DOI:
10.1039/D3GC04436J
(Communication)
Green Chem., 2024,
26, 11140-11146
Reusable Co-catalysts for general and selective α-alkylation of nitriles with alcohols†
Received
15th November 2023
, Accepted 16th October 2024
First published on 21st October 2024
Abstract
A general cobalt-catalyzed α-alkylation of nitriles with alcohols is reported. Utilizing this straightforward borrowing hydrogen methodology, a series of substituted and functionalized nitriles can be easily coupled with benzylic, heterocyclic, and aliphatic alcohols to prepare diverse functionalized nitriles in good to excellent yields (>70 examples). Key for this synthesis is the use of specific cobalt-nanoparticles supported on N-doped carbon, which were conveniently prepared by pyrolysis of a templated material generated in situ by mixing cobalt chloride, zinc chloride, D-glucosamine hydrochloride, and colloidal silica, and subsequent removal of the silica.
Introduction
The construction of carbon–carbon bonds is of central importance in organic synthesis. Among the many known methods, base- or metal-catalyzed alkylations of carbonyl compounds continue to be of major interest. In this respect, nitriles also represent valuable starting materials, which can be readily transformed into a plethora of amines, carboxylic acids, amides, acetamidines, ketones, N-heterocycles, and so on (Scheme 1).1–3 In addition, nitrile moieties constitute integral parts of several drugs and biomolecules.4–7 Because of their importance, synthetic methods for their preparation are continuously improved. Traditionally, stoichiometric nucleophilic substitution reactions with (over)stoichiometric amounts of metal hydrides prevailed in this area.8,9 However, these processes have environmental drawbacks due to the inevitable generation of large amounts of waste.
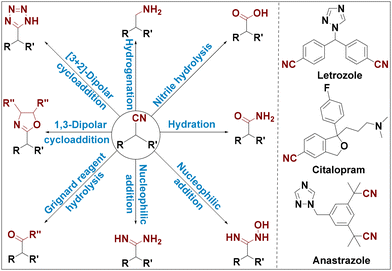 |
| Scheme 1 Various synthetic applications of nitriles and selected nitrile-containing drugs. | |
Clearly, α-alkylation of nitriles with alcohols via the so-called borrowing hydrogen (BH) or hydrogen auto-transfer methodologies offers more sustainable approaches. Notably, the utilization of alcohols as starting materials allows the application of bio-based feedstocks and there is no need for any external/additional hydrogen as the alcohol substrate serves as the hydrogen donor. The general mechanism for the alkylation of nitriles with alcohols is shown in Scheme 2 (bottom). First the alcohol undergoes catalytic dehydrogenation to give the corresponding aldehyde, which is then condensed with the nitrile to form the respective α,β-unsaturated nitrile as an intermediate. Finally, this intermediate is reduced in the presence of the catalyst to produce the desired alkylated nitrile. Consequently, this method features high step- and atom-economy as water is the only by-product.
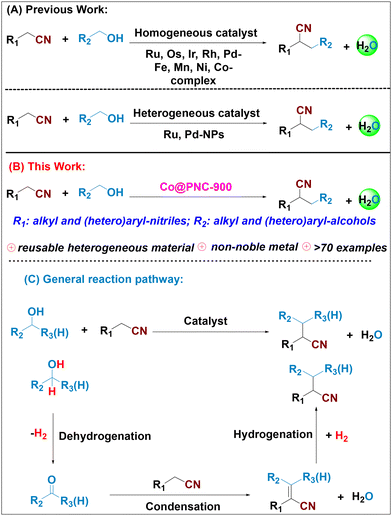 |
| Scheme 2 State-of-the-art transition-metal-catalysed α-alkylation of nitriles with alcohols. (A) Previous works, (B) this work, and (C) general reaction pathway. | |
Until now, a variety of molecularly-defined catalysts have been successfully developed for catalytic α-alkylation of nitriles with alcohols.10–27 Pioneering work was reported by Grigg and co-workers utilizing ruthenium catalysts for α-alkylation of acetonitrile in 1981.10 Afterwards, Ir,11–14 Rh,15–17 Ru,13,18–21 and Os22 based catalytic protocols were developed for this transformation. Mainly in the past decade, homogeneous non-noble metal complexes based on Fe,23 Mn,3,24 Ni25 and Co26,27 were also reported to be effective in the α-alkylation of nitriles.
An ideal catalyst system for nitrile alkylation should be environmentally benign, inexpensive, generally applicable, stable, and easily recyclable. In this respect, especially the development of heterogeneous catalysts is desirable, although surprisingly few materials are known. In 2004, Kaneda and co-workers developed the first heterogeneous ruthenium supported hydrotalcite catalyst, which required prolonged heating at high temperature (180 °C, 20–30 h) and a large excess of alcohols as alkylating reagents.28 Since then, only two other materials based on palladium and ruthenium have been introduced for such transformations.29,30 Indeed, to the best of our knowledge, no heterogeneous non-noble metal catalyst is known so far to promote nitrile alkylation via hydrogen borrowing methodologies (Scheme 2).
In recent years, many research groups including ours have explored metal complexes with nitrogen-containing ligands and metal–organic frameworks (MOFs) with different linkers as suitable precursors for the preparation of supported metal nanoparticles.31–45 Utilizing controlled pyrolysis conditions, well-dispersed metal–nitrogen–carbon catalysts are formed. The resulting materials were successfully applied for many reactions such as oxidations,41,45 reductive aminations,39,46 and hydrogenation and related transformations.31,38,43,44 Notably, the synthesis of such doped-carbonaceous materials is also possible from sustainable resources. Following this approach, abundantly available cellulose, chitin, and glucose constitute ideal candidates for the development of new catalysts.47–50
Herein, we report the synthesis, characterization, and application of novel supported cobalt-nanoparticles for the preparation of functionalized nitriles. The optimal catalyst was prepared by pyrolysis of a templated material obtained in situ from a reaction mixture of cobalt and zinc salts, glucosamine, and commercially available colloidal silica (LUDOX® HS-40; 40 wt% suspension in H2O) and subsequent removal of the silica content.
Results and discussion
Preparation and catalytic evaluation of Co-nanoparticles
At the start of this project, we synthesized different Co–N–C materials by mixing CoCl2·6H2O with D-glucosamine hydrochloride and colloidal silica. To obtain hierarchically porous materials, ZnCl2 was also added which is known to create porous nanostructures.50,51 After pyrolysis of the catalyst precursors under argon at 800–1000 °C, the formed silica was removed with 5 M NH4HF2 solution (Fig. 1). The details of the synthetic procedure are described in the ESI.† The resulting cobalt materials are represented as Co@PNC-T, where PNC and T denote porous N-doped carbon and the pyrolysis temperature, respectively.
 |
| Fig. 1 Preparation of cobalt nanoparticles supported on porous N-doped carbon. | |
The activities and selectivities of these Co-materials (Co@NC-T) were initially evaluated for the N-alkylation of phenylacetonitrile 1 with benzyl alcohol 2 to prepare 2,3-diphenylpropanenitrile 3 in the presence of 1 equivalent of K3PO4 at 140 °C for 24 h in toluene solvent (Table 1).
Table 1 α-Alkylation of phenylacetonitrile with benzyl alcohol: testing of cobalt catalysts

|
Entry |
Catalyst |
Conv.1 (%) |
Yield 3 (%) |
Yield 3a (%) |
Reaction conditions: 0.5 mmol phenylacetonitrile, 1 mmol benzyl alcohol, 50 mg catalyst (2.04 mol% Co), 0.5 mmol K3PO4 (1 equiv.), 2 mL toluene, 140 °C, 24 h. Conversions and yields are based on phenylacetonitrile and determined by GC using n-hexadecane as a standard. In homogeneous catalytic reactions, 10 mol% of cobalt nitrate and 30 mol% of ligand were used. Without a base. Without a catalyst and in the presence of 0.5 mmol K3PO4 (1 equiv.). |
1 |
CO@PNC-900 |
99 |
95 |
2 |
2 |
Co@NC-900 (without zinc) |
88 |
71 |
10 |
3 |
Co@PNC-800 |
87 |
74 |
9 |
4 |
Co@PNC-1000 |
82 |
72 |
7 |
5 |
Porous N-doped carbon (without cobalt) |
<5 |
<5 |
— |
6 |
Co-particles-900 (without a ligand) |
61 |
43 |
15 |
7 |
Co@NC-900-SiO2 (remaining SiO2) |
57 |
42 |
11 |
8 |
CoZn-L-SiO2 (unpyrolyzed) |
<5 |
<5 |
— |
9 |
Co(NO3)2·6H2O |
<5 |
<5 |
— |
10 |
Zn(NO3)2·6H2O |
<5 |
<5 |
— |
11 |
Co(NO3)2-L |
<5 |
<5 |
— |
12a |
Co@NC-900-1 (without a base) |
68 |
57 |
10 |
13b |
0.5 mmol K3PO4 (without a catalyst) |
<5 |
<5 |
— |
Among all the prepared materials, Co@PNC-900 was found to be the best one and a quantitative yield of the desired product 3 was obtained (Table 1; entry 1). The material prepared using the cobalt salt and D-glucosamine hydrochloride, without the zinc salt as a precursor, showed lower activity and produced 71% of the desired product 3 (Table 1; entry 2), which indicates the beneficial effect of the porous material. Next, we tested the effect of different pyrolysis temperatures on the yield of product 3. Here, a pyrolysis temperature of 900 °C was the best one (Table 1; entries 1, 3 and 4). Notably, no zinc was detected by ICP-OES in these three samples. In addition, we also synthesised a porous carbon material without using the cobalt salt and tested its activity. As expected, only trace amounts of 3 were formed (Table 1; entry 5). Furthermore, the material prepared without D-glucosamine hydrochloride or the one with remaining silica was found to be less active and produced 43% and 30% of 3, respectively (Table 1; entries 6 and 7). Using related homogeneous catalysts and non-pyrolyzed materials also gave only traces of 3 (Table 1; entries 8–11). Interestingly, by applying Co@PNC-900, the model reaction was also promoted in the absence of a base and gave 57% of 2,3-diphenylpropanenitrile 3 (Table 1; entry 12). Finally, different solvents, bases, and other reaction parameters such as the temperature, amount of catalyst and reaction time were evaluated for the model reaction (Tables S2–S4; ESI†).
Characterization of Co-based materials
To further understand the structure of the catalytic active material, we performed X-ray powder diffraction (XRD), scanning transmission electron microscopy (STEM), and X-ray photoelectron spectroscopy (XPS) characterization studies. The X-ray diffraction pattern of Co@NC-900-SiO2 does not exhibit diffraction peaks which can be assigned to crystalline metallic or oxidic cobalt species. Instead, only amorphous silica is indicated by a broad peak around 22° 2theta (Fig. S1†). Even after the removal of SiO2 or recycling of the catalysts, no additional peaks can be found, indicating a high dispersion of metal species. However, distorted carbon might be the origin of wide peaks in the regions of 20–30° and 40–45° 2theta which can be interpreted as the partly graphitic-like structure (Fig. S2 and 3†). Next, STEM analysis of Co@PNC-900 showed the presence of highly porous carbon with sizes similar to those of mostly dissolved SiO2 particles (Fig. 2). In the carbon phase, small amounts of N, O, Si and Co could also be detected (Fig. S4†) without accompanying particles, indicating a highly distributed phase. Additionally, few rather large Co oxide particles and SiO2 particles could be identified as minority phases. Zn traces were not found, indicating that zinc was completely removed at 900 °C.
 |
| Fig. 2 STEM-HAADF (A and C) and STEM-ABF (B and D) images of the Co@PNC-900 catalyst showing an overview (A and B) and high-resolution details (C and D) of the highly porous carbon framework containing Co. | |
XPS analysis was used to further determine the surface elemental compositions and chemical state. XPS analysis revealed the composition of the surface, as shown in Table S1.† Besides carbon, nitrogen, and cobalt, there are also oxygen and traces of silica, probably originating from the support and/or the preparation process. In the C 1s spectra, five peaks are observed at ∼284.7, 289.1, 287.7, 288.9 and 291.1 eV, which are assigned to C–C, C–O, C
O, and C
O–C as well as satellite peaks, respectively (Fig. 3a).31 The O 1s spectra (Fig. 3b) showed three peaks at 531.7, 533.4, and 536.2 eV, which correspond to the C
O, C–O, and COO– bonds.31 The N 1s spectra (Fig. 3c) displayed five peaks with binding energies at 398.3, 399.8, 401.0, and 402.8 eV as well as 405.8 eV, which can be ascribed to pyridinic, pyrrolic, graphitic, and oxidized pyridinic N, respectively.52,53 The fifth peak at 405.8 eV only appears for the samples treated with NH4HF2 to remove the Si and might be originating from the nitrite groups (–NO2) remaining at the surface.54 In the cobalt region, the Co 2p3/2 peak is located at 780.4 eV and can be attributed to the Co2+ species55 with a pronounced satellite feature at 786.4 eV (Fig. 3d). For the recycled catalyst, Co@PNC-900-R, identical cobalt and nitrogen species could be observed with similar binding energies and oxidation states like in the case of the fresh catalyst, thus confirming the high stability of the catalyst (see Fig. S6† for comparison).
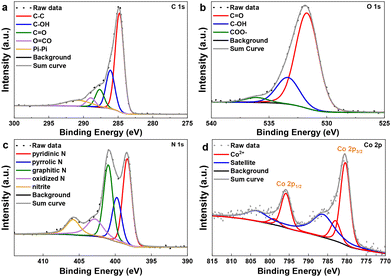 |
| Fig. 3 X-ray photoelectron spectra for Co@PNC-900. (a) C 1s, (b) O 1s, (c) N 1s and (d) Co 2p regions. | |
Stability, recycling, and reusability of the Co@PNC-900 catalyst
For any given heterogeneous catalyst, its stability and reusability are important aspects. To prove these features, we performed recycling experiments for the model reaction under two different reaction conditions (24 h, complete conversion and 5 h, around 50% conversion). As shown in Fig. 4, Co@PNC-900 exhibited high stability and was recycled and reused up to 7 times without significant deactivation.
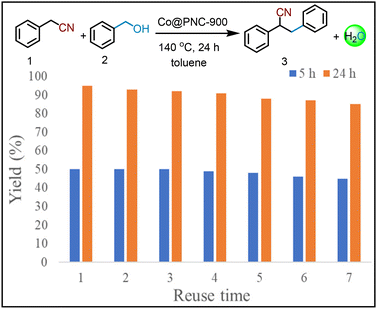 |
| Fig. 4 Stability and recycling of the Co@PNC-900 catalyst for the synthesis of 2,3-diphenylpropanenitrile. Reaction conditions: 1.5 mmol phenylacetonitrile, 3 mmol benzyl alcohol, 160 mg catalyst (2.17 mol% Co), 1.5 mmol K3PO4, 4 mL toluene, 140 °C, 5 h and 24 h. Conversions and yields are based on phenylacetonitrile and determined by GC using n-hexadecane as a standard. | |
α-Alkylation of phenylacetonitrile 2 with different alcohols
With the optimal catalyst (Co@PNC-900) and the optimal reaction conditions in hand, we explored its general applicability for the synthesis of functionalized nitrile products. First, we investigated the α-alkylation of 2 using various alcohols. As shown in Scheme 3, various benzylic, heterocyclic, and aliphatic primary alcohols reacted well with phenylacetonitrile and gave the corresponding α-alkylated nitriles in up to 86% yield (Scheme 3; products 4–10). Halide substituents (F-, Cl-, Br-) at the meta- or para-position of the phenyl ring were well tolerated (Scheme 3, products 11–15). Similarly, trifluoromethyl-, thioether-, ether-, nitrile-, and N,N-dimethyl group containing substrates gave the desired products in up to 88% yield (Scheme 3; products 16–21). Next, different heterocyclic alcohols containing O, S and N atoms, such as piperonyl alcohol, 1,4-benzodioxin-6-methanol, 2-thiophenemethanol, and furane-, pyridine-, and indole-based alcohols were coupled with 2 to provide the corresponding heterocyclic compounds in up to 85% yield (Scheme 3; products 22–29). In addition, multi-substituted alcohols smoothly reacted and produced the corresponding alkylated nitriles (Scheme 3, products 28 and 30–34).
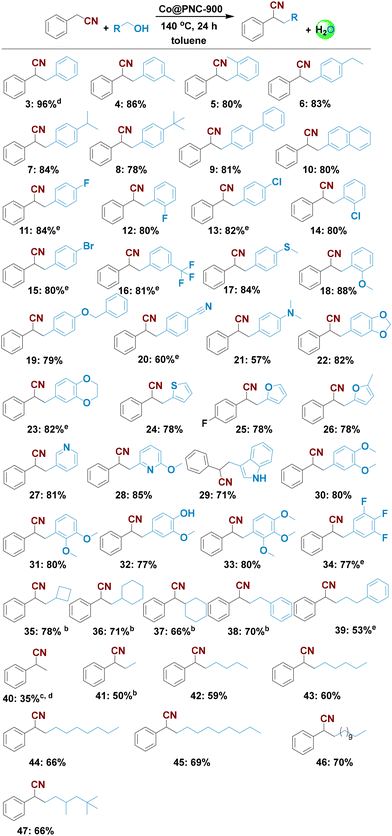 |
| Scheme 3 Co-nanoparticle-catalyzed α-alkylation of phenylacetonitrile with different alcohols.a a Reaction conditions: 0.5 mmol nitrile, 1 mmol alcohol, 50 mg catalyst (2.04 mol% Co), 0.5 mmol K3PO4 (1 equiv.), 2 mL toluene, 140 °C, 24 h, isolated yields. b The same as “a” with 80 mg catalyst (3.27 mol% Co) at 150 °C. c The same as “b” with 1 mmol KoMe. d GC yields obtained using n-hexadecane as a standard. e 1H NMR yields obtained using n-hexadecane as a standard. | |
Compared to benzylic alcohols, aliphatic alcohols are less reactive, and thus more difficult to activate.31 Nevertheless, our catalyst allowed for alkylation of such alcohols, albeit at higher temperature in the presence of 3.27 mol% catalyst (Scheme 3; products 35–47). Under these conditions, linear and cyclic aliphatic alcohols gave up to 78% of α-alkylated nitriles. Furthermore, we prepared the corresponding methylated products by simply utilizing methanol. Compared to traditional methylation reagents such as methyl iodide and dimethyl sulfate, methanol constitutes a more benign C1 source; however, it is particularly difficult to dehydrogenate.56 Nonetheless, starting from phenylacetonitrile 2 and methanol, selective monomethylation occurred to give nitrile 40 in 35% yield.
α-Alkylation of different nitriles with benzylic alcohols: scope of nitriles
Next, we explored the reactivity of different nitriles to prepare substituted and functionalized nitriles under standard conditions. Exemplarily, F-, Cl- and CF3-substituted as well as methoxy- and heterocyclic-containing substrates provided the corresponding products in good isolated yields up to 85% (Scheme 4; products 51–58). However, on performing the alkylation of five aliphatic nitriles as coupling partners, only small amounts of the desired products or no desired products were obtained (Scheme S1†).
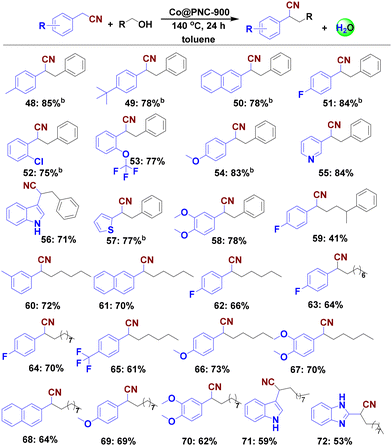 |
| Scheme 4 Co-nanoparticle-catalyzed α-alkylation of various nitriles with alcohols. Reaction conditions: 0.5 mmol nitrile, 1 mmol alcohol, 50 mg catalyst (2.04 mol% Co), 0.5 mmol K3PO4 (1 equiv.), 2 mL toluene, 140 °C, 24 h, isolated yields. a 1H NMR yields obtained using n-hexadecane as a standard. | |
In contrast, benzylic, araliphatic and aliphatic primary alcohols reacted well with different nitriles to give the desired α-alkylated products in up to 73% yield (Scheme 4, products 59–72). Finally, using glucose as an alkylating agent under the optimal conditions provided a complex reaction mixture.
Conclusions
In summary, we present the Co containing N-doped carbon matrix Co@PNC-900 as a novel heterogeneous catalyst, which allows for the general and selective synthesis of alkylated nitriles from alcohols by an atom-efficient borrowing hydrogen methodology. The optimal material is easily generated by mixing cobalt and zinc salts, D-glucosamine hydrochloride, and colloidal silica, followed by pyrolysis of the templated material, and subsequent removal of the silica. Functionalized and structurally diverse nitriles can be conveniently prepared starting from inexpensive and easily available alcohols and nitriles in the presence of Co@PNC-900.
Data availability
Data for this article, including compound characterization, calculations, and experimental data, are available as the ESI† at https://doi.org/10.1039/d3gc04436j.
Conflicts of interest
There are no conflicts to declare.
Acknowledgements
We gratefully acknowledge the Deutsche Forschungsgemeinschaft (DFG; Project number 447724917) and the State of Mecklenburg-Vorpommern for financial and general support. R. V. J. thanks the European Union under the REFRESH – Research Excellence for Region Sustainability and High-tech Industries project number CZ.10.03.01/00/22_003/0000048 via the Operational Programme Just Transition for general and financial support. Z. M. thanks the Chinese Scholarship Council (CSC) for a research fellowship. We thank the analytical team of the Leibniz-Institut für Katalyse e.V. for their excellent service.
References
- S. S. Kulp and M. J. McGee, J. Org. Chem., 1983, 48, 4097–4098 CrossRef CAS.
- V. G. Chandrashekhar, W. Baumann, M. Beller and R. V. Jagadeesh, Science, 2022, 376, 1433–1441 CrossRef CAS PubMed.
- A. Jana, C. B. Reddy and B. Maji, ACS Catal., 2018, 8, 9226–9231 CrossRef CAS.
- F. F. Fleming, L. Yao, P. Ravikumar, L. Funk and B. C. Shook, J. Med. Chem., 2010, 53, 7902–7917 CrossRef CAS PubMed.
- E. Pascual, F. Sivera, U. Yasothan and P. Kirkpatrick, Nat. Rev. Drug Discovery, 2009, 8, 191–193 CrossRef CAS PubMed.
- M. El-Kemary, J. A. Organero and A. Douhal, J. Med. Chem., 2006, 49, 3086–3091 CrossRef CAS PubMed.
- Y. C. Mu, T. T. Nguyen, M. J. Koh, R. R. Schrock and A. H. Hoveyda, Nat. Chem., 2019, 11, 478–487 CrossRef CAS PubMed.
- G. P. Ellis and T. M. Romney-Alexander, Chem. Rev., 1987, 87, 779–794 CrossRef CAS.
- R. Bacon and H. Hill, J. Chem. Soc., 1964, 1097–1107 RSC.
- R. Grigg, T. R. Mitchell, S. Sutthivaiyakit and N. Tongpenyai, Tetrahedron Lett., 1981, 22, 4107–4110 CrossRef CAS.
- C. Löfberg, R. Grigg, M. A. Whittaker, A. Keep and A. Derrick, J. Org. Chem., 2006, 71, 8023–8027 CrossRef PubMed.
- K. Taguchi, H. Nakagawa, T. Hirabayashi, S. Sakaguchi and Y. Ishii, J. Am. Chem. Soc., 2004, 126, 72–73 CrossRef CAS PubMed.
- G. Onodera, Y. Nishibayashi and S. Uemura, Angew. Chem., Int. Ed., 2006, 45, 3819–3822 CrossRef CAS PubMed.
- B. Anxionnat, D. Gomez Pardo, G. Ricci and J. Cossy, Org. Lett., 2011, 13, 4084–4087 CrossRef CAS PubMed.
- F. Li, X. Y. Zou and N. N. Wang, Adv. Synth. Catal., 2015, 357, 1405–1415 CrossRef CAS.
- B. W. H. Turnbull and P. A. Evans, J. Am. Chem. Soc., 2015, 137, 6156–6159 CrossRef CAS PubMed.
- J. J. Li, Y. X. Liu, W. J. Tang, D. Xue, C. Q. Li, J. L. Xiao and C. Wang, Chem. – Eur. J., 2017, 23, 14445–14449 CrossRef CAS PubMed.
- S. Thiyagarajan and C. Gunanathan, ACS Catal., 2017, 7, 5483–5490 CrossRef CAS.
- S. Burling, B. M. Paine, D. Nama, V. S. Brown, M. F. Mahon, T. J. Prior, P. S. Pregosin, M. K. Whittlesey and J. M. Williams, J. Am. Chem. Soc., 2007, 129, 1987–1995 CrossRef CAS PubMed.
- T. Kuwahara, T. Fukuyama and I. Ryu, Chem. Lett., 2013, 42, 1163–1165 CrossRef CAS.
- J. Alos, T. Bolano, M. A. Esteruelas, M. Olivan, E. Onate and M. Valencia, Inorg. Chem., 2014, 53, 1195–1209 CrossRef CAS PubMed.
- M. L. Buil, M. A. Esteruelas, J. Herrero, S. Izquierdo, I. M. Pastor and M. Yus, ACS Catal., 2013, 3, 2072–2075 CrossRef CAS.
- W. Ma, S. Y. Cui, H. M. Sun, W. J. Tang, D. Xue, C. Q. Li, J. Fan, J. L. Xiao and C. Wang, Chem. – Eur. J., 2018, 24, 13118–13123 CrossRef CAS PubMed.
- J. C. Borghs, M. A. Tran, J. Sklyaruk, M. Rueping and O. El-Sepelgy, J. Org. Chem., 2019, 84, 7927–7935 CrossRef CAS PubMed.
- S. Bera, A. Bera and D. Banerjee, Chem. Commun., 2020, 56, 6850–6853 RSC.
- K. Paudel, S. Xu and K. Y. Ding, J. Org. Chem., 2020, 85, 14980–14988 CrossRef CAS PubMed.
- A. Singh and M. Findlater, Organometallics, 2022, 41, 3145–3151 CrossRef CAS.
- K. Motokura, D. Nishimura, K. Mori, T. Mizugaki, K. Ebitani and K. Kaneda, J. Am. Chem. Soc., 2004, 126, 5662–5663 CrossRef CAS PubMed.
- K. Motokura, N. Fujita, K. Mori, T. Mizugaki, K. Ebitani, K. Jitsukawa and K. Kaneda, Chem. – Eur. J., 2006, 12, 8228–8239 CrossRef CAS PubMed.
- A. Corma, T. Ródenas and M. J. Sabater, J. Catal., 2011, 279, 319–327 CrossRef CAS.
- Z. Ma, B. Zhou, X. M. Li, R. G. Kadam, M. B. Gawande, M. Petr, R. Zbořil, M. Beller and R. V. Jagadeesh, Chem. Sci., 2022, 13, 111–117 RSC.
- G. Hahn, P. Kunnas, N. d. Jonge and R. Kempe, Nat. Catal., 2019, 2, 71–77 CrossRef CAS.
- S. E. Sayed, A. Bordet, C. Weidenthaler, W. Hetaba, K. L. Luska and W. Leitner, ACS Catal., 2020, 10, 2124–2130 CrossRef.
- Cobalt nanoparticles:
(a) X. J. Cui, W. Li, K. Junge, Z. F. Fei, M. Beller and P. J. Dyson, Angew. Chem., Int. Ed., 2020, 59, 7501–7507 CrossRef CAS PubMed;
(b) D. Nandan, G. Zoppellaro, I. Medřík, C. Aparicio, P. Kumar, M. Petr, O. Tomanec, M. B. Gawande, R. S. Varma and R. Zbořil, Green Chem., 2018, 20, 3542–3556 RSC;
(c) G. Jaiswal, V. G. Landge, M. Subaramanian, R. G. Kadam, R. Zbořil, M. B. Gawande and E. Balaraman, ACS Sustainable Chem. Eng., 2020, 8, 11058–11068 CrossRef CAS.
- X. J. Cui, S. Shyshkanov, T. N. Nguyen, A. Chidambaram, Z. F. Fei, K. C. Stylianou and P. J. Dyson, Angew. Chem., Int. Ed., 2020, 59, 16371–16375 CrossRef CAS PubMed.
- I. S. Pieta, A. Rathi, P. Pieta, R. Nowakowski, M. Hołdynski, M. Pisarek, A. Kaminska, M. B. Gawande and R. Zboril, Appl. Catal., B, 2019, 244, 272–283 CrossRef CAS.
- G. Jaiswal, V. G. Landge, D. Jagadeesan and E. Balaraman, Nat. Commun., 2017, 8, 2147 CrossRef PubMed.
- R. V. Jagadeesh, A.-E. Surkus, H. Junge, M.-M. Pohl, J. Radnik, J. Rabeah, H. Huan, V. Schünemann, A. Brückner and M. Beller, Science, 2013, 342, 1073–1076 CrossRef CAS PubMed.
- R. V. Jagadeesh, K. Murugesan, A. S. Alshammari, H. Neumann, M.-M. Pohl, J. Radnik and M. Beller, Science, 2017, 358, 326–332 CrossRef CAS PubMed.
- B. Zhou, Z. Ma, A. M. Alenad, C. Kreyenschulte, S. Bartling, M. Beller and R. V. Jagadeesh, Green Chem., 2022, 24, 4566–4572 RSC.
- T. Senthamarai, V. G. Chandrashekhar, N. Rockstroh, J. Rabeah, S. Bartling, R. V. Jagadeesh and M. Beller, Chem, 2022, 8, 508–531 CAS.
- P. Ryabchuk, G. Agostini, M.-M. Pohl, H. Lund, A. Agapova, H. Junge, K. Junge and M. Beller, Sci. Adv., 2018, 4, eaat0761 CrossRef PubMed.
- K. Murugesan, V. G. Chandrashekhar, C. Kreyenschulte, M. Beller and R. V. Jagadeesh, Angew. Chem., Int. Ed., 2020, 59, 17408–17412 CrossRef CAS PubMed.
- B. Zhou, V. G. Chandrashekhar, Z. Ma, C. Kreyenschulte, S. Bartling, H. Lund, M. Beller and R. V. Jagadeesh, Angew. Chem., Int. Ed., 2023, 62, e2022156 Search PubMed.
- R. V. Jagadeesh, H. Junge and M. Beller, Nat. Commun., 2014, 5, 4123 CrossRef CAS PubMed.
- K. Murugesan, M. Beller and R. V. Jagadeesh, Angew. Chem., Int. Ed., 2019, 58, 5064–5068 CrossRef CAS PubMed.
- W. Li, J. Rabeah, F. Bourriquen, D. Yang, C. Kreyenschulte, N. Rockstroh, H. Lund, S. Bartling, A.-E. Surkus, K. Junge, A. Brückner, A. W. Lei and M. Beller, Nat. Chem., 2022, 14, 334–341 CrossRef CAS PubMed.
- B. Sahoo, A. E. Surkus, M. M. Pohl, J. Radnik, M. Schneider, S. Bachmann, M. Scalone, K. Junge and M. Beller, Angew. Chem., Int. Ed., 2017, 56, 11242–11247 CrossRef CAS PubMed.
- S. Q. Zhou, L. Shang, Y. X. Zhao, R. Shi, G. I. N. Waterhouse, Y.-C. Huang, L. R. Zheng and T. R. Zhang, Adv. Mater., 2019, 31, 1900509 CrossRef PubMed.
- C. Z. Zhu, Q. R. Shi, B. Z. Xu, S. F. Fu, G. Wan, C. Yang, S. Y. Yao, J. H. Song, H. Zhou, D. Du, S. P. Beckman, D. Su and Y. H. Lin, Adv. Energy Mater., 2018, 8, 1801956 CrossRef.
- X. Li, A.-E. Surkus, J. Rabeah, M. Anwar, S. Dastigir, H. Junge, A. Brückner and M. Beller, Angew. Chem., Int. Ed., 2020, 59, 15849–15854 CrossRef CAS PubMed.
- R. Z. Liu, Y. Z. Zhao, R. X. Huang, Y. J. Zhao and H. P. Zhou, CrystEngComm, 2010, 12, 4091–4094 RSC.
- Z. Z. Du, X. J. Chen, W. Hu, C. H. Chuang, S. Xie, A. J. Hu, W. S. Yan, X. H. Kong, X. J. Wu, H. X. Ji and L.-J. Wan, J. Am. Chem. Soc., 2019, 141, 3977–3985 CrossRef CAS PubMed.
-
NIST X-ray Photoelectron Spectroscopy Database, NIST Standard Reference Database Number 20, National Institute of Standards and Technology, Gaithersburg MD, 20899, 2000 Search PubMed.
- Z. Wang, S. J. Peng, Y. X. Hu, L. L. Li, T. Yan, G. R. Yang, D. X. Ji, M. Srinivasan, Z. J. Pan and S. Ramakrishna, J. Mater., 2017, 5, 4949–4961 CAS.
- L. L. Lin, W. Zhou, R. Gao, S. Y. Yao, X. Zhang, W. Q. Xu, S. J. Zheng, Z. Jiang, Q. L. Yu, Y.-W. Li, C. Shi, X.-D. Wen and D. Ma, Nature, 2017, 544, 80–83 CrossRef CAS PubMed.
|
This journal is © The Royal Society of Chemistry 2024 |
Click here to see how this site uses Cookies. View our privacy policy here.