DOI:
10.1039/D3FO05014A
(Paper)
Food Funct., 2024,
15, 2422-2432
Urolithin A production drives the effects of pomegranate on the gut microbial metabolism of bile acids and cholesterol in mild dyslipidaemic overweight and obese individuals
Received
14th November 2023
, Accepted 22nd January 2024
First published on 23rd January 2024
Abstract
The metabolism of (poly)phenols and some host metabolites, including bile acids (BAs) and cholesterol, varies among individuals depending on their gut microbiota. The gut microbial metabolism of ellagitannins (ETs) and ellagic acid (EA) produces urolithins (Uros), yielding three metabotypes with quantitative and qualitative differences based on dissimilar Uro-producing profiles (UM-A, UM-B, and UM-0, i.e., non-producers). Previous animal studies demonstrated that polyphenols impact BAs and cholesterol microbial metabolism, but data on their effects in humans and data regarding the inter-individual variability of these metabolic conversions are scant. We evaluated whether UMs, as distinctive functional gut-microbiome signatures, could determine the potential effect of a pomegranate extract (PE) rich in ET-EA on the metabolism of BAs and cholesterol in mild dyslipidaemic overweight-obese individuals, with possible consequences on host-lipid homeostasis and gut health. At the baseline, UM-B presented the highest levels of faecal total and secondary BAs and coprostanol, suggesting that the lipid absorption capacity and gut cytotoxic risk could be augmented in UM-B. PE intake significantly reduced faecal coprostanol and BA production, especially secondary BAs, and modulated the gut microbiome, reducing the gut cytotoxic risk, especially in UM-B individuals. The lowering of faecal microbial coprostanol and BAs and some BA-metabolising bacteria was quantitatively correlated with Uro concentrations, mainly faecal Uro-A. This suggests that PE consumption could exert cardiovascular and gut protection through Uro-A production as a direct driver of the effects and indirectly by reducing the Coriobacteriaceae family and BA pool, known factors involved in the gut absorption of lipids.
Introduction
The human intestine is colonised by several microbial species that interact with dietary and host-derived molecules in the intestine, significantly contributing to host physiology.1 The gut microbiota can produce different metabolites from several dietary compounds, which exert biological activity and play an important role in maintaining gut and metabolic health. This is the case of ellagitannins (ETs) and ellagic acid (EA) from pomegranate, nuts, and berries, which are extensively metabolised by the human gut microbiota to produce different urolithins (Uros) with potential health benefits.2 The human gut microbiota can also transform host-derived molecules, such as cholesterol and bile acids (BAs), which are important signalling metabolites in the host. Indeed, cholesterol and BAs significantly impact host lipid homeostasis.3,4 However, there is a consistent human inter-individual variability in the microbial metabolism of dietary and host-derived molecules depending on the subjects’ gut microbial signatures.5–7
One of the main differences between the metabolic profiles associated with ET and EA metabolism is the final Uros produced (urolithin metabotypes, UMs). UM-A individuals only yield urolithin A (Uro-A) as the final catabolite. In contrast, UM-B subjects produce Uro-A and, distinctively, also isourolithin A (IsoUro-A) and urolithin B (Uro-B). Finally, UM-0 individuals cannot produce intermediate and final Uros in the ET–EA metabolic pathway.8 Differences in the human gut microbial ecologies associated with UMs were previously described.9 UMs have also been proposed as potential cardiovascular disease risk biomarkers because the blood lipid profile was reported to be associated with UMs.10,11 However, the link for this association remains unknown so far. On the other hand, recent studies have also revealed inter-individual differences in the microbial metabolism of cholesterol and conjugated primary BAs, which might exert a much wider range of biological activities than those initially recognised.4,12–14 In this regard, secondary metabolites of faecal cholesterol and BAs, produced by the gut microbiota, may promote health or favour disease development depending on the quantity and type produced. Specifically, continuous exposure to elevated luminal levels of secondary BAs and cholesterol microbial metabolites might increase gut permeability and susceptibility to intestinal inflammation and colorectal cancer.3 In addition to the toxic effects of secondary steroids per se, the concentration of putrefactive compounds could also increase gut toxicity due to the release of amino acids (glycine and taurine), which are the products of the microbial hydrolysis of BAs.12
The possibility of modulating the microbial metabolism of dietary and host-derived molecules by targeting the gut microbiota composition to improve human health has only recently started to be explored.12,14 Indeed, some animal studies have begun to evaluate the impact of some polyphenols on the gut microbial transformations of BAs and cholesterol, as reviewed previously.12,14 These results suggest that phenolic-rich diets hamper the conversion of primary BAs to secondary BAs and cholesterol to coprostanol.12 However, further studies are crucial to establish whether the effects observed in animal studies can be replicated in humans, and more data regarding the role of the gut microbial metabolism of polyphenols in the inter-individual differences of these metabolic conversions are needed.
In the POMEcardio trial, we described the UM-dependent effects on cardiovascular risk markers after consuming a pomegranate extract (PE) in overweight-obese individuals with mild dyslipidemia.10 In the present study, we aimed to evaluate (i) the effects of PE intake in these volunteers on the gut microbial metabolism of BAs and cholesterol, (ii) whether these effects of PE could also be Uro-dose-dependent, (iii) the possible link between BAs and cholesterol metabolism and the gut microbiota signature associated with UMs, and (iv) establish a possible link between BAs and cholesterol metabolism, Uro production capacity, microbial relative abundances and cardiovascular risk markers improvement. To this purpose, the results were analysed considering the participants as a single group and after clustering the volunteers according to their UMs (UM-A, UM-B, and UM-0).
Materials and methods
Chemical and reagents
Standards of cholic acid (CA), chenodeoxycholic acid (CDCA), deoxycholic acid (DCA), lithocholic acid (LCA), isolithocholic acid (iso-LCA), ursodeoxycholic acid (UDCA), coprostanol, cholesterol, 5α-cholestane, sodium acetate, and sodium hydroxide were purchased from Sigma-Aldrich (St Louis, MO, USA). Uro-A, IsoUro-A, Uro-B, and their derived phase-II conjugated metabolites were chemically synthesised and purified by Villapharma Research S.L. (Parque Tecnológico de Fuente Álamo, Murcia, Spain). HPLC-grade acetonitrile, hexane, cyclohexane, methanol, and ethanol were purchased from JT Baker (Deventer, The Netherlands), and formic acid was purchased from Panreac (Barcelona, Spain). Milli-Q system (Millipore Corp., Bedford, MA, USA) ultrapure water was used throughout the study. PE was encapsulated in identical hard gelatin capsules supplied by Laboratorios Admira S.L. (Alcantarilla, Murcia, Spain), following the European Union's good manufacturing practices. Capsules were bottled with a specific blind code for participants and researchers. Each capsule (450 mg of PE) contained 160 mg of phenolic compounds, as previously described.10 The phenolic content of PE capsules was analyzed by HPLC-DAD-ESI-MS/MS, as previously described, and was as follows: 54.5 ± 1.2 mg punicalagins, 45.4 ± 0.9 mg free ellagic acid, 2.7 ± 0.2 mg punicalin, 2.6 ± 0.1 mg ellagic acid-hexoside and 1.2 ± 0.1 mg ellagic acid-pentoside.10 After acid hydrolysis, the total ellagitannin content per capsule was 72.9 ± 1.1 mg free ellagic acid, 69.3 ± 0.9 mg gallagic acid dilactone, 10.3 ± 0.1 mg sanguisorbic acid, 3.1 ± 0.04 mg gallic acid, 2.9 ± 0.2 mg punicalin and 1.9 ± 0.2 mg valoneic acid dilactone.10
Study design and subjects
Urine, faecal and blood samples were obtained in a previous trial (POMEcardio), included in the European ‘Bacchus Project’ (FP7-KBBE-2012), registered at clinicaltrials.gov (NCT02061098) and approved by the Spanish National Research Council's Bioethics Committee (CSIC, Madrid, Spain) (reference 312090), following the ethical guidelines outlined in the Declaration of Helsinki (1975) and its amendments. Briefly, that study was designed to evaluate the effect of PE consumption in subjects with mild hyperlipidemia and potentially increased gut dysbiosis. Overweight-obese subjects (BMI > 27 kg m−2) over 40 years, with no diagnosed chronic disease, were included. Fifty participants were recruited, and 49 completed the protocol. Detailed information about the design is shown elsewhere.10 Briefly, the study was a 6-month follow-up, double-blind, crossover, dose-response, randomized, and placebo-controlled trial. The study consisted of two test phases with each test phase lasting for 3 weeks with a 3-week washout period between each test phase and a 3-week washout period after the final test phase. In the first test phase, participants consumed either one capsule of PE or placebo daily in a crossover fashion. In the second test phase, the same design was followed but volunteers ingested either four capsules of PE or placebo daily.10 In the present study, considering the absence of effects after consuming the placebo and the effects observed with PE at the maximum dose tested, samples from two time points were analysed: after consuming one capsule of PE daily for 3 days and four capsules daily for 3 weeks.
Sampling procedures
As described previously,10 participants provided urine and faecal samples on the morning of each visit. Blood samples were collected in vacutainers between 8
:
00 and 9
:
00 a.m. to minimise circadian variations in the supine position after 20 min of rest. Serum and plasma were obtained. All samples were stored at −80 °C until further analysis.10
Blood lipid profile and urolithin metabotype determination
Serobiochemical variables were measured as previously reported, including Tchol (total cholesterol), HDLc (high-density lipoprotein cholesterol), LDLc (low-density lipoprotein cholesterol), VLDLc (very low-density lipoprotein cholesterol), TG (triglycerides), ApoA-1 (apolipoprotein A-1), ApoB (apolipoprotein B), oxLDLc (oxidised LDLc), IDLc (intermediate-density lipoprotein cholesterol), and HOMA-IR (Homeostatic Model Assessment for Insulin Resistance).10 Urine and faecal samples were processed and analysed by using an ultra-performance liquid chromatograph coupled to a quadrupole-time-of-flight mass spectrometer using an electrospray interface (UPLC-ESI-QTOF-MS) to determine urolithins as described elsewhere.10,15 At baseline, urine samples after consumption of 1 capsule for 3 days were used to stratify volunteers by UMs.10
Faecal cholesterol and coprostanol analyses
Cholesterol and its microbial metabolite coprostanol were extracted from faecal samples as previously described with minor modifications.16 Briefly, 50 μL of diluted faeces (1
:
10 with H2O Milli-Q) were spiked with 20 μL of 5α-cholestane (5 mmol L−1) as an internal standard for sterol determination. The hydrolysis process was carried out with 5 mL of freshly prepared methanolic sodium hydroxide (1 mol L−1, 90% methanol) for 60 min at 70 °C. The neutral sterols were extracted with 4 mL of cyclohexane using a vortex mixer for 20 min. Samples were centrifuged at 3500g for 5 min, and the organic phase was separated and evaporated in a speed vacuum concentrator (Savant SPD121P, Thermo Fisher Scientific, Madrid, Spain). The dried samples were then reconstituted in 1 mL of hexane and filtered through a 0.22 μm polyvinylidene fluoride (PVDF) filter (Millipore, Thermo Fisher Scientific, Madrid, Spain). The faecal cholesterol and coprostanol levels were then determined by gas chromatography (GC) coupled to mass spectrometry (MS).16 An aliquot of 1 μL of sample was injected into the GC-MS system. Separation was performed using an Agilent 7890A GC equipped with an Agilent 5975C MS detector. Cholesterol and coprostanol were separated on an HP-5MS 30 m x 0.25 mm (i.d.) capillary column (Agilent) with helium as the carrier gas (1 mL min−1). Injections were carried out in a splitless mode at 280 °C. The oven temperature was first held at 150 °C for 5 min, and then increased to 280 °C at a rate of 20 °C min−1, which was maintained for 10 min. Electron impact mass spectra (EI-MS) were recorded with an ionisation voltage of 70 eV and a temperature source of 230 °C. The acquisition was performed in scanning mode. Identification of cholesterol and coprostanol was based on the retention time of standard compounds and with the assistance of the NIST 08 libraries.
Faecal BA determination
BAs were extracted from faecal samples following a validated method with minor modifications.17 Fifty μL of diluted faeces (1
:
10 with H2O Milli-Q) were resuspended in 450 μL of cold sodium acetate buffer (50 mmol L−1, pH 5.6). 1.5 mL of pure ethanol was added, and the samples were shaken at 1200 rpm for 15 min at 25 °C (ThermoCell Mixing Block MB-102, BIOER, Hangzhou, China). After centrifugation, the supernatant was diluted four times with H2O Milli-Q and applied to a Chromafix C18 S cartridge (270 mg) (Macherey-Nagel, Düren, Germany), which was previously conditioned with 3 mL of ethanol and then 3 mL of water Milli-Q. Following the sample loading, the cartridge was washed with 3 mL of Milli-Q water to remove water-soluble impurities. Finally, BAs were eluted with 3 mL of ethanol and dried using a speed vacuum concentrator. The residue was resuspended in 200 μL of methanol and filtered through a 0.22 μm PVDF filter. The samples were diluted in methanol (1
:
10) before injection into an Agilent 1290 Infinity UPLC system coupled to the 6550 Accurate-Mass quadrupole TOF mass spectrometer (Agilent Technologies, Waldbronn, Germany) using an electrospray interface with Jet Stream technology. Separation was achieved using a reversed-phase Poroshell 120 EC-C18 column (3 × 100 mm, 2.7 μm) (Agilent Technologies, Waldbronn, Germany) operating at 25 °C. The mobile phases used were water
:
formic acid (99.9
:
0.1 v/v; phase A) and acetonitrile
:
formic acid (99.9
:
0.1 v/v; phase B). BAs were separated using the following gradient conditions: 0–4 min, 50–90% phase-B; 4–7 min, 90–99% phase-B; 7–10 min, 99% phase-B. Finally, the system returned to the initial conditions (50% phase-B) for 1 min, and the column was re-equilibrated for an additional 1 minute. The flow rate was constant at 0.4 mL min−1, and the injection volume was 2 μL. The operating conditions were as follows: gas temperature – 150 °C, drying nitrogen gas – 14 L min−1, nebuliser pressure – 40 psi, sheath gas temperature – 350 °C, sheath gas flow – 11 L min−1. Spectra were acquired in single MS mode with a mass range between m/z 50 and 1500, negative polarity, and a TOF spectral acquisition rate of 1.5 spectra per second. Before the analysis, the instrument was calibrated externally by injecting a mixture of reference compounds (Tuning Mix) to ensure mass accuracy during the MS analyses. Continuous internal calibration was performed during analyses using the signals at m/z 112.9855 and m/z 1033.9881. Data were acquired using the Mass Hunter Workstation software (version B.08.00, Service Pack 1, Agilent Technologies) and processed using the Mass Hunter Qualitative Analysis software (version B.08.00, Service Pack 1, Agilent Technologies). All samples were injected in the same batch, and the order of sample injection was randomised to prevent sample bias. The quantification of BAs was determined by their interpolation within the calibration curve obtained by the injection of their corresponding standards by peak area integration of their extracted ion chromatograms.
Gut microbiota analysis
Gut microbiota analysis was performed by 16S rDNA sequencing (targeting the V3–V4 hypervariable regions) on a MiSeq-Illumina platform (FISABIO sequencing service, Valencia, Spain) after extracting bacterial DNA from the faecal samples as detailed elsewhere.18 The obtained data were processed to construct Operational Taxonomic Unit (OTU) tables at a 97% similarity threshold to classify taxa at the genus level, determine the phyla and families to which they belong, and estimate the relative abundance of each OTU, as previously described19 but using the last updated RDP classifier database 2.13. Furthermore, in the present study, the individuals were clustered by UMs and the differences in relative abundances before and after PE consumption were evaluated through the linear discriminant analysis effect size (LEfSe) algorithm using the online interface Galaxy (https://huttenhower.sph.harvard.edu/galaxy/root).
Statistical analysis
Statistical analyses were performed using the SPSS software v.28.0 (SPSS Inc., Chicago, IL, USA), and plots were generated using GraphPad Prism 7.0 (GraphPad Software, San Diego, CA, USA). The Shapiro–Wilk test was used to examine data normality. Differences between two dependent groups (i.e., before versus after PE consumption) were analysed using the paired Student's t-test or Wilcoxon signed rank test when data distribution was normal or non-normal, respectively. The Kruskal–Wallis test, followed by Dunn's test, was used to compare more than two groups (i.e., UM-A vs. UM-B vs. UM-0), and the corresponding pairwise comparisons were adjusted by the Bonferroni correction for multiple tests. Spearman's rank correlation was performed to study associations between variables (faecal cholesterol and BAs, blood lipid profile, and specific bacterial groups). A multivariate model was applied to evaluate the interaction effect between UM and time points on BA concentration. Statistical significance was set at *P < 0.05.
Results
Effect of PE intake on the phenolic metabolite profile
Fig. 1 shows the inter-individual differences in Uro-A concentrations in the faeces and urine from the overweight-obese individuals after 3-week intake of four daily capsules of PE. The faecal Uro-A concentration range was higher in the faeces from UM-A (4–423 μg g−1 faeces) than in the UM-B group (2–180 μg g−1 faeces), whereas the urinary Uro-A concentration range was similar in the UM-A (0.1–86 mg per 24 h in urine) and UM-B (0.1–89 mg per 24 h in urine) groups (Fig. 1A and B).
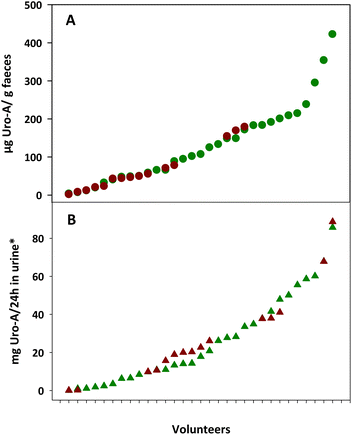 |
| Fig. 1 Faecal (A) and urinary (B) urolithin A (Uro-A) in urolithin metabotype A (green plots) and urolithin metabotype B (red plots) volunteers after 3-week intake of four daily capsules of PE. *Estimated values that were calculated considering that 1.3 g is the mean excretion of creatinine over a 24-hour period. | |
Effect of PE intake on faecal BAs and cholesterol metabolism
Fig. 2 shows the concentration of the faecal BAs and sterols analysed in the overweight-obese individuals at baseline and after a 3-week intake of 4 capsules of PE. At baseline, secondary BAs (DCA and LCA) concentration predominated in the faeces compared to primary BAs (CA and CDCA) (Fig. 2B and C). The total BAs, secondary BAs, coprostanol and the coprostanol/cholesterol ratio were significantly lower in the UM-0 than in the UM-B group (Fig. 2A, F, G, K and L). In contrast, the primary BA concentration and faecal cholesterol did not differ among UMs at baseline (Fig. 2B and J).
 |
| Fig. 2 Faecal BAs (A-I) and sterols (J-L) at baseline (blue bars) and after consuming PE for 3 weeks (red bars) in all volunteer samples (“All”, n = 49) or in those grouped by urolithin metabotypes: UM-0 (metabotype 0, n = 6), UM-A (metabotype A, n = 29) and UM-B (metabotype B, n = 14). *Significant differences (P < 0.05). | |
After PE consumption for 3 weeks, a significant reduction of total BAs, primary BAs, secondary BAs, CA, CDCA, DCA, LCA, UDCA, iso-LCA and coprostanol was observed when all volunteers were considered and in the UM-A and UM-B subgroups (Fig. 2). The coprostanol/cholesterol ratio was also reduced upon the 3-week intake of PE when all volunteers were considered and in the UM-A group, but was not significantly reduced in the UM-B group (Fig. 2L). In contrast, these parameter reductions were not observed after PE intake in the UM-0 group (Fig. 2). In addition, faecal cholesterol was not significantly reduced, although in the case of the UM-B group, it was nearly statistically significant (P = 0.056) (Fig. 2J).
Effect of PE intake on the gut microbiota composition
Linear discriminant analysis (LDA) from LEfSe analyses showed an increase in the relative abundance of some bacterial groups, including the well-known butyrate-producing bacteria Odoribacter (family Odoribacteraceae), Dysosmobacter (family Oscillospiraceae) and Peptoniphilus (family Peptoniphilaceae) in all volunteers after 3 weeks of PE consumption (Fig. 3A). Other groups also increased after PE consumption, such as the propionate-producing genera Phocaeicola (family Bacteroidaceae) (Fig. 3A). On the other hand, PE consumption decreased the abundance of the BA-deconjugating bacteria involved in the amino acid hydrolysis from primary BAs such as Romboutsia (family Clostridiaceae), as well as bacteria involved in primary BA metabolism to secondary and tertiary BAs, such as Sarcina (family Clostridiaceae) and the family Coriobacteriaceae (class Coriobacteriia) (Fig. 3A). When the volunteers were stratified according to their UMs, the modulation of the gut microbiota by PE differed depending on the UM (Fig. 3). For example, bacterial groups that increased by PE in all volunteers group (Fig. 3A) coincided with those observed in UM-A volunteers (Fig. 3B) but not in UM-B volunteers, where no bacteria increase was observed (Fig. 3C). In addition, the genus Flavonifractor increased after PE consumption only in the UM-A group (Fig. 3B). In contrast, some bacterial groups reduced by PE in all volunteers such as Hydrogenoanaerobacterium, Anaerofustis and the family Coriobacteriaceae (Fig. 3A) coincided with those reduced in UM-B (Fig. 3C), but not with those of UM-A, where other bacteria, such as the well-known bile-resistant and BA-metabolising bacteria Acinetobacter (family Moraxellaceae), Parvimonas (family Peptoniphilaceae), Paeniclostridium (family Clostridiaceae) and unclassified-Enterobacteriaceae were reduced (Fig. 3B). In addition, BA-deconjugating and BA-metabolising bacteria, such as Collinsella (family Coriobacteriaceae), decreased after PE consumption, but only in the UM-B group (Fig. 3C). In the case of the UM-0 group, the corresponding LDA score from LEfSe analyses showed that PE consumption did not significantly modify the gut microbiota composition (Fig. 3D).
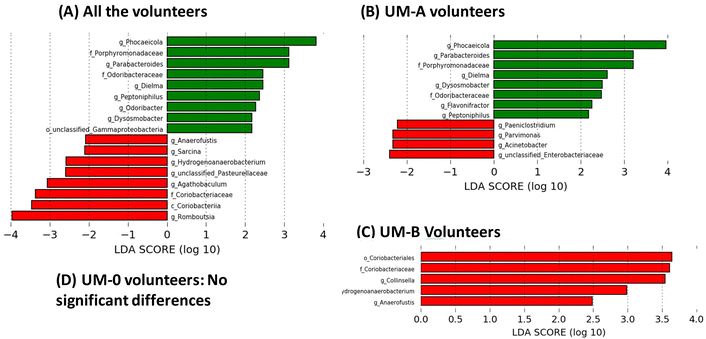 |
| Fig. 3 Linear discriminant analysis (LDA) effect size (LEfSe) of the gut microbiota that shows significant differences in the faecal microbiome at baseline (red bars) and after consuming PE for 3 weeks (green bars) in all volunteers (A) or in those grouped by urolithin metabotypes (B, C and D): UM-A (metabotype A, n = 29), UM-B (metabotype B, n = 14) and UM-0 (metabotype 0, n = 6). | |
Associations between urolithins, faecal BAs and sterols, gut microbiota, and clinical serobiochemical variables
The correlation analysis of quantitative variables revealed that both the total Uros and Uro-A were negatively correlated with total, primary, secondary and tertiary BAs (Fig. 4A). Accordingly, CA and CDCA (primary BAs), DCA and LCA (secondary BAs), and UDCA and Iso-LCA showed negative correlations with faecal total Uros and Uro-A (present in UM-A and UM-B). Urinary and faecal Uro-B (only present in UM-B) correlated negatively only with UCDA. Therefore, the faecal Uro-A concentration was the only metabolite correlated with all the faecal BAs analysed. Coprostanol was also negatively associated with faecal Uro-A, unlike faecal cholesterol and the coprostanol/cholesterol ratio (Fig. 4A). In contrast, urinary and faecal Uro-B negatively correlated with faecal cholesterol. The correlation analysis of faecal microbiota relative abundances revealed that some discriminating genera upon PE consumption correlated with microbial metabolites (Fig. 4B). For example, some bacteria that increased after PE consumption (Fig. 3), such as the propionate-producing Phocaeicola, the butyrate-producing Odoribacter as well as Parabacteroides, were positively correlated with faecal Uro-A, and negatively correlated with primary, secondary, tertiary, and total BAs (Fig. 4B). In contrast, some BA-deconjugating and BA-metabolising bacteria decreased after PE consumption (Fig. 3) and correlated with the reduction of BAs, especially secondary and tertiary BAs (Fig. 4B). These bacteria included a BA-deconjugating genus like Romboutsia, and bile-resistant and BA-metabolising bacteria like Sarcina (family Clostridiaceae), Acinetobacter (family Moraxellaceae), Parvimonas (family Peptoniphilaceae) and Collinsella, as well as its family (Coriobacteriaceae) and class (Coriobacteriia). Furthermore, these bacteria were negatively associated with faecal Uro-A, but this association did not reach statistical significance in the case of Romboutsia (P = 0.053) and Parvimonas (P = 0.104). Faecal coprostanol was also correlated with some discriminating bacteria of PE consumption. For example, bacterial groups that increased after consuming PE, such as Parabacteroides, positively correlated with faecal Uro-A and negatively correlated with coprostanol. In contrast, some bacteria that decreased after PE consumption, such as Sarcina, Anaerofustis, Coriobacteriaceae, and Coriobacteriia, positively correlated with coprostanol concentration (Fig. 4B).
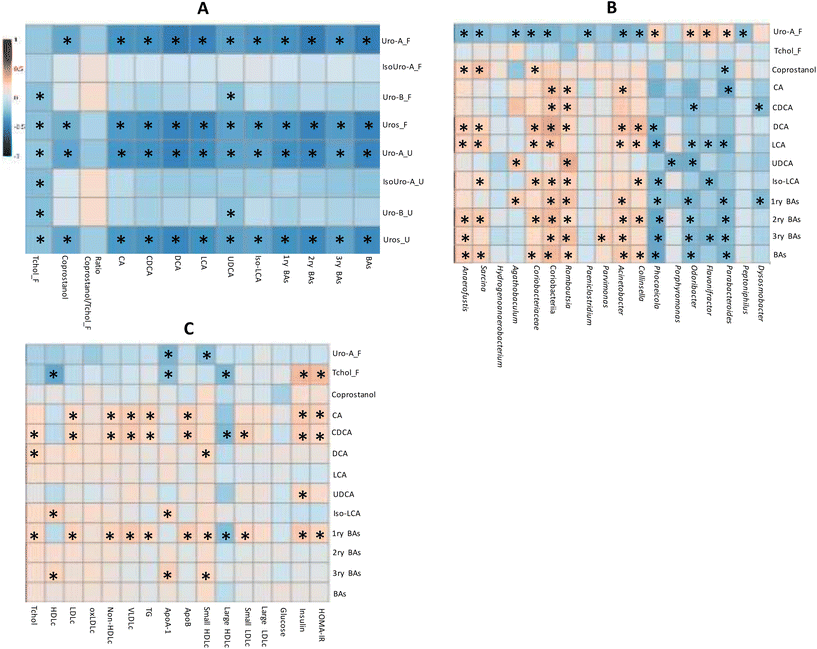 |
| Fig. 4 Spearman's correlation heat maps of faecal BAs and sterols with: (A) urolithins extracted from the faeces “F” and urine “U” and (B) discriminating bacteria of PE consumption and (C) serobiochemical variables. *: Spearman's correlation values with P < 0.05. | |
The serobiochemical variables10 correlated with some microbial metabolites, including those derived from BA metabolism. Thus, primary BAs positively correlated with serum Tchol, LDLc, non-HDLc, VLDLc, TG, Apo-B, small HDLc, small and large LDLc, insulin and HOMA-IR (Fig. 4C). Accordingly, CA and CDCA (primary BAs) were also positively associated with most of these serobiochemical variables. Some secondary BAs, such as DCA, also showed positive correlations with Tchol and small HDLc. Tertiary BAs positively correlated with HDLc, ApoB, and small HDLc. Other microbial metabolites, such as coprostanol, did not correlate with these serobiochemical variables.
Microbial faecal Uro-A was also not correlated with most of them (Fig. 4C). In contrast, faecal cholesterol was negatively associated with HDLc, ApoA, and large HDLc and positively associated with insulin and HOMA-IR.
Discussion
The gut microbiota, cholesterol, BAs, and health status are closely integrated and influence each other, making it difficult to ascertain whether gut dysbiosis and modified BA pools are a cause or consequence of disease.20 The gut microbial conversion of gut cholesterol releases coprostanol as the main metabolite.12 The primary BAs, CA and CDCA, are synthesised from cholesterol in the liver and stored in the gallbladder (conjugated with the amino acids glycine and taurine). Later, they are excreted into the duodenum to facilitate the digestion and absorption of lipophilic compounds, including dietary lipids, fat-soluble vitamins, and cholesterol.14 Most conjugated primary BAs are reabsorbed in the ileum except for a small fraction, deconjugated by bile salt hydrolase produced by different intestinal BA-deconjugating bacteria, which release deconjugated primary BAs. This deconjugated BA fraction reaches the colon, where CA and CDCA are dehydroxylated by the intestinal BA-metabolising bacteria into DCA and lithocholic acid LCA, respectively.5,13,14 These secondary BAs can be further transformed until tertiary BAs. UDCA, the most common tertiary bile acid, is produced from CDCA via 7-keto-CDCA, whereas iso-LCA is produced from CDCA via LCA.1
When addressing the possible effect of some dietary polyphenols, such as ETs, on BAs and cholesterol metabolism, the complexity is further increased due to the inter-individual differences in ETs and the host's steroid metabolism influenced by the gut microbiota of each individual. Indeed, three main UMs upon ET metabolism have been identified in the population by a qualitative criterion (i.e., producers vs. non-producers of specific Uros), but within Uro producers (UM-A or UM-B) also exist a Uro production gradient that gives rise to higher and lower Uro producers.2,21 To date, the effects of ET-rich products, including pomegranate, on the microbial transformation of colonic BAs and cholesterol have not been explored in depth in humans, and there is no information about the role of Uros, UMs and their associated gut microbiota in these effects.
In the present study, primary BAs and cholesterol concentration in the faeces did not differ among UMs at baseline. In contrast, UM-B presented the highest levels of total BAs, secondary BAs and coprostanol, which were statistically higher than those of UM-0 but not those of UM-A, which showed intermediate mean levels compared to the other two UMs. Therefore, lipid absorption capacity, microbial BA metabolism, and gut cytotoxic risk could be augmented in UM-B overweight-obese subjects, especially compared to UM-0, because gut BAs promote absorption of lipids, including cholesterol, by acting as emulsifiers. Moreover, secondary BAs are considered cytotoxic, resulting in an increased risk of gut inflammation, gut permeability and colon cancer.12 Interestingly, differences in the human gut microbial ecologies associated with UMs have been identified, and current evidence suggests that the UM-B group could be potentially prone to gut dysbiosis.2,9–11,18,21,22 As previously reported,10 the UM-B individuals of the present study were also at higher cardiovascular risk since their serum Tchol, LDLc, VLDLc, oxLDLc, ApoB, non-HDLc, IDLc, and small and large LDLc levels were higher than those in the UM-0 and UM-A groups at the baseline. The UM-A group showed intermediate mean levels compared to the other two UM groups.
Faecal and urinary Uro excretion by PE consumption was seen in Uro producers (UM-A or UM-B) with qualitative and quantitative differences.10 Accordingly, in the present study, we show the inter-individual differences in Uro-A production in both Uro-producing metabotypes (UM-A and UM-B) after 3-week intake of four daily capsules of PE (Fig. 1). As previously reported,10 urolithin concentration is dose-dependently increased (around 3.5-fold) after increasing consumption from one to four PE capsules. Also, three Uro non-producers (UM-0) became producers following PE consumption. Notably, PE improved some serum cardiovascular risk markers, but only in UM-B subjects.10 As previously described, the most significant quantitative inverse correlations between urinary Uros and cardiovascular markers were observed in UM-B individuals, i.e., Tchol, LDLc, and non-HDLc negatively correlated with urinary Uro-A excretion. In contrast, LDLc also correlated with urinary IsoUro-A and Uro-B.10 However, no significant correlations were found between faecal Uro excretion and most cardiovascular risk markers. These results suggest circulating Uros in plasma could be responsible for the beneficial effects on cardiovascular risk markers and agree with previous studies in different rodent models mimicking cardiometabolic disorders, which described positive effects in serobiochemical parameters after oral and intraperitoneal administration of Uros as reviewed before.2 Regarding the evidence of the cardioprotective activities of Uros in vitro, several studies reported Uro activity on cardiomyocytes, macrophages, hepatocytes, and adipocytes, as reviewed elsewhere.2 Uros also significantly decreased serum and hepatic cholesterol, suggesting the hypolipidemic effect of Uro-A and Uro-B in rats.23 In that study, Uro-A and Uro-B treatments downregulated the expression of the liver X receptors (LXRα) and sterol regulatory element binding protein 1c (SREBP1c), involved in de novo lipogenesis.23 However, whether Uros could also exert their cardiovascular and intestinal protective effects indirectly through modulating the gut microbiota involved in transforming host metabolites such as BAs and cholesterol has not been explored.
The present study showed that PE intake in overweight-obese volunteers significantly reduced the faecal BA pool (deconjugated-primary BAs, secondary and tertiary BAs) and coprostanol in all volunteers before clustering and in the Uro-producing metabotype subgroups (UM-A and UM-B), unlike UM-0. Although the total BA concentrations in the UM-A and UM-B groups were similar after PE consumption, the decrease was quantitatively more remarkable in the UM-B than in UM-A individuals, since UM-B started from higher concentrations. These total BA reductions were mainly due to the larger quantitative reduction of secondary BAs in the UM-B vs. UM-A subjects since the decrease of primary BAs was similar in both UMs (Fig. 2). Accordingly, the reduction of DCA and LCA (secondary BAs) was quantitatively more relevant in the UM-B subjects vs. the UM-A subjects, whereas CA and CDCA (primary BAs) were similarly reduced in the UM-A and UM-B groups (Fig. 2). In the UM-0 group (n = 6), these reductions were also observed in three (50%) of them who became Uro-A producers after PE consumption (data not shown). Therefore, these reductions were not significant after PE intake in the UM-0 group, which showed the lowest baseline BA and coprostanol concentrations. Consequently, differences among the three UMs in the BA concentration disappeared after the PE intervention (Fig. 2). However, coprostanol levels were still higher in the UM-B group than in the UM-0 group after the PE intervention for 3 weeks (Fig. 2). The lack of effects in the UM-0 group could be due to only three of them becoming Uro-A producers after PE consumption.10 According to this, when all the volunteers were considered (n = 49), we observed a quantitative inverse correlation of Uro-A (a common metabolite of UM-A and UM-B) with the faecal BA pool (primary, secondary and tertiary BAs) and coprostanol. A previous study in nasutin and Uro-A-producing rats showed that a high-fat diet significantly increased the concentration of BAs in the liver and cecum. However, these high levels of BAs were reduced after supplementing the diets with ET-rich raspberry pomace.24 The authors observed that this supplementation markedly increased the expression levels of small heterodimer partner 1 (SHP-1) associated with farnesoid-X-receptor (FXR) and reduced the synthesis of BAs in the liver, thereby reducing the gut BAs.24 This aligns with the reduction of primary BAs by PE intake observed in the present study. In addition to the reduction in the hepatic production of primary BAs, other studies in animal models have also proved that dietary phenolic compounds can impact the microbial metabolism of gut BAs and cholesterol. For instance, rats showed lower levels of faecal secondary BAs after administering tea polyphenols and gallotannins, and apple, grape, and red beet juices, as well as curcumin, caffeic acid, catechin, rutin, and ellagic acid, as reviewed previously.12 However, a previous study reported no reduction in primary to secondary BA conversion, while cholesterol metabolism to coprostanol was significantly reduced in healthy humans after consuming pomegranate juice for four weeks.25 Regarding that study,25 the marginal changes observed in BA metabolism by pomegranate juice intake could be due to the presence of 25% UM-0 since no clustering by UMs nor quantitative correlations with Uros excretion was approached, and all individuals were considered a single group. This rationale aligns with previous studies that propose individual stratification according to the polyphenol metabolism capacity of their gut microbiota to understand individuals’ response to dietary polyphenols.26,27
We also observed a modulation of the gut microbiota abundance by PE in all the volunteers before clustering and in the Uro-producing metabotype subgroups (UM-A and UM-B). PE consumption decreased the abundance of Romboutsia, a BA-deconjugating bacteria involved in the amino acid hydrolysis process from conjugated primary BAs, as well as the BA-metabolising bacteria involved in primary BA metabolism to secondary and tertiary BAs such as Sarcina, Acinetobacter, Collinsella and the family Coriobacteriaceae.3,28,29 Although microbial modulation by PE was not identical in the UM-A and UM-B groups, some microbial genera involved in BA metabolism were reduced in both the groups. In the UM-0 group (n = 6), the lack of gut microbiota modulation could be due to only three volunteers becoming Uro-A producers after PE consumption. Accordingly, when all the volunteers were considered (n = 49), the relative abundance of these bacteria also correlated negatively with the faecal Uro-A levels and positively with BAs, especially secondary and tertiary BAs. According to this, a previous study in a colitis rat model showed that Uro-A oral administration decreased inflammation markers and modulated the gut microbiota favourably.30 Another previous study in hamsters has shown that the prevalence of Coriobacteriaceae is dependent on host genotype. Indeed, Coriobacteriaceae has been positively correlated with cholesterol absorption, free cholesterol in the liver, plasma non-HDLc, total cholesterol, liver weight, and white adipose tissue mass.31 Furthermore, this family has been associated with susceptibility to carcinomas and tumour development in a murine model32 and with UM-B in humans.9 PE consumption also increased some bacterial groups, including the well-known butyrate-producing bacteria (Odoribacter and Dysosmobacter) or propionate-producing genera (Phocaeicola),33 which were positively associated with the faecal Uro-A levels. Interestingly, Dysosmobacter welbionis has been reported to prevent diet-induced obesity and metabolic disorders in mice.34 Similarly, a previous study conducted with rats showed that Uro-A and Uro-B intake improved liver and kidney functions and modulated the gut microbiota. In particular, Uro-A decreased species diversity and microbial richness and negatively impacted the composition of pathogenic microbes in normal rats.35 Overall, our results suggest that Uro-A (shared microbial metabolite between UM-A and UM-B) is the main driver of the PE effects on the gut microbiota, and it is likely to impact BA profiles in the host, reducing gut cytotoxicity and with potential consequences for lipid metabolism and signalling. The precise role of coprostanol (also negatively correlated with Uro-A production) in the pathogenesis of dyslipidemia remains elusive. It deserves further research to elucidate whether coprostanol is a biomarker that can also influence the pathogenesis of cardiometabolic diseases and dyslipidemia.36 Additionally, more research is needed to confirm the impact of Uros intake on the host's health and safety before being considered a possible cardiovascular and gut cytotoxic protector molecule.
Conclusions
Although the lipid-lowering effect of ET-rich foods in cardiometabolic diseases has been previously reported, it is unknown whether ETs and (or) their microbial metabolites Uros exert direct cardioprotective effects in humans and (or) indirectly through modulating the composition and functionality of the gut microbiota, especially those microbes involved in BA and cholesterol metabolism. The total number of microbial species and families engaged in host cholesterol and BA transformations, their metabolic pathways, and the roles that cholesterol and BA microbial metabolism play in host health continue to be elucidated. These aspects are often underappreciated as contributors to sterol-related diseases. Our results show that PE intake reduces gut microbial metabolism of BAs and cholesterol and modulates the gut microbiota in an Uro-A concentration-dependent manner. This suggests that Uro-A could not only exert direct cardioprotective effects, but also indirectly affect through modulating the composition and functionality of the gut microbiota, including the reduction of Coriobacteriaceae family and BA pool, which promote gut absorption of lipids. The role that some polyphenols and derived microbial metabolites, such as Uros, may exert in the gut microbial ecology associated with steroid metabolism highlights the need for further research to understand the complex interplay between dietary polyphenols, host BAs and sterols, the gut microbiota and mechanisms in the host that regulate serum cholesterol levels.
Author contributions
Conceptualization and design: M. V. S. Experiment execution: A. C. M., C. E. I., A. M., and M. R. V. Methodology and software: A. C. M., C. E. I., A. M., F. V., M. R. V., and M. V. S. Writing: M. V. S. Critical review of the manuscript for important intellectual content: J. C. E., A. C. M., and C. E. I. All authors have read and approved the final manuscript.
Conflicts of interest
There are no conflicts to declare.
Acknowledgements
This work has been funded by the Projects 20880/PI/18 (Fundación Séneca de la Región de Murcia, Spain), PID2022-136419OB-100 and AGROALNEXT programme by the Ministry of Science and Innovation (MICIN, AEI/10.13039/501100011033, and PRTR-C17.I1, Spain) with funding from “ERDF A way of making Europe”, the European Union NextGenerationEU (PRTR-C17.I1) and by Fundación Séneca with funding from Comunidad Autónoma Región de Murcia (CARM). C.E.I.-A. holds a predoctoral grant from MICIN (grant number FPU18/03961).
References
- I. Rowland, G. Gibson, A. Heinken, K. Scott, J. Swann, I. Thiele, K. Tuohy and I. Rowland, Gut microbiota functions: metabolism of nutrients and other food components, Eur. J. Nutr., 2018, 57, 1–24 CrossRef CAS PubMed.
- R. García-Villalba, J. A. Giménez-Bastida, A. Cortés-Martín, M. Á. Ávila-Gálvez, F. A. Tomás-Barberán, M. V. Selma, J. C. Espín and A. González-Sarrías, Urolithins: a comprehensive update on their metabolism, bioactivity, and associated gut microbiota, Mol. Nutr. Food Res., 2022, 2101019 CrossRef PubMed.
- D. V. Guzior and R. A. Quinn, Review: microbial transformations of human bile acids, Microbiome, 2021, 9, 1–13 CrossRef PubMed.
- N. Molinero, L. Ruiz, B. Sánchez, A. Margolles and S. Delgado, Intestinal Bacteria Interplay With Bile and Cholesterol Metabolism: Implications on Host Physiology, Front. Physiol., 2019, 10, 185 CrossRef PubMed.
- A. Kriaa, M. Bourgin, A. Potiron, H. Mkaouar, A. Jablaoui, P. Gérard, E. Maguin and M. Rhimi, Microbial impact on cholesterol and bile acid metabolism: current status and future prospects, J. Lipid Res., 2019, 60, 323–332 CrossRef CAS PubMed .J. I.
-
M. V. Selma, F. A. Tomás-Barberán, M. Romo-Vaquero, A. Cortés-Martín and J. C. Espín, in Dietary Polyphenols: Metabolism and Health Effects, ed. R. García-Villaba. F. A. Tomás-Barberán and A. González-Sarrías, Wiley, Oxford, UK, 2020, ch. 13, pp. 497–531 Search PubMed.
- M. V. Selma, J. C. Espín and F. A. Tomás-Barberán, Interaction between Phenolics and Gut Microbiota: Role in Human Health, J. Agric. Food Chem., 2009, 57, 6485–6501 CrossRef CAS PubMed.
- R. García-Villalba, F. A. Tomás-Barberán, C. E. Iglesias-Aguirre, J. A. Giménez-Bastida, A. González-Sarrías, M. V. Selma and J. C. Espín, Ellagitannins, urolithins, and neuroprotection: Human evidence and the possible link to the gut microbiota, Mol. Aspects Med., 2023, 89, 101109 CrossRef PubMed.
- M. Romo-Vaquero, A. Cortés-Martín, V. Loria-Kohen, A. Ramírez-de-Molina, I. García-Mantrana, M. C. Collado, J. C. Espín and M. V. Selma, Deciphering the Human Gut Microbiome of Urolithin Metabotypes: Association with Enterotypes and Potential Cardiometabolic Health Implications, Mol. Nutr. Food Res., 2019, 63, 1800958 CrossRef PubMed.
- A. González-Sarrías, R. García-Villalba, M. Romo-Vaquero, C. Alasalvar, A. Örem, P. Zafrilla, F. A. Tomás-Barberán, M. V. Selma and J. C. Espín, Clustering according to urolithin metabotype explains the interindividual variability in the improvement of cardiovascular risk biomarkers in overweight-obese individuals consuming pomegranate: A randomised clinical trial, Mol. Nutr. Food Res., 2017, 61, 1600830 CrossRef PubMed.
- M. V. Selma, A. González-Sarrías, J. Salas-Salvadó, C. Andrés-Lacueva, C. Alasalvar, A. Örem, F. A. Tomás-Barberán and J. C. Espín, The gut microbiota metabolism of pomegranate or walnut ellagitannins yields two urolithin-metabotypes that correlate with cardiometabolic risk biomarkers: Comparison between normoweight, overweight-obesity and metabolic syndrome, Clin. Nutr., 2018, 37, 897–905 CrossRef CAS PubMed .5C.
- J. I. Mosele, A. Macià and M.-J. Motilva, Metabolic and microbial modulation of the large intestine ecosystem by non-absorbed diet phenolic compounds: A review, Molecules, 2015, 20, 17429–17468 CrossRef CAS PubMed.
- S. L. Collins, J. G. Stine, J. E. Bisanz, C. D. Okafor and A. D. Patterson, Bile acids and the gut microbiota: metabolic interactions and impacts on disease, Nat. Rev. Microbiol., 2023, 21, 236–247 CrossRef CAS PubMed.
- C. Callender, I. Attaye and M. Nieuwdorp, The Interaction between the Gut Microbiome and Bile Acids in Cardiometabolic Diseases, Metabolites, 2022, 12, 65 CrossRef CAS PubMed.
- M. Romo-Vaquero, R. García-Villalba, A. González-Sarrías, D. Beltrán, F. A. Tomás-Barberán, J. C. Espín and M. V. Selma, Interindividual variability in the human metabolism of ellagic acid: Contribution of Gordonibacter to urolithin production, J. Funct. Foods, 2015, 17, 785–791 CrossRef CAS.
- S. Keller and G. Jahreis, Determination of underivatised sterols and bile acid trimethyl silyl ether methyl esters by gas chromatography-mass spectrometry-single ion monitoring in faeces, J. Chromatogr. B: Anal. Technol. Biomed. Life Sci., 2004, 813, 199–207 CrossRef CAS PubMed.
- G. Kakiyama, A. Muto, H. Takei, H. Nittono, T. Murai, T. Kurosawa, A. F. Hofmann, W. M. Pandak and J. S. Bajaj, A simple and accurate HPLC method for fecal bile acid profile in healthy and cirrhotic subjects : validation by GC-MS and LC-MS, J. Lipid Res., 2014, 55, 978–990 CrossRef CAS PubMed.
- A. Cortés-Martín, M. Romo-Vaquero, I. García-Mantrana, A. Rodríguez-Varela, M. C. Collado, J. C. Espín and M. V. Selma, Urolithin Metabotypes can Anticipate the Different Restoration of the Gut Microbiota and Anthropometric Profiles during the First Year Postpartum, Nutrients, 2019, 11, 2079 CrossRef PubMed.
- A. González-Sarrías, M. Romo-Vaquero, R. García-Villalba, A. Cortés-Martín, M. V. Selma and J. C. Espín, The Endotoxemia Marker Lipopolysaccharide-Binding Protein is Reduced in Overweight-Obese Subjects Consuming Pomegranate Extract by Modulating the Gut Microbiota: A Randomized Clinical Trial, Mol. Nutr. Food Res., 2018, 4, 1800160 CrossRef PubMed.
- N. M. Sagar, I. A. Cree, J. A. Covington and R. P. Arasaradnam, The Interplay of the Gut Microbiome, Bile Acids, and Volatile Organic Compounds, Gastroenterol. Res. Pract., 2015, 2015, 10–15 Search PubMed.
- C. E. Iglesias-Aguirre, M. Romo-vaquero, M. V. Selma and J. C. Espín, Unveiling metabotype clustering in resveratrol, daidzein, and ellagic acid metabolism: Prevalence, associated gut microbiomes, and their distinctive microbial networks, Food Res. Int., 2023, 173, 113470 CrossRef PubMed.
- I. García-Mantrana, M. Calatayud, M. Romo-Vaquero, J. C. Espín, M. V. Selma and M. C. Collado, Urolithin metabotypes can determine the modulation of gut microbiota in healthy individuals by tracking walnuts consumption over three days, Nutrients, 2019, 11, 1–13 CrossRef PubMed.
- A. O. Abdulrahman, A. Kuerban, Z. A. Alshehri, W. H. Abdulaal, J. A. Khan and M. I. Khan, Urolithins attenuate multiple symptoms of obesity in rats fed on a high-fat diet, Diabetes, Metab. Syndr. Obes., 2020, 13, 3337–3348 CrossRef CAS PubMed.
- B. Fotschki, J. Juśkiewicz, A. Jurgoński, N. Rigby, M. Sójka, K. Kołodziejczyk, A. Mackie and Z. Zduńczyk, Raspberry pomace alters cecal microbial activity and reduces secondary bile acids in rats fed a high-fat diet, J. Nutr. Biochem., 2017, 46, 13–20 CrossRef CAS PubMed.
- J. I. Mosele, M.-J. Gosalbes, A. Macià, L. Rubio, J. F. Vázquez-Castellanos, N. Jiménez-Hernández, A. Moya, A. Latorre and M.-J. Motilva, Effect of daily intake of pomegranate juice on fecal microbiota and feces metabolites, Mol. Nutr. Food Res., 2015, 59, 1942–1953 CrossRef CAS.
- C. E. Iglesias-Aguirre, A. Cortés-Martín, M. Á. Ávila-Gálvez, J. A. Gimenez-Bastida, M. V. Selma, A. González-Sarrías and J. C. Espin, Main drivers of (poly)phenols effects on human health: metabolites production and(or) gut microbiota-associated metabotypes?, Food Funct., 2021, 12, 10324–10355 RSC.
- A. Cortés-Martín, M. V. Selma, F. A. Tomás-Barberán, A. González-Sarrías and J. C. Espín, Where to Look into the Puzzle of Polyphenols and Health? The Postbiotics and Gut Microbiota Associated with Human Metabotypes, Mol. Nutr. Food Res., 2020, 64, 1900952 CrossRef PubMed.
- V. Aries, J. S. Crowther, B. S. Drasar and M. J. Hill, Degradation of bile salts
by human intestinal bacteria., Gut, 1969, 10, 575–576 CrossRef CAS PubMed.
- D. D. M. Grigorescu and D. Serban, Metabolism of Bile Acids by Strains of Acinetobacter Anitratum and Acinetobacter Lwoffii, Am. J. Gastroenterol., 1978, 69, 450–452 Search PubMed.
- M. Larrosa, A. González-Sarrías, M. J. Yáñez-Gascón, M. V. Selma, M. Azorín-Ortuño, S. Toti, F. Tomás-Barberán, P. Dolara and J. C. Espín, Anti-inflammatory properties of a pomegranate extract and its metabolite urolithin-A in a colitis rat model and the effect of colon inflammation on phenolic metabolism., J. Nutr. Biochem., 2010, 21, 717–725 CrossRef CAS PubMed.
- I. Martínez, D. J. Perdicaro, A. W. Brown, S. Hammons, T. J. Carden, T. P. Carr, K. M. Eskridge and J. Walter, Diet-induced alterations of host cholesterol metabolism are likely to affect the gut microbiota composition in hamsters, Appl. Environ. Microbiol., 2013, 79, 516–524 CrossRef.
- A. K. Benson, S. A. Kelly, R. Legge, F. Ma, S. J. Low, J. Kim, M. Zhang, P. L. Oh, D. Nehrenberg, K. Hua, S. D. Kachman, E. N. Moriyama, J. Walter, D. A. Peterson and D. Pomp, Individuality in gut microbiota composition is a complex polygenic trait shaped by multiple environmental and host genetic factors, Proc. Natl. Acad. Sci., 2010, 107, 18933–18938 CrossRef CAS PubMed.
- R. Lück and U. Deppenmeier, Genetic tools for the redirection of the central carbon flow towards the production of lactate in the human gut bacterium Phocaeicola (Bacteroides) vulgatus, Appl. Microbiol. Biotechnol., 2022, 106, 1211–1225 CrossRef.
- T. L. Roy, E. M. De Hase, M. V. Hul, A. Paquot, R. Pelicaen, M. Régnier, C. Depommier, C. Druart, A. Everard, D. Maiter, N. M. Delzenne, L. B. Bindels, M. De Barsy, A. Loumaye, M. P. Hermans, J. P. Thissen, S. Vieira-Silva, G. Falony, J. Raes, G. G. Muccioli and P. D. Cani,
Dysosmobacter welbionis is a newly isolated human commensal bacterium preventing diet-induced obesity and metabolic disorders in mice, Gut, 2022, 71, 534–543 CrossRef PubMed.
- A. K. A. Khalaf, A. O. Abdulrahman, M. Kaleem, S. M. Nur, A. H. Asseri, H. Choudhry and M. I. Khan, Comparative analysis of the impact of urolithins on the composition of the gut microbiota in normal-diet fed rats, Nutrients, 2021, 13, 3885 CrossRef PubMed.
- C. Juste and P. Gérard, Cholesterol-to-coprostanol conversion by the gut microbiota: What we know, suspect, and ignore, Microorganisms, 2021, 9, 1881 CrossRef CAS PubMed.
Footnote |
† These authors contributed equally to this work. |
|
This journal is © The Royal Society of Chemistry 2024 |
Click here to see how this site uses Cookies. View our privacy policy here.