer (ThermoFisher Scientific, Karlsruhe, Germany), 20 units ribonuclease inhibitor (ThermoFisher Scientific, Karlsruhe, Germany), 1 mM deoxyribonucleoside triphosphates (dNTPs, ThermoFisher Scientific, Karlsruhe, Germany) and 200 units M-MuLV reverse transcriptase RevertAid (ThermoFisher Scientific, Karlsruhe, Germany) as described.28
Quantitative real-time reverse transcription PCR.
Quantitative real-time reverse transcription PCR (RT-qPCR) was performed using maxima SYBR green qPCR master mix (ThermoFisher Scientific, Karlsruhe, Germany) as described.29 Each sample was measured in duplicates on a LightCycler 480 instrument (Roche Diagnostics, Mannheim, Germany) using the Light Cycler software version 1.5.0.39. Cycling parameters included an initial incubation for 15 min at 95 °C, a 40-cycle PCR that consisted of denaturation at 94 °C for 15 s, and a combined annealing and extension phase at 60 °C for 30 s. The relative mRNA expression of the target genes was normalized to the expression of the reference gene GAPDH (glyceraldehyde-3-phosphate dehydrogenase) based on the equation of Pfaffl et al.30 PCR efficiencies were tested using cDNA dilutions. All primers were purchased from Eurofins Genomics (Ebersberg, Germany), except primers for GAPDH which were purchased from Invitrogen (ThermoFisher Scientific, Karlsruhe, Germany). The primer sequences are provided in Table 2.
Table 2 Primer sequences
Genes |
Sequence |
GAPDH: glyceraldehyde-3-phosphate dehydrogenase, GPX1: glutathione peroxidase 1, GSTP1: glutathione S-transferase P1, p21: cyclin-dependent kinase inhibitor 1A, SOD2: superoxide dismutase 2.
|
Catalase
|
Forward 5′-TGG ACA AGT ACA ATG CTG AG-3′ |
Reverse 5′-TTA CAC GGA TGA ACG CTA AG-3′ |
SOD2
|
Forward 5′-GCC CTG GAA CCT CAC ATC AAC-3′ |
Reverse 5′-CAA CGC CTC CTG GTA CTT CTC-3′ |
GSTP1
|
Forward 5′-TTG GAC TGG TAC AGG GTG AGG TC-3′ |
Reverse 5′-CTG CGC ATG CTG CTG GCA GAT C-3′ |
GPX1
|
Forward 5′-GAC TAC ACC CAG ATG AAC GA-3′ |
Reverse 5′-ACG TAC TTG AGG GAA TTC AG-3′ |
p21
|
Forward 5′-CAC TGT CTT GTA CCC TTG TG-3′ |
Reverse 5′-CTT CCT CTT GGA GAA GAT CAG-3′ |
Cyclin D2
|
Forward 5′-CCA CCG ACT TTA AGT TTG CC-3′ |
Reverse 5′-CTT TGA GAC AAT CCA CGT CTG-3′ |
GAPDH
|
Forward 5′-CAA CAG CGA CAC CCA CTC CT-3′ |
Reverse 5′-CAC CCT GTT GCT GTA GCC AAA-3′ |
2.8 Statistical analyses
Unless stated otherwise, means and standard deviations of at least three independent experiments were calculated and statistical differences were analyzed by one-way ANOVA and Bonferroni post hoc test using GraphPad Prism® software version 5 (GraphPad, San Diego, CA, USA) and SPSS® Statistics software version 26 (IBM Corporation, Armonk, NY, USA).
3. Results
3.1 Growth inhibition
Treatment of LT97 cells with 10 μM, 25 μM, 50 μM or 100 μM α-TOH for 24 h and 48 h had no significant impact on relative cell numbers (Fig. 1A and B). Only a marginal reduction of cell growth down to 87.2 ± 7.1% and 76.1 ± 14.1% was observed after 24 h and 48 h treatment, respectively, with the highest α-TOH concentration of 100 μM. In comparison, treatment of LT97 cells with increasing concentrations of the LCM α-13′-OH (10–100 μM) led to a dose-dependent decrease of relative cell numbers and a significant reduction of cell growth compared to cells treated with the vehicle control (Fig. 1C and D). Incubation of cells with 100 μM α-13′-OH led to a reduction of cell growth down to 29.4 ± 5.5% and 12.1 ± 6.3% after 24 h and 48 h, respectively. Treatment of LT97 cells with the LCM α-13′-COOH (0.5–10 μM) also resulted in a significant and dose-dependent reduction of relative cell numbers down to 27.6 ± 18.0% and 31.0 ± 11.3% after 24 h and 48 h, respectively (Fig. 1E and F).
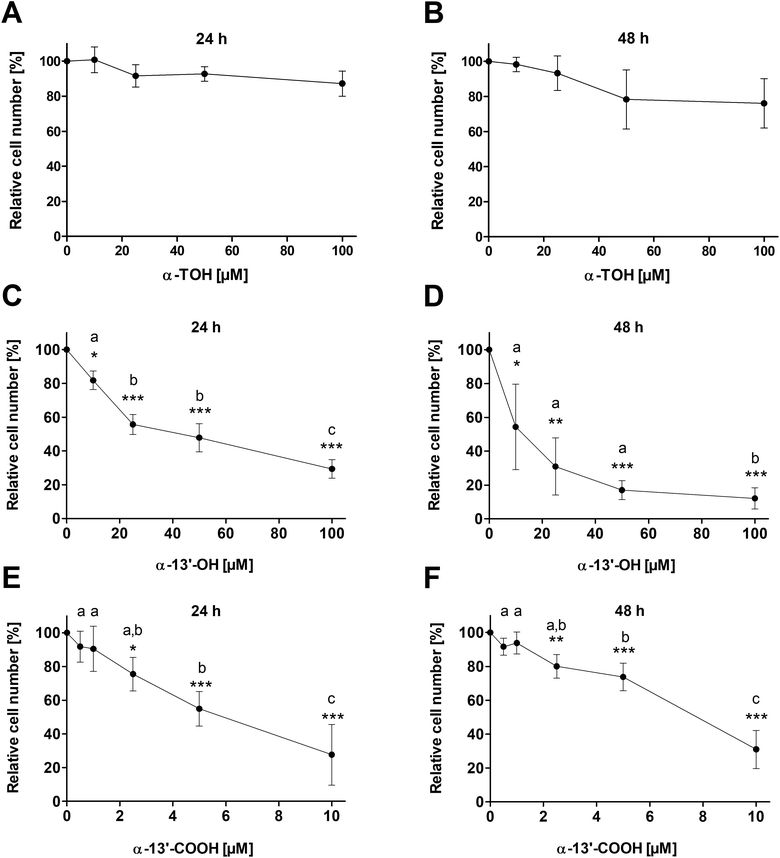 |
| Fig. 1 Relative cell number [%] of LT97 colon adenoma cells after treatment with different concentrations of α-TOH, α-13′-OH (10 μM, 25 μM, 50 μM, 100 μM) or α-13′-COOH (0.5 μM, 1 μM, 2.5 μM, 5 μM and 10 μM) for 24 h (A, C and E) and 48 h (B, D and F). Relative cell numbers were calculated based on the vehicle control DMSO which was set 100% (mean ± SD, n = 3). Significant differences compared to cells treated with the vehicle control (* p < 0.05, ** p < 0.01, *** p < 0.001) and significant differences between different groups (a,b,cp < 0.05, different letters represent statistically different results) were obtained by one-way ANOVA and Bonferroni's multiple comparison test. α-TOH: α-tocopherol, α-13′-OH: α-13′-hydroxychromanol, α-13′-COOH: α-13′-carboxychromanol. | |
3.2 Apoptosis
Caspase-3 activity was determined as an important marker of advanced apoptotic effects. Treatment of LT97 cells with 100 μM α-TOH, 10 μM α-13′-OH or 5 μM α-13′-COOH for 24 h had no clear impact on caspase-3 activity (Fig. 2A). In contrast, the positive control butyrate (4 mM) induced a significant increase of relative caspase-3 activity of 14.1 ± 1.6-fold compared to the respective medium control. Butyrate also significantly enhanced caspase-3 activity after 48 h of incubation to a level of 14.6 ± 7.8-fold. In contrast, treatment of LT97 cells with α-TOH and the LCM resulted in a decrease of relative caspase-3 activity compared to the respective DMSO vehicle control after 48 h.
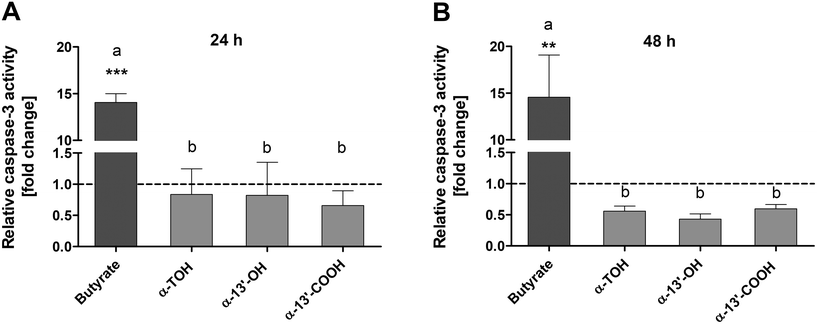 |
| Fig. 2 Relative caspase-3 activity [fold change] in LT97 cells after treatment with 100 μM α-TOH, 10 μM α-13′-OH, 5 μM α-13′-COOH or the positive control (4 mM butyrate) for 24 h (A) and 48 h (B). Fold changes were calculated based on the vehicle control (DMSO, dashed line), which was set to 1 (mean + SD, n = 3). Significant differences between cells treated with butyrate and the vehicle control (** p < 0.01, *** p < 0.001) and significant differences between different groups (a,bp < 0.05, different letters represent statistically different results) were obtained by one-way ANOVA and Bonferroni's multiple comparison test. α-TOH: α-tocopherol, α-13′-OH: α-13′-hydroxychromanol, α-13′-COOH: α-13′-carboxychromanol. | |
3.3 Genotoxic and antigenotoxic effects
Genotoxic and antigenotoxic effects of α-TOH, α-13′-OH and α-13′-COOH were measured in LT97 cells after short-term (1 h) and long-term (24 h) treatment. Incubation of LT97 with α-TOH, α-13′-OH or α-13′-COOH for 1 h resulted in tail intensities of 1.9 ± 0.4%, 3.6 ± 0.7% and 2.4 ± 0.9%, respectively (Fig. 3A). After 24 h, tail intensities of 0.9 ± 0.2%, 0.8 ± 0.4% and 1.4 ± 0.2% were measured for α-TOH, α-13′-OH and α-13′-COOH, respectively (Fig. 3B). These tail intensities were comparable to that observed after treatment with the respective negative controls (Fig. 3A and B). In comparison, treatment with 75 μM H2O2 resulted in significant higher tail intensities (1 h: 20.4 ± 2.3%, 24 h: 24.5 ± 3.2%) compared to the respective negative controls. Antigenotoxic effects in LT97 cells were determined after co-incubation with 75 μM H2O2 and α-TOH or LCM. Tail intensities measured after short-term treatment (45 min) with α-13′-OH (22.2 ± 3.1%) and α-13′-COOH (21.9 ± 2.1%) and subsequent co-treatment with 75 μM H2O2 (15 min) were significantly lower than the tail intensity induced by the positive control (75 μM H2O2, 33.4 ± 1.2%) (Fig. 3C). Incubation of LT97 cells with α-TOH also resulted in a reduced tail intensity (27.8 ± 2.3%, p = 0.083) compared to the positive control (Fig. 3C). Treatment of cells for 24 h with α-TOH (17.6 ± 1.6%), α-13′-OH (14.8 ± 1.5%) and α-13′-COOH (14.1 ± 2.4%) and additional co-treatment with 75 μM H2O2 for 15 min also resulted in significantly lower tail intensities than treatment with the positive control alone (24.5 ± 3.2%) (Fig. 3D).
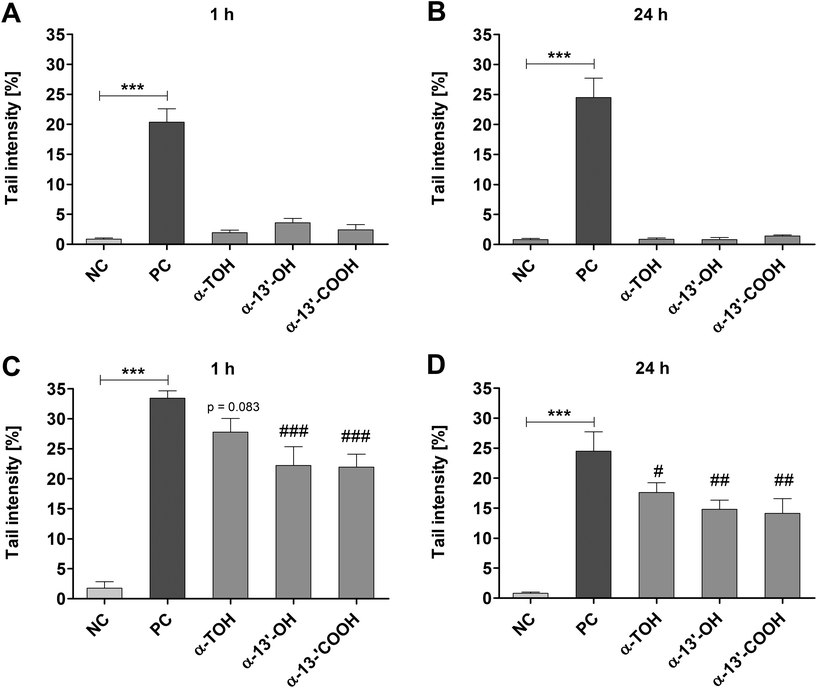 |
| Fig. 3 Genotoxic (A and B) and antigenotoxic effects (C and D) of α-TOH and its long-chain metabolites expressed as tail intensities [%] measured in nuclei of LT97 cells after treatment with 100 μM α-TOH, 10 μM α-13′-OH, 5 μM α-13′-COOH or controls (NC: negative control = PBS/DMSO or cell culture medium/DMSO, PC: positive control = 75 μM H2O2) for 1 h (A and C) and 24 h (B and D) (mean + SD, n = 3). Antigenotoxic effects were detected in cells after co-incubation with α-TOH as well as long-chain metabolites together with 75 μM H2O2 (for 15 min) after 1 h and 24 h (C and D). Significant differences between NC and PC (*** p < 0.001) and between cells treated with α-TOH as well as long-chain metabolites and PC (#p < 0.05, ##p < 0.01, ###p < 0.001) were obtained by one-way ANOVA and Bonferroni's multiple comparison test. α-TOH: α-tocopherol, α-13′-OH: α-13′-hydroxychromanol, α-13′-COOH: α-13′-carboxychromanol. | |
3.4 ROS formation
The induction of ROS in LT97 cells by α-TOH and its LCM α-13′-OH or α-13′-COOH as well as the potential to reduce ROS was checked via DCF assay. No induction of relative ROS levels was observed in LT97 cells after treatment with α-TOH, α-13′-OH and α-13′-COOH (Fig. 4A, C and E). In contrast, a significant reduction of H2O2-induced ROS levels was observed after co-treatment of cells with 5 μM (5.3 ± 0.6-fold) and 10 μM α-13′-COOH (5.3 ± 0.8-fold) and 2 mM H2O2 in comparison to the H2O2 co-treated DMSO control (7.2 ± 1.2-fold) (Fig. 4F). In comparison, α-TOH and α-13′-OH treatment had no effect on H2O2-induced ROS levels in LT97 cells (Fig. 4B and D).
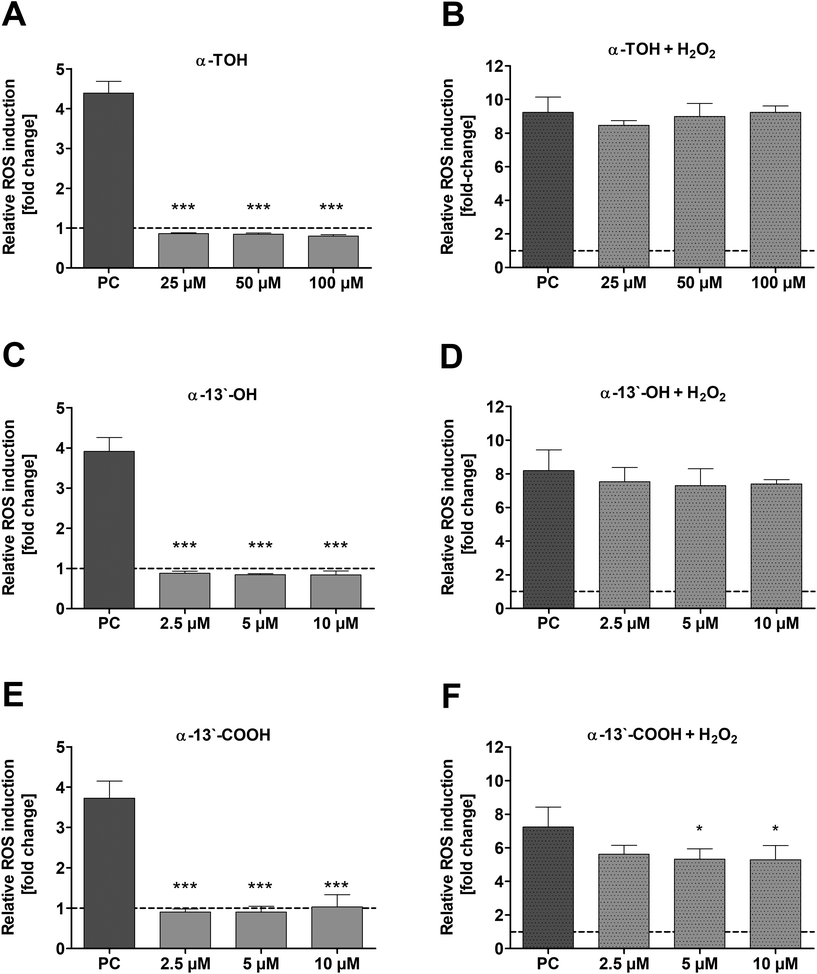 |
| Fig. 4 Relative levels of ROS [fold change] in LT97 cells after treatment with different concentrations of α-TOH (25 μM, 50 μM, 100 μM), α-13′-OH or α-13′-COOH (2.5 μM, 5 μM and 10 μM) for 24 h as well as PC (positive control: 2 mM H2O2) (A, C and E) and co-incubation with 2 mM H2O2 (B, D and F). Relative ROS induction was calculated based on the control (cell culture medium/DMSO), which was set to 1 (dashed line) (mean + SD, n = 4). Significant differences between cells treated with α-TOH as well as long-chain metabolites and PC (* p < 0.05, *** p < 0.001) were obtained by one-way ANOVA and Bonferroni's multiple comparison test. α-TOH: α-tocopherol, α-13′-OH: α-13′-hydroxychromanol, α-13′-COOH: α-13′-carboxychromanol. | |
3.5 mRNA expression
The expression of genes involved in oxidative stress response such as catalase, SOD2, GSTP1 and GPX1 in LT97 colon adenoma cells was not significantly altered by α-TOH, α-13′-OH or α-13′-COOH (Fig. 5A–D) at the investigated time points and concentrations. Only a marginal increase in GSTP1 mRNA expression was observed after treatment of cells with 100 μM α-TOH (1.4 ± 0.8-fold, Fig. 5C). Furthermore, the mRNA expression of the cell cycle relevant genes p21 and cyclin D2 in LT97 cells was not affected by treatment with α-TOH and the LCM (Fig. 5E and F). For p21 a marginal increase was observed after treatment with α-TOH (1.5 ± 0.7-fold, Fig. 5E), while this treatment resulted in an insignificant slight decrease in cyclin D2 mRNA level (0.7 ± 0.5-fold) (Fig. 5F).
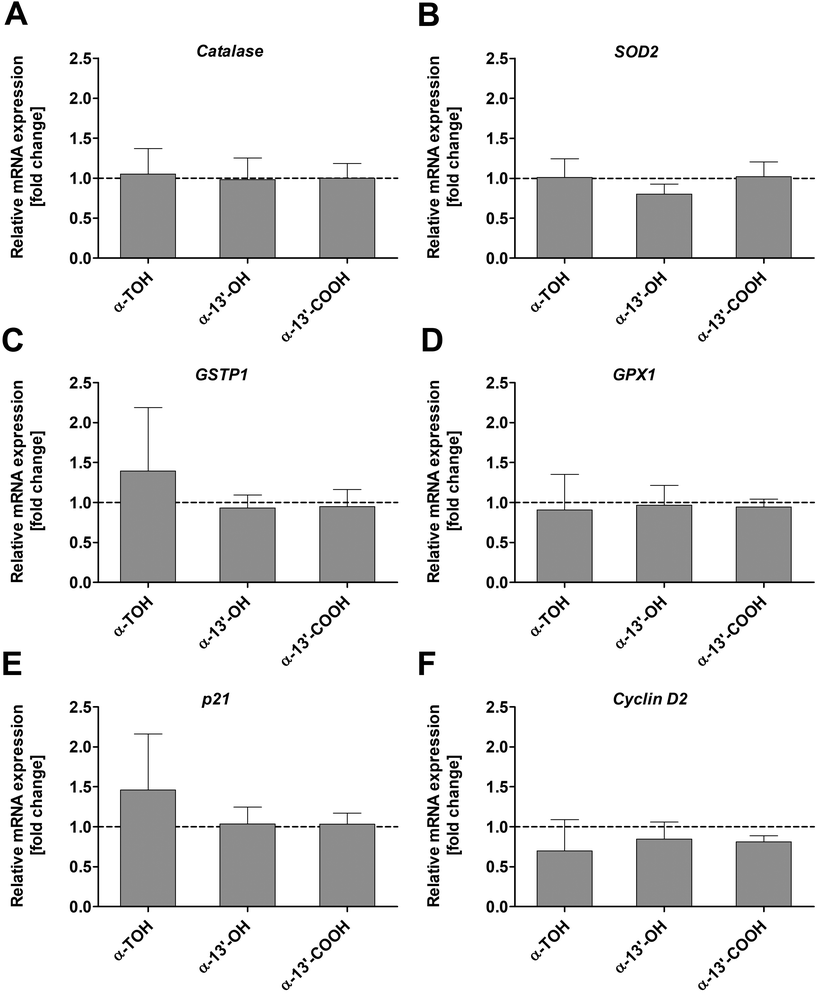 |
| Fig. 5 Relative mRNA expression [fold change] of catalase (A), SOD2 (B), GSTP1 (C), GPX1 (D), p21 (E), and cyclin D2 (F) in LT97 cells after treatment with 100 μM α-TOH, 10 μM α-13′-OH, or 5 μM α-13′-COOH for 24 h. Fold changes were calculated based on the vehicle control (DMSO, dashed line), which was set to 1 (mean + SD, n = 4). Results were checked for significant differences using one-way ANOVA and Bonferroni's multiple comparison test. α-TOH: α-tocopherol, α-13′-OH: α-13′-hydroxychromanol, α-13′-COOH: α-13′-carboxychromanol, GPX1: glutathione peroxidase 1, GSTP1: glutathione S-transferase P1, p21: cyclin-dependent kinase inhibitor 1, SOD2: superoxide dismutase 2. | |
4. Discussion
Particularly regarding potential anticancer effects of vitamin E there is a discrepancy between results obtained by in vitro and in vivo studies as reviewed.3,4,9,10 Furthermore, there is still a lack of understanding the mechanistic behind potential beneficial effects of vitamin E intake since human intervention studies failed to reproduce in vitro effects or even indicate adverse health effects such as an increased overall mortality risk.31 These discrepancies might be explained by the metabolism of vitamin E in vivo, and it is proposed that the LCM mediate at least in part the function of vitamin E (α-TOH).4,8 Therefore, studies investigating also the metabolized forms of vitamin E are essential to further elucidating mechanistic aspects. Some studies already showed anticancer effects of the LCM in animals,32,33 and in vitro in liver17 and colon cancer cells,14 but the exact mechanism remains poorly understood. For example, anticancer effects of vitamin E and its metabolites have been demonstrated in vitro in several cancer cell types. Chemopreventive effects of γ-TOH have also been investigated in several animal cancer models (e.g. prostate, colon, esophageal and breast cancer models).12 Mechanistically, anticancer effects of γ-TOH and metabolites are mediated by accumulation of certain ceramides and sphingolipids12–15 and by inhibition of eicosanoid synthesis by blocking COX and LOX.12 Furthermore, in the different animal models, other mechanisms of anticancer potential were revealed as e.g. inhibition of NF-κB activation and CXCR3-mediated inflammation in a murine esophageal cancer model12,34 or e.g. by increasing p21, p27 and PPAR-γ in female Sprague Dawley rats as mammary tumor models.12,35 Mostly, α-TOH showed no or little anticancer effects,12 whereas studies indicate, that LCM of α-TOH have an anticancer potential. Furthermore, Chen et al. demonstrated inhibitory activities of tocopherols on NF-κB p65 and p-STAT3 pro-inflammatory mediators in a murine model of colon cancer.36 In this study, the authors also showed that γ-TOH reduced DNA damage and increased levels of cleaved caspase-3 and they discussed that the anticancer effects of tocopherols may be mainly mediated by protection against DNA damage at the early stage of colon carcinogenesis.
Therefore, the aim of the present study was to focus unraveling anticancer effects of α-TOH and its LCM in premalignant colon adenoma cells as a model of early carcinogenesis to provide further insights into the chemopreventive potential of vitamin E metabolites regarding colon cancer development. Since mechanistic aspects of anticancer activity of vitamin E in general are reviewed and discussed in detail elsewhere,8,12 the present discussion focused on the comparison between effects of particularly LCM on colon adenoma cells and results obtained by other in other colon cancer cell models.
The LCM α-13′-OH and α-13′-COOH significantly inhibited the growth of LT97 colon adenoma cells in a dose-dependent manner. Here, α-13′-COOH (IC50: 5.8 μM) was more effective in reducing relative cell numbers than α-13′-OH (IC50: 37.4 μM). In contrast, the metabolic precursor α-TOH had no effect. These results are in line with earlier studies investigating anticancer effects of vitamin E metabolites in different types of cancer cells. In general, in vitro and in vivo studies demonstrated that α-TOH exhibits no or only weak anticancer effects whereas other congeners such as γ- or δ-tocopherol or tocotrienols are more effective.3,7,10,18,37–39 Birringer et al.17 investigated antiproliferative effects of LCM of α- and δ-TOH in HepG2 cells. The authors reported highest growth inhibitory activity for carboxy-chromanols, whereas α-13′-OH or the metabolic precursors had no effect on HepG2 cell growth and the IC50 value of δ-13′-OH was >50 μM. In contrast to the results of Birringer et al.,17 the present study revealed also antiproliferative effects for α-13′-OH in LT97 cells. These discrepancies might be explained by a different susceptibility of the premalignant cells towards the hydroxylchromanol or a higher conversion rate of α-13′-OH to the more antiproliferative active metabolite α-13′-COOH in LT97 cells compared to HepG2 cells. Such higher conversion rate was observed by Birringer et al.17 for δ-13′-OH to its carboxy-metabolite compared to α-13′-OH in HepG2 probably mediating the antiproliferative effects of δ-13′-OH. Furthermore, the anticancer effects of α-13′-COOH in HepG2 cells were mediated by pro-apoptotic pathways including activation of caspase-3 and cleavage of PARP which are associated with ROS induction and mitochondrial damage.17 In the present study, no pro-oxidative action of α-13′-COOH or of α-13′-OH and the precursor α-TOH was observed since ROS levels were not elevated in LT97 cells. This might be due to lower sub-toxic concentrations used in the present study compared to the study of Birringer et al.,17 who used metabolites in a concentration of 20 μM. Interestingly, particularly α-13′-COOH reduced H2O2-induced ROS levels in LT97 cells. In addition, α-13′-COOH, α-13′-OH and α-TOH significantly reduced levels of H2O2-induced DNA damage indicating the antioxidant action and ROS scavenging capacity of tocopherol and its LCM used in sub-toxic concentrations. Therefore, an induction of ROS mediated apoptosis in LT97 cells might be excluded at least for the concentrations of metabolites used in the present study. Furthermore, the antigenotoxic effects indicate that α-TOH and its metabolites might protect non-transformed or early transformed cells such as the premalignant LT97 cells from DNA damage that could accumulate and contribute to further carcinogenic cell transformation. Chen et al. also showed that e.g. γ-TOH reduced DNA damage in a murine colon cancer model and discussed that the anticancer effects of tocopherols may be mainly mediated by protection against DNA damage at the early stage of colon carcinogenesis.36 Therefore, α-TOH and the LCM may act as so-called “blocking agents” in terms of primary chemoprevention by inhibiting the initiation of colon epithelial cells via increasing detoxification and reducing toxification (e.g. increase of antioxidant activity or expression of antioxidant enzymes involved in detoxification).40,41 The results of the present study indicate, that the antigenotoxic effects of α-TOH and its LCM are mediated predominantly by ROS-scavenging capacity because mRNA levels of detoxifying and antioxidant active enzymes were not affected in LT97 cells at least at the concentrations and time points of treatment used. The protective effects of different vitamin E congeners against DNA damage have already been demonstrated in several studies.42–44
Furthermore, to some extend α-TOH but in particular its metabolites might also act as “suppressing” agents in terms of secondary chemoprevention by suppressing the promotion of colon carcinogenesis (e.g. inhibiting proliferation and inducing apoptosis of initiated cells40,41). The treatment of LT97 cells with LCM for 24 h or 48 h resulted in reduced cell numbers, but an induction of caspase-3 activity as a marker of apoptosis was not observed. This is in contrast to results obtained by others14,17,18 and might again be explained by lower concentrations used in the present study. For example, Birringer et al.17 observed enhanced activation of caspase-3, -7 and -9 after 6 h of treatment in HepG2 cells with 20 μM α-13′-COOH and δ-13′-COOH, while in the study of Jang et al.14 caspase-9 cleavage was enhanced by δ-13′-COOH (10–20 μM) after 24 h in HCT116 cells. Therefore, it cannot be excluded that higher concentrations might induce caspase activity in LT97 cells, too. But the lack of caspase initiation observed in the present study might also indicate that the antiproliferative effects of α-13′-COOH and α-13′-OH are mediated by other cytotoxic mechanisms. For example, a caspase-independent programmed cell and paraptosis-like mechanism, as it was postulated for γ- and δ-tocotrienol in the human colon cancer cell line SW620,45,46 was accompanied by decreased levels of cyclin D1 gene expression. In the present study, cyclin D2 was analyzed and a marginal but insignificant decline in mRNA levels was observed, whereas p21 mRNA levels were not affected. In a recent study, Liao et al.47 demonstrated that α-13′-COOH modulated the cell cycle and insignificantly upregulated p21 gene expression in murine macrophages. Treatment of murine RAW264.7 macrophages with α-13′-COOH resulted in an induction of G0/G1 cell cycle arrest and apoptosis which was associated with a suppressed proteolytic activation of the sterol regulatory element-binding protein (SREBP)1 and with decreased cellular levels of stearoyl-CoA desaturase 1 (SCD1). The resulting shift from monounsaturated to saturated fatty acids in neutral lipids and phospholipids caused a decrease of the stress-preventive, pro-survival lipokine 1,2-dioleoyl-sn-glycero-3-phospho-(10-myo-inositol) [PI(18:1/18:1)]. Therefore, the antiproliferative effects of α-13′-COOH might also be mediated by interference with lipid metabolism and SCD1 activity resulting in a disturbed cellular lipid homeostasis and ER (endoplasmatic reticulum) function and an increased sensitivity to lipotoxicity and ferroptosis which triggers cell cycle arrest and apoptosis. Jang et al. already proposed an interference of antiproliferative effects of δ-13′-COOH observed in colon cancer cells with sphingolipid metabolism.14 Therefore, several mechanisms might be responsible for the observed antiproliferative effects of α-13′-COOH and α-13′-OH in LT97 colon adenoma cells.
In conclusion, α-TOH and its LCM might exert chemopreventive effects via antigenotoxic and ROS-scavenging mechanisms thereby preventing DNA damage while the metabolites trigger growth inhibition probably via inducing cell death which might be mediated by apoptosis and cell cycle arrest. But, for a better understanding of specific secondary chemopreventive effects, the exact mechanisms by which vitamin E metabolites induce cell death in premalignant colon cells such as LT97 and other colon cancer cell lines in comparison to non-transformed cells should be examined in further studies.
Data availability
All data published in this article are accessible from the corresponding author upon request.
Author contributions
Wiebke Schlörmann: conceptualization, validation, formal analysis, investigation, data curation, writing – original draft, writing – review & editing, visualization, supervision, project administration. Sijia Liao: formal analysis, investigation, data curation, writing – original draft, writing – review & editing. Tülin Dinc: formal analysis, investigation, data curation. Stefan Lorkowski: conceptualization, writing – review & editing, supervision, project administration, funding acquisition. Maria Wallert: conceptualization, validation, formal analysis, investigation, data curation, writing – review & editing, supervision. Michael Glei: conceptualization, writing – review & editing, supervision, project administration, funding acquisition. All authors have read and agreed to the published version of the manuscript.
Conflicts of interest
The authors declare no conflict of interest.
Acknowledgements
This IGF project (AiF 16642 BR) of the FEI (Research Association of the German Food Industry) was supported via AiF (German Federation of Industrial Research Associations) within the program for promoting the Industrial Collective Research (IGF) of the Federal Ministry of Economics and Climate Action (BMWK), based on a resolution of the German Parliament. We thank Esther Woschee for performing DCFH-DA assay. We also thank Marc Birringer, Stefan Kluge and Carsten Rohrer for performing synthesis of long-chain metabolites.
References
- H. M. Evans and K. S. Bishop, On the Existence of a Hitherto Unrecognized Dietary Factor Essential for Reproduction, Science, 1922, 56, 650–651 CrossRef CAS PubMed.
- M. Birringer, K. Siems, A. Maxones, J. Frank and S. Lorkowski, Natural 6-hydroxy-chromanols and -chromenols: structural diversity, biosynthetic pathways and health implications, RSC Adv., 2018, 8, 4803–4841 RSC.
- M. Wallert, S. Kluge, M. Schubert, A. Koeberle, O. Werz, M. Birringer and S. Lorkowski, Diversity of Chromanol and Chromenol Structures and Functions: An Emerging Class of Anti-Inflammatory and Anti-Carcinogenic Agents, Front. Pharmacol., 2020, 11, 362 CrossRef CAS PubMed.
- M. Wallert, L. Schmolz, F. Galli, M. Birringer and S. Lorkowski, Regulatory metabolites of vitamin E and their putative relevance for atherogenesis, Redox Biol., 2014, 2, 495–503 CrossRef CAS PubMed.
- R. Brigelius-Flohe, Vitamin E: the shrew waiting to be tamed, Free Radicals Biol. Med., 2009, 46, 543–554 CrossRef CAS.
- M. Schubert, S. Kluge, L. Schmolz, M. Wallert, F. Galli, M. Birringer and S. Lorkowski, Long-Chain Metabolites of Vitamin E: Metabolic Activation as a General Concept for Lipid-Soluble Vitamins?, Antioxidants, 2018, 7(10) CAS.
- Q. Jiang, Natural Forms of Vitamin E as Effective Agents for Cancer Prevention and Therapy, Adv. Nutr., 2017, 8, 850–867 CrossRef CAS.
- Q. Jiang, Natural forms of vitamin E and metabolites-regulation of cancer cell death and underlying mechanisms, IUBMB Life, 2019, 71, 495–506 CrossRef CAS PubMed.
- J. Ju, S. C. Picinich, Z. Yang, Y. Zhao, N. Suh, A. N. Kong and C. S. Yang, Cancer-preventive activities of tocopherols and tocotrienols, Carcinogenesis, 2010, 31, 533–542 CrossRef CAS.
- C. S. Yang, P. Luo, Z. Zeng, H. Wang, M. Malafa and N. Suh, Vitamin E and cancer prevention: Studies with different forms of tocopherols and tocotrienols, Mol. Carcinog., 2020, 59, 365–389 CrossRef CAS PubMed.
- S. M. Lippman, E. A. Klein, P. J. Goodman, M. S. Lucia, I. M. Thompson, L. G. Ford, H. L. Parnes, L. M. Minasian, J. M. Gaziano, J. A. Hartline, J. K. Parsons, J. D. Bearden 3rd, E. D. Crawford, G. E. Goodman, J. Claudio, E. Winquist, E. D. Cook, D. D. Karp, P. Walther, M. M. Lieber, A. R. Kristal, A. K. Darke, K. B. Arnold, P. A. Ganz, R. M. Santella, D. Albanes, P. R. Taylor, J. L. Probstfield, T. J. Jagpal, J. J. Crowley, F. L. Meyskens Jr., L. H. Baker and C. A. Coltman Jr., Effect of selenium and vitamin E on risk of prostate cancer and other cancers: the Selenium and Vitamin E Cancer Prevention Trial (SELECT), J. Am. Med. Assoc., 2009, 301, 39–51 CrossRef CAS PubMed.
- Q. Jiang, S. Im, J. G. Wagner, M. L. Hernandez and D. B. Peden, Gamma-tocopherol, a major form of vitamin E in diets: Insights into antioxidant and anti-inflammatory effects, mechanisms, and roles in disease management, Free Radicals Biol. Med., 2022, 178, 347–359 CrossRef CAS PubMed.
- Q. Jiang, J. Wong, H. Fyrst, J. D. Saba and B. N. Ames, gamma-Tocopherol or combinations of vitamin E forms induce cell death in human prostate cancer cells by interrupting sphingolipid synthesis, Proc. Natl. Acad. Sci. U. S. A., 2004, 101, 17825–17830 CrossRef CAS.
- Y. Jang, N. Y. Park, A. L. Rostgaard-Hansen, J. Huang and Q. Jiang, Vitamin E metabolite 13′-carboxychromanols inhibit pro-inflammatory enzymes, induce apoptosis and autophagy in human cancer cells by modulating sphingolipids and suppress colon tumor development in mice, Free Radicals Biol. Med., 2016, 95, 190–199 CrossRef CAS PubMed.
- A. Gopalan, W. Yu, Q. Jiang, Y. Jang, B. G. Sanders and K. Kline, Involvement of de novo ceramide synthesis in gamma-tocopherol and gamma-tocotrienol-induced apoptosis in human breast cancer cells, Mol. Nutr. Food Res., 2012, 56, 1803–1811 CrossRef CAS PubMed.
- L. Schmölz, M. Birringer, S. Lorkowski and M. Wallert, Complexity of vitamin E metabolism, World J. Biol. Chem., 2016, 7, 14–43 CrossRef.
- M. Birringer, D. Lington, S. Vertuani, S. Manfredini, D. Scharlau, M. Glei and M. Ristow, Proapoptotic effects of long-chain vitamin E metabolites in HepG2 cells are mediated by oxidative stress, Free Radicals Biol. Med., 2010, 49, 1315–1322 CrossRef CAS.
- S. E. Campbell, W. L. Stone, S. Lee, S. Whaley, H. Yang, M. Qui, P. Goforth, D. Sherman, D. McHaffie and K. Krishnan, Comparative effects of RRR-alpha- and RRR-gamma-tocopherol on proliferation and apoptosis in human colon cancer cell lines, BMC Cancer, 2006, 6, 13 CrossRef.
- R. Gysin, A. Azzi and T. Visarius, Gamma-tocopherol inhibits human cancer cell cycle progression and cell proliferation by down-regulation of cyclins, FASEB J., 2002, 16, 1952–1954 CrossRef CAS PubMed.
- K. Terashima, T. Shimamura, M. Tanabayashi, M. Aqil, J. A. Akinniyi and M. Niwa, Constituents of the seeds of Garcinia kola: Two new antioxidants, garcinoic acid and garcinal, Heterocycles, 1997, 45, 1559–1566 CrossRef CAS.
- M. Richter, D. Jurek, F. Wrba, K. Kaserer, G. Wurzer, J. Karner-Hanusch and B. Marian, Cells obtained from colorectal microadenomas mirror early premalignant growth patterns in vitro, Eur. J. Cancer, 2002, 38, 1937–1945 CrossRef CAS.
- W. Schlörmann, C. Horlebein, S. M. Hübner, E. Wittwer and M. Glei, Potential Role of ROS in Butyrate- and Dietary Fiber-Mediated Growth Inhibition and Modulation of Cell Cycle-, Apoptosis- and Antioxidant-Relevant Proteins in LT97 Colon Adenoma and HT29 Colon Carcinoma Cells, Cancers, 2023, 15(2), 440 CrossRef PubMed.
- W. Schlörmann, J. A. Bockwoldt, M. F. Mayr, S. Lorkowski, C. Dawczynski, S. Rohn, M. A. Ehrmann and M. Glei, Fermentation profile, cholesterol-reducing properties and chemopreventive potential of beta-glucans from Levilactobacillus brevis and Pediococcus claussenii - a comparative study with beta-glucans from different sources, Food Funct., 2021, 12, 10615–10631 RSC.
- M. Glei, S. Fischer, J. Lamberty, D. Ludwig, S. Lorkowski and W. Schlörmann, Chemopreventive Potential of In Vitro Fermented Raw and Roasted Hazelnuts in LT97 Colon Adenoma Cells, Anticancer Res., 2018, 38, 83–93 Search PubMed.
- M. Glei, T. Schneider and W. Schlörmann, Comet assay: an essential tool in toxicological research, Arch. Toxicol., 2016, 90, 2315–2336 CrossRef CAS.
- J. Pelka, H. Gehrke, M. Esselen, M. Turk, M. Crone, S. Brase, T. Muller, H. Blank, W. Send, V. Zibat, P. Brenner, R. Schneider, D. Gerthsen and D. Marko, Cellular uptake of platinum nanoparticles in human colon carcinoma cells and their impact on cellular redox systems and DNA integrity, Chem. Res. Toxicol., 2009, 22, 649–659 Search PubMed.
- K. Stolle, M. Schnoor, G. Fuellen, M. Spitzer, P. Cullen and S. Lorkowski, Cloning, genomic organization, and tissue-specific expression of the RASL11B gene, Biochim. Biophys. Acta, 2007, 1769, 514–524 CrossRef CAS.
- K. Stolle, M. Schnoor, G. Fuellen, M. Spitzer, T. Engel, F. Spener, P. Cullen and S. Lorkowski, Cloning, cellular localization, genomic organization, and tissue-specific expression of the TGFbeta1-inducible SMAP-5 gene, Gene, 2005, 351, 119–130 CrossRef CAS PubMed.
- M. Wallert, L. Schmolz, A. Koeberle, V. Krauth, M. Glei, F. Galli, O. Werz, M. Birringer and S. Lorkowski, alpha-Tocopherol long-chain metabolite alpha-13′-COOH affects the inflammatory response of lipopolysaccharide-activated murine RAW264.7 macrophages, Mol. Nutr. Food Res., 2015, 59, 1524–1534 CrossRef CAS.
- M. W. Pfaffl, G. W. Horgan and L. Dempfle, Relative expression software tool (REST) for group-wise comparison and statistical analysis of relative expression results in real-time PCR, Nucleic Acids Res., 2002, 30, 36 CrossRef PubMed.
- E. A. Klein, I. M. Thompson Jr., C. M. Tangen, J. J. Crowley, M. S. Lucia, P. J. Goodman, L. M. Minasian, L. G. Ford, H. L. Parnes, J. M. Gaziano, D. D. Karp, M. M. Lieber, P. J. Walther, L. Klotz, J. K. Parsons, J. L. Chin, A. K. Darke, S. M. Lippman, G. E. Goodman, F. L. Meyskens Jr. and L. H. Baker, Vitamin E and the risk of prostate cancer: the Selenium and Vitamin E Cancer Prevention Trial (SELECT), J. Am. Med. Assoc., 2011, 306, 1549–1556 CrossRef CAS.
- Q. Jiang, Z. Jiang, Y. J. Hall, Y. Jang, P. W. Snyder, C. Bain, J. Huang, A. Jannasch, B. Cooper, Y. Wang and M. Moreland, Gamma-tocopherol attenuates moderate but not severe colitis and suppresses moderate colitis-promoted colon tumorigenesis in mice, Free Radicals Biol. Med., 2013, 65, 1069–1077 CrossRef CAS.
- C. Yang, Y. Zhao, S. Im, C. Nakatsu, Y. Jones-Hall and Q. Jiang, Vitamin E delta-tocotrienol and metabolite 13′-carboxychromanol inhibit colitis-associated colon tumorigenesis and modulate gut microbiota in mice, J. Nutr. Biochem., 2021, 89, 108567 CrossRef CAS PubMed.
- H. Yang, M. Xu, F. Lu, Q. N. Zhang, Y. Q. Feng, C. S. Yang, N. Li and X. D. Jia, Tocopherols inhibit esophageal carcinogenesis through attenuating NF-κB activation and CXCR3-mediated inflammation, Oncogene, 2018, 37, 3909–3923 CrossRef CAS PubMed.
- H. J. Lee, J. Ju, S. Paul, J. Y. So, A. DeCastro, A. Smolarek, M. J. Lee, C. S. Yang, H. L. Newmark and N. Suh, Mixed tocopherols prevent mammary tumorigenesis by inhibiting estrogen action and activating PPAR-gamma, Clin. Cancer Res., 2009, 15, 4242–4249 CrossRef CAS.
- J. X. Chen, A. Liu, M. J. Lee, H. Wang, S. Yu, E. Chi, K. Reuhl, N. Suh and C. S. Yang, delta- and gamma-tocopherols inhibit phIP/DSS-induced colon carcinogenesis by protection against early cellular and DNA damages, Mol. Carcinog., 2017, 56, 172–183 CrossRef CAS PubMed.
- F. Guan, G. Li, A. B. Liu, M. J. Lee, Z. Yang, Y. K. Chen, Y. Lin, W. Shih and C. S. Yang, delta- and gamma-tocopherols, but not alpha-tocopherol, inhibit colon carcinogenesis in azoxymethane-treated F344 rats, Cancer Prev. Res., 2012, 5, 644–654 CrossRef CAS.
- G. X. Li, M. J. Lee, A. B. Liu, Z. Yang, Y. Lin, W. J. Shih and C. S. Yang, delta-tocopherol is more active than alpha - or gamma -tocopherol in inhibiting lung tumorigenesis in vivo, Cancer Prev. Res., 2011, 4, 404–413 CrossRef CAS.
- A. K. Smolarek, J. Y. So, B. Burgess, A. N. Kong, K. Reuhl, Y. Lin, W. J. Shih, G. Li, M. J. Lee, Y. K. Chen, C. S. Yang and N. Suh, Dietary administration of delta- and gamma-tocopherol inhibits tumorigenesis in the animal model of estrogen receptor-positive, but not HER-2 breast cancer, Cancer Prev. Res., 2012, 5, 1310–1320 CrossRef CAS PubMed.
- D. Scharlau, A. Borowicki, N. Habermann, T. Hofmann, S. Klenow, C. Miene, U. Munjal, K. Stein and M. Glei, Mechanisms of primary cancer prevention by butyrate and other products formed during gut flora-mediated fermentation of dietary fibre, Mutat. Res., 2009, 682, 39–53 CAS.
- W. P. Steward and K. Brown, Cancer chemoprevention: a rapidly evolving field, Br. J. Cancer, 2013, 109, 1–7 CrossRef CAS.
- G. J. Delinasios, M. Karbaschi, M. S. Cooke and A. R. Young, Vitamin E inhibits the UVAI induction of “light” and “dark” cyclobutane pyrimidine dimers, and oxidatively generated DNA damage, in keratinocytes, Sci. Rep., 2018, 8, 423 CrossRef PubMed.
- O. Fantappie, M. Lodovici, P. Fabrizio, S. Marchettia, V. Fabbroni, M. Solazzo, N. Lasagna, P. Pantaleo and R. Mazzanti, Vitamin E protects DNA from oxidative damage in human hepatocellular carcinoma cell lines, Free Radicals Res., 2004, 38, 751–759 CrossRef CAS.
- E. Horvathova, D. Slamenova, L. Hlincikova, T. K. Mandal, A. Gabelova and A. R. Collins, The nature and origin of DNA single-strand breaks determined with the comet assay, Mutat. Res., 1998, 409, 163–171 CAS.
- J. S. Zhang, D. M. Li, N. He, Y. H. Liu, C. H. Wang, S. Q. Jiang, B. Q. Chen and J. R. Liu, A paraptosis-like cell death induced by delta-tocotrienol in human colon carcinoma SW620 cells is associated with the suppression of the Wnt signaling pathway, Toxicology, 2011, 285, 8–17 CrossRef CAS PubMed.
- J. S. Zhang, D. M. Li, Y. Ma, N. He, Q. Gu, F. S. Wang, S. Q. Jiang, B. Q. Chen and J. R. Liu, gamma-Tocotrienol induces paraptosis-like cell death in human colon carcinoma SW620 cells, PLoS One, 2013, 8, e57779 CrossRef CAS PubMed.
- S. Liao, A. Gollowitzer, L. Bormel, C. Maier, L. Gottschalk, O. Werz, M. Wallert, A. Koeberle and S. Lorkowski, alpha-Tocopherol-13′-Carboxychromanol Induces Cell Cycle Arrest and Cell Death by Inhibiting the SREBP1-SCD1 Axis and Causing Imbalance in Lipid Desaturation, Int. J. Mol. Sci., 2023, 24(11), 9229 CrossRef CAS PubMed.
|
This journal is © The Royal Society of Chemistry 2024 |
Click here to see how this site uses Cookies. View our privacy policy here.