DOI:
10.1039/D4LF00129J
(Communication)
RSC Appl. Interfaces, 2024,
1, 677-683
Poly(2-methoxyethyl acrylate)-grafted microfiltration membranes exhibiting low-fouling properties in the presence of salt species†
Received
13th April 2024
, Accepted 24th April 2024
First published on 2nd May 2024
Abstract
This study successfully demonstrated that membranes whose surfaces and pore walls were modified with poly(2-methoxyethyl acrylate) (PMEA) exhibit low-fouling properties against organic substances even in the presence of salt species. We also demonstrated the low-fouling property of the modified membrane by using real seawater.
1 Introduction
Membranes used in various processes of water purification and wastewater treatment are susceptible to fouling.1–5 Although pore clogging and pore-entrance blocking have been suggested as the mechanisms of fouling, the adsorption of organic substances, such as proteins and polysaccharides, may also contribute to the increase in filtration resistance.6–8 To suppress adsorptive fouling, a number of surface modification methods using hydrophilic and zwitterionic polymers have been investigated.9–16 These studies use filtration tests to demonstrate that surface modification imparts low-fouling properties to the membranes. However, demonstration tests using model feed solutions are conducted in the absence of salts, which are present in a variety of real feed solutions.
Fane et al.17 reported that the filtration fluxes of aqueous solutions of bovine serum albumin (BSA) through polymeric ultrafiltration membranes depend on the pH and salt concentration of the feed solution, which changes the conformation and charge of the solutes. Zhai et al.18 tested the adsorption of sodium alginate, fulvic acid, humic acid, BSA, and glucan on polyvinylidene difluoride (PVDF) membranes at different sodium chloride concentrations. They suggested that the charge and structure of these substances affect their adsorption, although they did not discuss the effect of adsorption on the filtration flux. Katsoufidou et al.19 conducted filtration tests using aqueous solutions of sodium alginate at different concentrations of calcium ions. Sodium alginate undergoes gelation as the concentration of calcium ions increases, which increases the filtration resistance. These fundamental studies are focused on the effect of salt on the conformation of organic substances that induce membrane fouling, and not the low-fouling properties of the membranes. The effect of salt concentration of crude oil-in-water (O/W) emulsions on the fouling of filtration membranes has been investigated. He et al.20 conducted filtration tests using crude O/W emulsions with different sodium chloride concentrations at a constant flux, revealing that fouling is dependent on the sodium chloride concentration owing to the electro-repulsive force. These oil droplets are generally larger than the organic substances, and accordingly the mechanism of fouling is unlikely organic substance adsorption. In this context, the effect of salt specifically on the low-fouling properties of surface-modified membranes has not been investigated to date.
Poly(2-methoxyethyl acrylate) (PMEA) is a nonionic biomaterial polymer. Although it is not a zwitterionic polymer, its excellent features as biomaterials have been extensively investigated after Tanaka et al. proposed the intermediate water concept.21–23 In this context, PMEA can be a low-fouling polymer for achieving low-fouling properties of membranes through surface modification. However, the surface modification of membranes with PMEA grafting has not been tested to low-fouling membranes, except for the study by Akamatsu et al. They first used PMEA as a low-fouling polymer to microfiltration membranes via plasma graft polymerization.24 This low-fouling performance was also demonstrated by the molecular dynamic simulations.25 Recently, the surfaces of polyethylene microfiltration membranes have been modified with PMEA using a novel surface modification technique in which the membrane is first immersed in advanced oxidized water and then subject to an activator generated by electron transfer–atom transfer radical polymerization (AGET–ATRP).26 This modification method is facile because it does not require deoxygenation nor depressurization, and the modified membranes exhibit excellent fouling resistance against BSA in the absence of salt species. The stability of the PMEA layer was also expected because PMEA was immobilized on the surface via chemical bonds. However, the low-fouling properties of these modified membranes in the presence of salt species have not been demonstrated. Even though PMEA is a nonionic polymer, PMEA is not completely electrically-neutral. As Nagumo et al. reported,27 each constituent group is partially charged. This is because of the difference of the electronegativity. The presence of salts with a high concentration may thus affect the low-fouling properties. Successful demonstration of the low-fouling properties of the membranes grafted with PMEA in the presence of salts will broaden the applicability of these membranes.
In this study, we investigated the low-fouling properties of PMEA-grafted polyethylene microfiltration membranes. The membranes were modified via the facile method we had previously developed and then used to treat not only aqueous solutions of α-amylase and dextrin with or without 3.5 wt% sodium chloride but also real seawater. The reason why α-amylase was selected for the low-fouling evaluation was because it is stable even in an aqueous solution with a high sodium chloride concentration. The results indicated that the modified membranes exhibited low-fouling properties in the presence of salt species.
2 Experimental
2.1 Chemicals and materials
Polyethylene microfiltration membranes with an average pore size of 60 nm, thickness of 30 μm, and porosity of 41% were kindly supplied by Asahi Kasei Corp. (Tokyo, Japan). 2-Methoxyethyl acrylate (MEA), ethanol, super-dehydrated dichloromethane, triethylamine, N,N,N′,N′′,N′′-pentamethyldiethylenetriamine (PMDETA), copper bromide, and a mixture of 63 wt% α-amylase and 37 wt% dextrin were purchased from Fujifilm Wako Pure Chemical Corporation (Osaka, Japan). α-Bromoisobutyl bromide (BIBB) was purchased from Sigma-Aldrich (St. Louis, MO). MEA was distilled prior to use, and the other reagents were used as received.
2.2 Modification of membranes with PMEA
Surface modification was conducted using a previously reported method,26 which is completely free from deoxygenation and depressurization steps. The scheme for the modification is shown in Fig. 1. Briefly, a membrane coupon was immersed in advanced oxidation water for 30 min, which was produced with a home-made water electrolysis device. The concentrations of ozone and hydrogen peroxide were 2 and 0.3 ppm, respectively. The membrane coupon was then immersed in a mixture of super-dehydrated dichloromethane (40 mL) and trimethylamine (3.5 mL), to which BIBB (3.3 mL) was added. After 25 h, the membrane was immersed in 20 mL of an aqueous solution of MEA, ascorbic acid, PMDETA, and copper bromide.
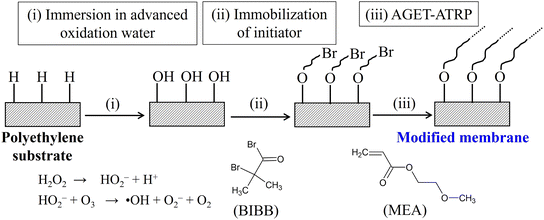 |
| Fig. 1 Schematic illustration of the modification. | |
2.3 Characterization
The grafting amount [mg cm−2] was used for the index, which is defined as the following equation:
Grafting amount [mg cm−2] = (W − W0)[mg]/A [cm2], |
where W0 and W are the weights [mg] of the membrane coupon before and after the grafting reaction of PMEA, respectively. A is the effective membrane area [cm2]. The grafting amount was controlled by the grafting time. PMEA on the membrane surface and pore walls was characterized using Fourier-transform infrared spectroscopy (FT-IR; FT/IR-4200; JASCO Corporation, Tokyo, Japan). Thermogravimetic analysis of the membranes were conducted using a TG analyzer (TGA8000, PerkinElmer Co. Ltd., Japan). The temperature was raised from room temperature to 800 °C, at a rate of 10 °C min−1. The zeta potential of the membrane surface at 25 °C was determined using a zeta-potential and particle size analyzer (ELSZ-2KH, Otsuka Electronics Co., Osaka, Japan). Measurements were conducted using 10 mM sodium chloride aqueous solutions (pH 5.8).
2.4 Membrane resistance and low-fouling properties in the absence/presence of sodium chloride
First, the membrane resistance R [m−1], defined by the following equation, was measured when pure water and aqueous solution containing 3.5 and 7.0 wt% sodium chloride were used as the feed solution:
where J is the flux [m3 m−2 s−1], ΔP is the transmembrane pressure [Pa] and μ is the viscosity [Pa s].
Then low-fouling properties were evaluated. The feed solutions were (1) aqueous solutions of α-amylase (630 ppm) and dextrin (370 ppm) with or without 3.5 wt% sodium chloride and (2) real seawater pre-treated to remove particulate substances. The total organic carbon (TOC) of the treated seawater sample was 2 ppm. Closed-loop cross-flow filtration tests were conducted following a previously reported procedure.26 Briefly, for the first 30 min, the transmembrane pressure (ΔP) was set to obtain a pure water flux of 4 × 10−6 m3 m−2 s−1. Then, the feed was changed to the above feed solution without changing ΔP to examine the low-fouling properties. The transmembrane pressure was increased to 1.5 times and 2.5 times after 120 min and 210 min, respectively. This control of the transmembrane pressure was for eliminating the effect of the intial flux on the fouling, and enabled the focus on the evaluation of the low-fouling properties of the membrane itself. The flow rate and temperature in these tests were 2 L min−1 and 25 °C, respectively.
3 Results and discussion
3.1 Membrane characterization
FT-IR analysis was used to confirm successful grafting of PMEA. Fig. S1† shows the FT-IR spectra of unmodified and modified membranes with a grafting amount of 0.12 mg cm−2, which was used for the subsequent filtration test with real seawater. The spectra were obtained by the transmission method. According to the publications,28,29 a peak was observed at 1727 cm−1 for the dried PMEA film via ATR-IR, which was assigned to the C
O stretching vibration of PMEA. Even though a slight difference in the wavenumber was observed, the characteristic peak at 1735–1730 cm−1 should correspond to PMEA because no other peak was observed near this wavelength. As reported previously,26 the modification method employed in this study achieved uniform grafting across the membrane surface and along the thickness direction and maintained the porous structures as microfiltration membranes. Fig. 2(a) shows the TG curves of the unmodified polyethylene substrate, PMEA and the modified membrane with a grafting amount of 0.19 mg cm−2. This experiment was conducted for the demonstration of the successful modification, not for the exploration of the thermal stability. As for the substrate, a drastic decrease in weight was observed at around 400 °C. As for PMEA, a drastic decrease was observed at around 300 °C. The TG curve of the modified membrane exists between these two curves. The weight loss attributed to the grafted PMEA was first observed and then the loss attributed to the substrate was observed. These curves indicated that the membranes consisted of the polyethylene substrate and PMEA. Fig. 2(b) shows the relationship between the grafting amount and the zeta potential of the modified membrane surface. The zeta potential of the unmodified polyethylene substrate was −10.5 mV. This negative charge was probably because of the preferential adsorption of hydroxide ions on the hydrophobic polyethylene surface, as many researchers discussed.30–33 The membrane surface became neutral with increasing grafting amount. The existence of PMEA probably prohibited such adsorptions. When the grafting amount was 0.17 mg cm−2, the zeta potential was only −3.4 mV. This trend was consistent with that observed for PVDF/PMEA blend membranes prepared by non-solvent induced separation (NIPS).34 The zeta potential approaches zero as the blending ratio of PMEA to PVDF increases. The above changes in the zeta potential with grafting amount also indicated that surface modification was successful.
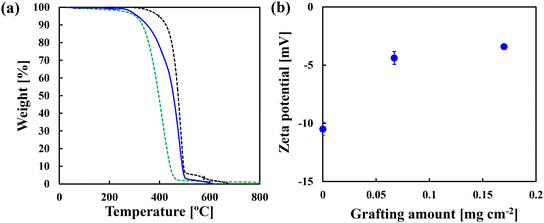 |
| Fig. 2 (a) Thermogravimetric curves of the unmodified polyethylene substrate (black, dashed), PMEA (green, dashed) and grafted membrane (blue, solid). (b) Relationship between the grafting amount and the zeta potential of the membrane surface. | |
3.2 Low-fouling properties in the absence of sodium chloride
Fig. 3 shows the flux changes over time when an aqueous solution of α-amylase (630 ppm) and dextrin (370 ppm) without salts was fed through the membranes. When the feed passing through the unmodified membrane changed to the aqueous solution, the flux gradually decreased from 4 × 10−6 to 1.7 × 10−6 m3 m−2 s−1 in 120 min owing to fouling. After ΔP was increased by 1.5 and 2.5 times the initial condition, the constant fluxes were 2.5 × 10−6 m3 m−2 s−1 at 210 min and 4.1 × 10−6 m3 m−2 s−1 at 300 min. The flux increased because the driving force (ΔP) increased, not because fouling was suppressed. By contrast, with the modified membrane (grafting amount of 0.17 mg cm−2), the flux did not decrease significantly, and it was 3.6 × 10−6 m3 m−2 s−1 in 120 min, indicating that the modified membrane resisted fouling. After ΔP was increased by 1.5 and 2.5 times the initial condition, the fluxes were 4.9 × 10−6 m3 m−2 s−1 at 210 min and 7.5 × 10−6 m3 m−2 s−1 at 300 min. These results indicated that the grafted membrane resisted fouling against α-amylase and dextrin. In our previous study, we also demonstrated that PMEA-modified membranes resisted fouling against BSA.26 This fouling mitigation, combined with the zeta potential of the modified membranes approaching zero with increasing grafting amount, indicated that fouling by these organic substances was suppressed by the unique hydration structure of PMEA as described in the Introduction, and not the electro-repulsive effect.
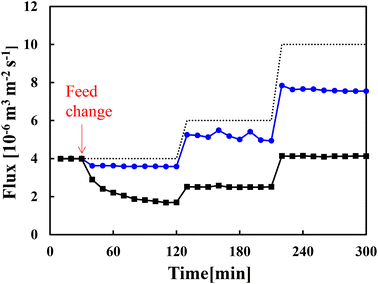 |
| Fig. 3 Time-course of the flux when an aqueous solution of α-amylase (630 ppm) and dextrin (370 ppm) without salts was fed through an unmodified membrane (black, squares) and a grafted (blue, circles) membrane with a grafting amount of 0.17 mg cm−2. The dotted line indicates an ideal flux through an unfouled membrane. | |
3.3 Membrane resistance and low-fouling properties in the presence of sodium chloride
Fig. 4(a) shows the relationship between the concentration of sodium chloride and membrane resistance for the unmodified membrane and a grafted membrane with a grafting amount of 0.22 mg cm−2. In these tests, no organic substances were contained in the feed. As for the unmodified membrane, the resistance was independent of the concentration of sodium chloride. This is because the structure of the polyethylene membranes was not affected. Considering the variation of data on the pure water permeability shown in our previous study,26 which is inversely proportional to the resistance, the resistance for the grafted membrane was almost independent or became small slightly smaller with the concentration of sodium chloride. The grafted PMEA would be slightly dehydrated due to the osmotic pressure difference. However, the membrane itself could be used without any problem. Fig. 4(b) shows the flux changes over time when an aqueous solution of α-amylase (630 ppm) and dextrin (370 ppm) with 3.5 wt% sodium chloride was fed through the membranes. The low-fouling properties of the modified membranes were clearly demonstrated. When the feed passing through the unmodified membrane changed to the aqueous solution, the flux decreased to 1.7 × 10−6 m3 m−2 s−1 in 120 min. When ΔP increased by 1.5 and 2.5 times the initial condition, the constant fluxes were 2.4 × 10−6 m−2 s−1 at 210 min and 3.6 × 10−6 m3 m−2 s−1 at 300 min. At each ΔP condition, the constant fluxes of the solution with and without sodium chloride were comparable, indicating that fouling occurred in the same extent, irrespective of the concentration of sodium chloride (0 or 3.5 wt%). This means that the electro-repulsive effect was not dominant for the fouling. By contrast, with the modified membrane (grafting amount of 0.046 mg cm−2), the flux did not decrease significantly when the feed changed to the solution. The constant fluxes at 120, 210, and 300 min were 2.6 × 10−6, 3.7 × 10−6 and 5.7 × 10−6 m3 m−2 s−1, respectively. An improvement in the low-fouling property was observed when the grafting amount was increased to 0.078 mg cm−2. When the feed changed to the aqueous solution, the flux minimally decreased to 3.6 × 10−6 m3 m−2 s−1 in 120 min. When ΔP increased by 1.5 and 2.5 times the initial condition, the fluxes were 4.8 × 10−6 m3 m−2 s−1 at 210 min and 7.2 × 10−6 m3 m−2 s−1 at 300 min. Therefore, increasing the grafting amount enhanced the low-fouling properties, even in the presence of 3.5 wt% sodium chloride. In an aqueous solution containing 3.5 wt% sodium chloride, the molar ratio of water to sodium chloride is 90
:
1 when the density of the solution is assumed to be 1.02 g cm−3. Based on the experimental results on the low-fouling properties with the estimated molar ratio, it is probable that the unique hydration structure of PMEA is maintained even in the presence of 3.5 wt% sodium chloride and then suppressed the adsorption of organic substances. This should be experimentally demonstrated via other methods in the future.
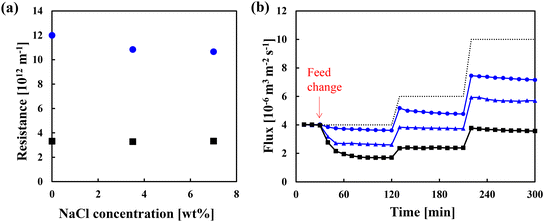 |
| Fig. 4 (a) Relationship between the concentration of sodium chloride and membrane resistance for unmodified (black, squares) and grafted (blue, circles) membranes with a grafting amount of 0.22 mg cm−2. (b) Time-course of the flux when an aqueous solution of α-amylase (630 ppm) and dextrin (370 ppm) with 3.5 wt% sodium chloride was fed through unmodified (black, squares) and grafted (blue, triangles (0.046 mg cm−2), circles (0.078 mg cm−2)) membranes. The dotted line indicates an ideal flux through an unfouled membrane. | |
3.4 Low-fouling properties in real seawater
Fig. 5 shows the flux changes over time when pre-treated real seawater was fed through the membranes. The flux through the unmodified membrane gradually decreased from 1 × 10−5 to 6.6 × 10−6 m3 m−2 s−1 in 24 h. Although the TOC in the feed solution was only 2 ppm, fouling occurred. By contrast, the flux through the modified membrane (grafting amount of 0.12 mg cm−2) minimally decreased to 8.1 × 10−6 m3 m−2 s−1 in 24 h. Although the seawater sample was pre-treated for removing large particulate substances, it contained various ion species, including sodium and chloride ions. The results confirmed that the low-fouling properties of the grafted membrane was maintained in the presence of salts.
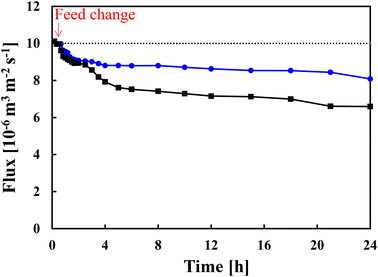 |
| Fig. 5 Time-course of the flux when pre-treated real seawater was fed through unmodified (black, squares) and grafted (blue, circles) membranes with a grafting amount of 0.12 mg cm−2. The dotted line indicates an ideal flux through an unfouled membrane. | |
4 Conclusion
We prepared PMEA-grafted polyethylene microfiltration membranes by treatment with advanced oxidation water followed by AGET–ATRP. The zeta potential of the modified membrane surface approached zero as the grafting amount increased. When the modified membrane was used to filter a solution of α-amylase and dextrin in the absence of salts, the flux did not decrease significantly. By contrast, the flux of the same solution through the unmodified membrane decreased significantly. When the modified membrane was used to filter a solution of α-amylase and dextrin in the presence of 3.5 wt% sodium chloride, the flux did not decrease significantly. By contrast, the flux of the same solution through the unmodified membrane decreased significantly. In addition, increasing the grafting amount enhanced the low-fouling properties of the membrane. Based on the results, it is probable that the unique hydration structure of PMEA suppressed the adsorption of organic substances, even in the presence of sodium chloride. Finally, the results showed that the modified membranes also resisted fouling against pre-treated real seawater. The excellent low-fouling properties of the modified membranes in the presence of salts will broaden the applicability of PMEA-grafted microfiltration membranes.
Author contributions
Kazuki Akamatsu: conceptualization, methodology, investigation, data curation, writing – original draft, supervision, and funding acquisition. Masamune Sano: validation, investigation, data curation, and writing – original draft. Fumio Okada: methodology and investigation. Shin-ichi Nakao: methodology. Xiao-lin Wang: methodology.
Conflicts of interest
There are no conflicts to declare.
Acknowledgements
The authors thank Asahi Kasei Corp., Japan, for supplying the polyethylene microfiltration membrane substrates. The authors also thank the Salt Industry Center of Japan for supplying the pre-treated real seawater sample. A part of this research was supported by a Grant-in-Aid for Scientific Research (B) (grant number 22H01853) from the Japan Society for the Promotion of Science (JSPS). We thank Edanz (https://jp.edanz.com/ac) for editing a draft of this manuscript.
References
- M. B. Asif and Z. Zhang, Ceramic membrane technology for water and wastewater treatment: A critical review of performance, full-scale applications, membrane fouling and prospects, Chem. Eng. J., 2021, 418, 129481, DOI:10.1016/j.cej.2021.129481.
- W. Gao, H. Liang, J. Ma, M. Han, Z. Chen, Z. Han and G. Li, Membrane fouling control in ultrafiltration technology for drinking water production: A review, Desalination, 2011, 272, 1–8, DOI:10.1016/j.desal.2011.01.051.
- N. H. Ismail, W. N. W. Salleh, A. F. Ismail, H. Hasbullah, N. Yusof, F. Aziz and J. Jaafar, Hydrophilic polymer-based membrane for oily wastewater treatment: A review, Sep. Purif. Technol., 2020, 233, 116007, DOI:10.1016/j.seppur.2019.116007.
- P. Krzeminski, L. Leverette, S. Malamis and E. Katsou, Membrane bioreactors – A review on recent developments in energy reduction, fouling control, novel configurations, LCA and market prospects, J. Membr. Sci., 2017, 527, 207–227, DOI:10.1016/j.memsci.2016.12.010.
- M. Maaz, M. Yasin, M. Aslam, G. Kumar, A. E. Atabani, M. Idrees, F. Anjum, F. Jamil, R. Ahmad, A. L. Khan, G. Lesage, M. Heran and J. Kim, Anaerobic membrane bioreactors for wastewater treatment: Novel configurations, fouling control and energy considerations, Bioresour. Technol., 2019, 283, 358–372, DOI:10.1016/j.biortech.2019.03.061.
- K. Akamatsu, Y. Kagami and S. Nakao, Effect of BSA and sodium alginate adsorption on decline of filtrate flux through polyethylene microfiltration membranes, J. Membr. Sci., 2020, 594, 117469, DOI:10.1016/j.memsci.2019.117469.
- E. Matthiasson, The role of macromolecular adsorption in fouling of ultrafiltration membranes, J. Membr. Sci., 1983, 16, 23–36, DOI:10.1016/S0376-7388(00)81297-1.
- Y. F. Yang, Y. Li, Q. L. Li, L. S. Wan and Z. K. Xu, Surface hydrophilization of microporous polypropylene membrane by grafting zwitterionic polymer for anti-biofouling, J. Membr. Sci., 2010, 362, 255–264, DOI:10.1016/j.memsci.2010.06.048.
- K. Akamatsu, W. Noto, H. Fukuzawa, A. Hara and S. Nakao, Grafting of carboxybetaine polymers to polyethylene membranes via plasma graft polymerization to improve low-fouling properties and to tune the molecular weight cut-off, Sep. Purif. Technol., 2018, 204, 298–303, DOI:10.1016/j.seppur.2018.05.004.
- S. Ayyaru and Y. H. Ahn, Application of sulfonic acid group functionalized graphene oxide to improve hydrophilicity, permeability, and antifouling of PVDF nanocomposite ultrafiltration membranes, J. Membr. Sci., 2017, 525, 210–219, DOI:10.1016/j.memsci.2016.10.048.
- Y. H. Chiao, H. T. Lin, M. B. M. Y. Ang, Y. H. Teow, S. R. Wickramasinghe and Y. Chang, Surface Zwitterionization via Grafting of Epoxylated Sulfobetaine Copolymers onto PVDF Membranes for Improved Permeability and Biofouling Mitigation, Ind. Eng. Chem. Res., 2023, 62, 2913–2923, DOI:10.1021/acs.iecr.2c04382.
- K. M. Dobosz, C. A. Kuo-LeBlanc, T. Emrick and J. D. Schiffman, Antifouling Ultrafiltration Membranes with Retained Pore Size by Controlled Deposition of Zwitterionic Polymers and Poly (ethylene glycol), Langmuir, 2019, 35, 1872–1881, DOI:10.1021/acs.langmuir.8b02184.
- M. Hadidi and A. L. Zydney, Fouling behavior of zwitterionic membranes: Impact of electrostatic and hydrophobic interactions, J. Membr. Sci., 2014, 452, 97–103, DOI:10.1016/j.memsci.2013.09.062.
- M. Quilitzsch, R. Osmond, M. Krug, M. Heijnen and M. Ulbricht, Macro-initiator mediated surface selective functionalization of ultrafiltration membranes with anti-fouling hydrogel layers applicable to ready-to-use capillary membrane modules, J. Membr. Sci., 2016, 518, 328–337, DOI:10.1016/j.memsci.2016.07.007.
- P. Zhang, S. Rajabzadeh, T. Istirokhatun, Q. Shen, Y. Jia, X. Yao, A. Venault, Y. Chang and H. Matsuyama, A novel method to immobilize zwitterionic copolymers
onto PVDF hollow fiber membrane surface to obtain antifouling membranes, J. Membr. Sci., 2022, 656, 120592, DOI:10.1016/j.memsci.2022.120592.
- X. Zhao and C. He, Efficient Preparation of Super Antifouling PVDF Ultrafiltration Membrane with One Step Fabricated Zwitterionic Surface, ACS Appl. Mater. Interfaces, 2015, 7, 17947–17953, DOI:10.1021/acsami.5b04648.
- A. G. Fane, C. J. D. Fell and A. Suki, The effect of pH and ionic environment on the ultrafiltration of protein solutions with retentive membranes, J. Membr. Sci., 1983, 16, 195–210, DOI:10.1016/S0376-7388(00)81310-1.
- Y. Zhai, D. Bai, Y. Wang, Y. Zhang, Y. Qi, X. Qiu, Y. Wang, Y. Wang and X. Zheng, Effect of Na+ on organic fouling depends on Na+ concentration and the property of the foulants, Desalination, 2022, 531, 115709, DOI:10.1016/j.desal.2022.115709.
- K. Katsoufidou, S. G. Yiantsios and A. J. Karabelas, Experimental study of ultrafiltration membrane fouling by sodium alginate and flux recovery by backwashing, J. Membr. Sci., 2007, 300, 137–146, DOI:10.1016/j.memsci.2007.05.017.
- Z. He, S. Kasemset, A. Y. Kirschner, Y. H. Cheng, D. R. Paul and B. D. Freeman, The effects of salt concentration and foulant surface charge on hydrocarbon fouling of a poly(vinylidene fluoride) microfiltration membrane, Water Res., 2017, 117, 230–241, DOI:10.1016/j.watres.2017.03.051.
- M. Tanaka, T. Motomura, M. Kawada, T. Anzai, Y. Kasori, T. Shiroya, K. Shimura, M. Onishi and A. Mochizuki, Blood compatible aspects of poly(2-methoxyethylacrylate) (PMEA)—relationship between protein adsorption and platelet adhesion on PMEA surface, Biomaterials, 2000, 21, 1471–1481, DOI:10.1016/S0142-9612(00)00031-4.
- M. Tanaka, T. Motomura, N. Ishii, K. Shimura, M. Onishi, A. Mochizuki and T. Hatakeyama, Cold crystallization of water in hydrated poly(2-methoxyethyl acrylate) (PMEA), Polym. Int., 2000, 49, 1709–1713, DOI:10.1002/1097-0126(200012)49:12<1709::AID-PI601>3.0.CO;2-L.
- M. Tanaka, T. Hayashi and S. Morita, The roles of water molecules at the biointerface of medical polymers, Polym. J., 2013, 45, 701–710, DOI:10.1038/pj.2012.229.
- K. Akamatsu, T. Furue, F. Han and S. Nakao, Plasma graft polymerization to develop low-fouling membranes grafted with poly(2-methoxyethylacrylate), Sep. Purif. Technol., 2013, 102, 157–162, DOI:10.1016/j.seppur.2012.10.013.
- R. Nagumo, K. Akamatsu, R. Miura, A. Suzuki, N. Hatakeyama, H. Takaba and A. Miyamoto, A Theoretical Design of Surface Modifiers for Suppression of Membrane Fouling: Potential of Poly(2-methoxyethylacrylate), J. Chem. Eng. Jpn., 2012, 45, 568–570, DOI:10.1252/jcej.12we110.
- K. Akamatsu, M. Sano, F. Okada, S. Nakao and X. Wang, Immersion in Advanced Oxidation Water Followed by AGET-ATRP to Develop Low-Fouling Microfiltration Membranes by Grafting Poly(2-methoxyethyl acrylate), Ind. Eng. Chem. Res., 2023, 62, 10611–10618, DOI:10.1021/acs.iecr.3c01571.
- R. Nagumo, T. Matsuoka and S. Iwata, Interactions between Acrylate/Methacrylate Biomaterials and Organic Foulants Evaluated by Molecular Dynamics Simulations of Simplified Binary Mixtures, ACS Biomater. Sci. Eng., 2021, 7, 3709–3717, DOI:10.1021/acsbiomaterials.1c00609.
- S. Morita, M. Tanaka and Y. Ozaki, Time-Resolved In Situ ATR-IR Observations of the Process of Sorption of Water into a Poly(2-methoxyethyl acrylate) Film, Langmuir, 2007, 27, 3750–3761, DOI:10.1021/la0625998.
- M. Tanaka, S. Morita and T. Hayashi, Role of interfacial water in determining the interactions of proteins and cells with hydrated materials, Colloids Surf., B, 2021, 198, 111449, DOI:10.1016/j.colsurfb.2020.111449.
- P. Dou, N. Xu, H. Wen and C. Liu, Facile hydrophilization of polyethylene substrate by in situ polymerization of m-phenylenediamine for preparing high-performance polyamide reverse osmosis membrane, J. Membr. Sci., 2023, 683, 121777, DOI:10.1016/j.memsci.2023.121777.
- N. Burmeister, E. Zorn, L. Preuss, D. Timm, N. Scharnagl, M. Rohnke, S. G. Wicha, W. R. Streit and W. Maison, Low-Fouling and Antibacterial Polymer Brushes via Surface-Initiated Polymerization of a Mixed Zwitterionic and Cationic Monomer, Langmuir, 2023, 39, 17959–17971, DOI:10.1021/acs.langmuir.3c02657.
- N. Sun, P. Dou, W. Zhai, H. He, L. D. Nghiem, V. Vatanpour, Y. Zhang, C. Liu and T. He, Polyethylene separator supported thin-film composite forward osmosis membranes for concentrating lithium enriched brine, Water Res., 2022, 216, 118297, DOI:10.1016/j.watres.2022.118297.
- R. Zangi and J. B. F. N. Engberts, Physisorption of Hydroxide Ions from Aqueous Solution to a Hydrophobic Surface, J. Am. Chem. Soc., 2005, 127, 2272–2276, DOI:10.1021/ja044426f.
- S. Ohno, S. Nakao, X. Wang and K. Akamatsu, Morphology and Surface Characteristics of PVDF/Poly(2-methoxyethyl acrylate) Blend Membranes Prepared via NIPS and Their pH-Independent Low-Fouling Properties, Ind. Eng. Chem. Res., 2022, 61, 15326–15335, DOI:10.1021/acs.iecr.2c02271.
|
This journal is © The Royal Society of Chemistry 2024 |
Click here to see how this site uses Cookies. View our privacy policy here.