DOI:
10.1039/D4LF00177J
(Review Article)
RSC Appl. Interfaces, 2024,
1, 1108-1128
Topographical characteristics of 3D printed polymeric microneedle surface and its impact on coating formulation attributes
Received
17th May 2024
, Accepted 31st August 2024
First published on 20th September 2024
Abstract
In recent years, 3D printing has emerged as a promising technique for fabricating polymeric microneedles (μNDs), offering flexibility and precision. However, the surface topography of these μNDs plays a pivotal role in determining coating formulation characteristics and, consequently, their efficacy in drug delivery applications. This review delves into contemporary approaches for evaluating the surface topography of 3D printed polymeric μNDs, exploring its impact on coating formulation characteristics. With reference to the latest research in the materials industry, we elucidate the close relationship between the adhesion of coating material and μND surface, considering their physical and chemical properties. Fundamental principles relevant to understanding the influence of surface topography on therapeutic coating are discussed, emphasising how surface microtopography influences adhesion strength. Moreover, non-contact methodologies such as profilometry are reviewed for characterising μND surface morphology, highlighting their utility in assessing surface roughness, porosity, and feature geometry. Additionally, we explore how coating formulation attributes dictate surface wetting, surface energy, and interfacial adhesion strength. Insights from this review offer valuable guidance for optimising the fabrication process of 3D printed polymeric μNDs, tailoring their surface topography to enhance coating formulation performance in diverse biomedical applications.
1. Introduction
Microneedle (μND) arrays are promising biomedical devices in the transdermal drug delivery (TDD) platform. Compared to traditional injection methods, μNDs can penetrate the skin and create transient microchannels in the stratum corneum (SC), while also taking advantage of the abundant cellular network in the skin tissue. However, the temporary microchannels have been shown to close quickly. For example, Haridass et al. showed that after the application of Nanopatch®, a high-density μND, microchannels were closed by 25% in diameter within the first 30 min and then 100% by 6 h post-application.1 Nonetheless, the process of inserting μND arrays into the skin causes less irritation to the subcutaneous nerves and blood vessels, and thus it is considered painless and safe.2 A recent study quantified pain on μND treated skin using a visual analogue scale (VAS) and showed that even projections of μND longer than 1 mm caused 40% less pain than hypodermic needles.3 In addition, due to its simple operation, the μND can be administered by the patient, which reduces the need for trained personnel and has high patient compliance.4 Furthermore, the advantage of bypassing the first-pass metabolism and the harsh condition of the gastrointestinal tract linked to oral administration make μNDs a useful tool for delivering therapeutics transdermally.5
Transdermal μNDs were originally pioneered by Mark Prausnitz's group in the late 1990s, demonstrating successful skin penetration of solid μNDs, followed by the application of a calcein-embedded patch on the prorated skin surface.6 This two-step method “poke and patch” proved highly efficient in increasing calcein drug permeability into the skin epidermis. However, this approach is not efficient due to i) its multi-step process (create pores, then apply the drug patch) and ii) the micropores are closed after some time post microoperation, consequently limiting the diffusion of therapeutics.5,7 To overcome these limitations, coated μNDs (CμNDs) were explored by researchers, utilising the ‘coat and poke’ approach.8 CμNDs consist of solid μNDs coated with a drug-containing biocompatible material.9 When the μND is inserted into the skin, the water-soluble coating bonded to the needle surface detaches from the projection and is dissolved in the skin, thus, delivering a wide range of active molecules, such as small molecules, viruses, DNA, and proteins.10,11 CμNDs can only carry small doses (up to 1 mg) of drugs and are only suitable for small and potent amounts, making them widely used in vaccine research.12–14 Several trials have shown that a microscopic level of vaccine delivered using μNDs can successfully activate the body's immune response.15–17 This saves a considerable amount of vaccine dose and reduces the cost and side effects of vaccination compared to vaccination by hypodermic needle injection.12 In addition, the high storage stability of vaccines carried on μNDs reduces the reliance on cold chain storage and transportation of vaccines. It makes it highly valued in non-developed countries where resources are limited.2,12 A major challenge encountered with CμNDs is that when μNDs are inserted into the skin, a part of the coating material remains on the skin surface and μNDs, making this part of the drug unable to enter the skin and be absorbed by the body (Fig. 1).11
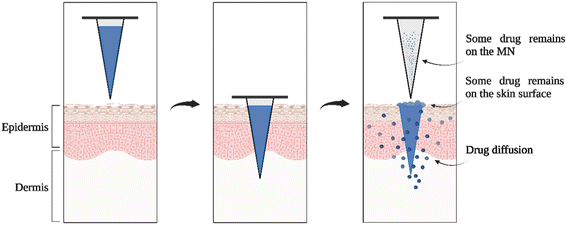 |
| Fig. 1 Schematic illustration of methods of CμNDs application to the skin. Figure created with https://BioRender.com and redrawn from ref. 11. | |
Since then, researchers have utilised multiple approaches to overcome this issue, such as dip-coating, gas jet drying, spray coating, electrohydrodynamic atomisation (EHDA), and ink-jet printing. These methods overcome drug waste and loss, variable coating thickness, and inaccurate drug dosage.18 These coating approaches were well documented by Haj-Ahmed et al., briefly; dip-coating method involves dipping the μND into a formulation, creating a thin film over the μND surface which eventually dries into solid particles, gas-jet approach disperses a small amount of coating solution over the μND surface that removing and drying the coating solution simultaneously, spray-coating approach involves the atomisation of microdroplet formulation onto the μNDs which coalesce to form a thin film over the μNDs, EHDA coating involves producing atomised microdroplet formulation using an electrical force which is then sprayed onto the μND tips, ink-jet printing approach involves spraying a microdroplet formulation using a piezoelectric dispenser onto the μND surface for a more uniform, accurate, and reproducible coating.19
Researchers have also employed ink-jet printing technology to optimise coating, ejecting tiny droplets with increased control and accuracy (droplet size) on the surface of μND projection to form a uniform coating layer. Economidou et al. coated insulin and sugar alcohol on 3D printed μNDs using the ink-jet printing technique, forming a uniform coating with no satellite droplets falling on the μNDs substrate.20 Uddin et al. reported in 2015 that ink-jet printing can coat three anticancer agents (5-fluororacil, curcumin, and cisplatin) evenly on metal μNDs.21 In Fig. 2, Scanning Electron Microscope (SEM) analysis demonstrated that ink-jet printing technology could precisely achieve high consistency and reproducibility for μND coating. This smoothly and uniformly coated film prevents drug loss during μND insertion, as mentioned earlier.20
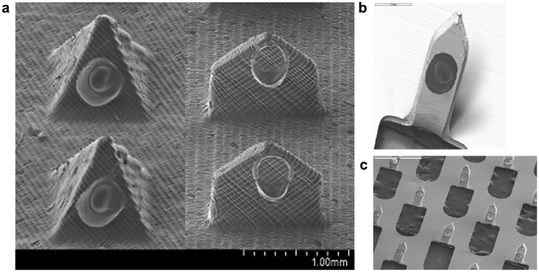 |
| Fig. 2 Examples of ink-jet printed μND. (a) Coated pyramid and spear,20 (b) and (c) μND arrays coated via ink-jet printing with high precision and accuracy.21 | |
Past studies demonstrated a correlation between surface roughness, porosity, and coating attributes.22 In this review, we comment and extrapolate from recent literature on the relationship between topographical features and coating efficiency, as well as elucidate the relationship between surface topography and improving coating efficiency. Further, we present the most common fabrication methods and coating approaches of μNDs. We then combine the latest progress in the fields of material science and coating formulation dynamics, from the perspective of the topographical features and coating attributes of μNDs and analyse the effects of these factors on achieving uniformity in the coating of therapeutics on μND surfaces.
2. Fabrication of μNDs
μNDs fabrication techniques have been evolving ever since ALZA corporation first introduced the concept of a μND in 1976, however, it was not possible to make such micro-structured devices until the 1990s with the advent of high precision microelectronics tools.23 Furthermore, the first paper to demonstrate μNDs technology for TDD was not until 1998, which showed a 4-fold increase in the absorption of a model drug in the human skin in vitro.24 This method was, however, a poke and patch approach, where the drug diffusion is limited to the time the microchannels remain open. To alleviate that, Matriano et al. first reported coated silicon μND patches by immersion in a coating solution, followed by successful skin application and delivery of protein antigen for immunisation purposes.25 Earlier CμNDs were mostly made from inorganic materials such as silicon, ceramics, and metals.14,26 This was primarily due to the material strength and modulus needed to withstand the biomechanical strength of the skin.26,27 It required utilising expensive instruments to optimise μND dimensional parameters such as needle geometry, size, shape, and width which depended on the skin layer depth,23 which makes the fabrication process both costly and time prohibitive.11 Researchers have since employed low-cost and efficient fabrication techniques to manufacture μNDs, such as drawing lithography, micro-molding, and 3D printing.28 These fabrication techniques will be highlighted in the following section. Additionally, the types of materials used, advantages and limitations of these fabrication techniques from recent studies has been highlighted in Table 1.
Table 1 Types of materials used, advantages and limitations of these fabrication methods
Method |
Typical materials |
Advantages |
Limitations |
Ref. |
N.B. for spatial resolution, the manufacturing methods are rated from least to highest convenience with regards to manufacturing process. DAB: droplet air borne, TPP: two photon polymerisation, CLIP: continuous liquid interface production, FDM: fused deposition modelling, PLA: poly(lactic acid), ABS: acrylonitrile butadiene styrene, ASA: acrylonitrile styrene acrylate, PC: polycarbonate, PPSF/PPSU: polyphenylsulfones, PEI: polyethyleneimine, ULTEM: polyetherimide. |
Lithography (electro-, thermal and magnetorheological drawing, photolithography, X-ray lithography and centrifugal) |
Epoxy/novolac, thermoplastics and photo resins biocompatible polymers, ceramics |
↑ Spatial resolution and consistency between batches, solid and hollow μNDs, ↑ precision |
UV/X-ray curing of polymers can inactivate loaded drug, complex machinery, scale-up issues |
29–33
|
Micromoulding |
Biodegradable polymers |
↑ Precision and consistency, multiple μNDs from a single mould |
↑ Cost, complexity of mould design, scaling up challenges |
34 and 35 |
Additive manufacturing: SLA, DLP, CLIP, TPP |
Epoxides, acrylates, rigid (RPU) and flexible (EPU) polyurethanes, cyanate ester (CE), and prototyping (PR) resolution 100 nm–10 μm |
↑ Spatial resolution (SLA < DLP < CLIP < TPP)*, built speed (TPP < SLA < DLP < CLIP), accuracy and precision (SLA < DLP < CLIP < TPP), DLP ↓ effect by oxygen inhibition |
Limited materials, slow and expensive printing, SLA UV-curing of polymer matrix makes loaded drug incompatible, limited mechanical properties, CLIP requires low viscosity resin and TPP requires ↑ photon density laser |
36–40
|
Additive manufacturing: FDM™ |
Thermoplastic and fiber-reinforced polymers, PLA, ABS, ASA, Nylon12, PC, PPSF/PPSU, PEI, ULTEM 50–200 μm |
↓ Cost, ↑ speed and simplicity |
↓ Mechanical properties, slow, rough surface, ↑ the temperature during the extrusion process, incompatible drug loading |
36 and 37 |
2.1 Lithography
Drawing lithography is the deformation of polymers by external stimuli, such as light,41,42 centrifugal force,43,44 electric45,46 and magnetic fields.29,31,47 This technique usually involves the transfer of the master pattern of geometric shapes onto a substrate surface.29,48–51 Photolithography is the most common technique used for pattern transfer which is widely used in the field of microelectronics.52–55 Lithography requires precise processing of the photoresist, which can be either methacrylate-based photopolymeric, photocrosslinker, or photodecomposer.52–55 Lithography possesses the ability to create products from a variety of materials (glass, metal, ceramics, and plastics) with high geometrical precision and smooth vertical sidewalls.29,48–55 However, this technique requires advanced cleanroom facilities51 and extended production time.48,54
2.2 Micromoulding
Micro-molding process consists of making replica master mould using a two-step process: first manufacturing a positive master μND array using laser34 or lithography,56 then using that array to fabricate replicas of negative μND mould.57 This technique of μND fabrication is cost-effective for mass production.34 Poly dimethyl siloxane (PDMS) is generally used to make negative micromould replicas due to its low cost, ease of use, low surface energy, and thermostability.58–60 The limitations of this fabrication technique are the drug loading capacity and mechanical behavior of the polymer.61
2.3 Additive manufacturing (3D printing)
3D printing as an additive manufacturing (AM) method enables precise and reproducible fabrication of high-resolution and high-quality μNDs,62 and can also rapidly modify the parameters associated with μNDs with the aid of computer-aided design (CAD). In addition, most 3D printing uses biocompatible polymers as raw materials, which facilitates the application of μNDs in the field of biological sciences.62 As an efficient and inexpensive method, 3D printing is based on two classifications: material extrusion (filament-based) and photopolymerisation (resin-based). Material extrusion-based fused deposition modeling (FDM) 3D printers heat thermoplastic filament and eject it through a nozzle, where it cools and is solidified into a predetermined structure on a build platform.63 Luzuriaga et al. printed polylactic acid (PLA) using an FDM 3D printer to create biodegradable and renewable μNDs.64 Despite the utilisation of a large collection of thermoplastic polymers, there are disadvantages such as print layer distortion, poor surface quality, print layer thickness, width, and orientation of filament materials, which translate to dimensional inaccuracies and lower structural integrity and surface texture.28
Secondly, technologies such as stereolithography (SLA), digital light processing (DLP), liquid crystal display (LCD), continuous liquid interface production (CLIP), and two-photon polymerisation (TPP/2PP) are based on photopolymerisation or photocuring.13,62,63,65 Among these technologies, structures are manufactured layer-wise using UV light to polymerise photosensitive polymers (e.g., resins). SLA is widely used due to its low cost and the high mechanical strength of the printed product.10,66,67 Krieger et al. developed a two-step “print and fill” fabrication method for manufacturing μND master molds.57 Unlike SLA, which uses a point light source for printing, DLP prints on the principle of printing the entire cross-section of an object at once. Therefore, even though DLP printers are very expensive, they are widely used in μND manufacturing because of their faster printing speed and high resolution.68–71 To date, stereolithography (SLA) and digital light processing (DLP) 3D printing have been widely explored to manipulate structural changes in μNDs.72–74 This is due to the layer-by-layer polymerisation of UV-sensitive polymers when exposed to light with a specific wavelength (usually 350–450 nm). Xenikakis et al. printed hollow μNDs (HμND) using biocompatible resin using LCD technology.75 As an optimised solution to traditional SLA, CLIP 3D printing has excellent printing characteristics. Caudill et al. used CLIP technology to ensure the accuracy and consistency of μND fabrication, especially producing μNDs with a smoother finish rather than a rougher finish, i.e., diminishing the ‘stair-stepping’ phenomenon effect on the μND surface.76 Moreover, one study showed that CLIP technology can be used to manufacture μNDs of almost any shape in one step.77 TPP 3D printing technology has shown the highest printing accuracy to date, enabling layer-by-layer fabrication of 3D structures at the nanoscale, and has already been commercialised.13,78 Faraji Rad et al. used TPP technology to fabricate high-precision μND arrays with complex structures.78 Moreover, 3D printed μNDs exhibiting dimensional variability (geometrical tweaking) possess excellent skin penetration abilities,73,74 and this quality is very important and is required to overcome the biomechanical forces exhibited by the skin fauna during a μND penetration event.79
3. Topographical factors determining high fidelity μNDs
When viewed under a high-magnification microscope, the smooth surface of any material appears rough, consisting of a series of “peaks and valleys”. From a micro perspective, the coating of the drug solution on μND is the adherence of the drug microparticles to the surface of the μND. The degree of adhesion of the coating material to the substrate determines the performance of the coating, and this interaction is strongly influenced by the contact topography. Also, coated films can achieve very high adhesion with rough surfaces within a certain thickness limitation.80 After the shot peening of the substrate by Lupicka et al., the surface roughness of the substrate increased, which increased the surface area and the porosity ratio in contact between the coating material and the substrate, thus promoting the mechanical locking between them.81,82 Surface roughness, porosity, and surface area are different physical properties of materials that are often interrelated, which will be discussed below.
3.1 Surface roughness
Surface roughness is deemed the deviation of the surface micro-features of a material from its ideal properties.83,84 According to the relevant ISO standards, the surface roughness-related parameters include the R-system parameters in 2D and the S-system parameters in 3D. Most measurement methods assume that the roughness profile of the surface is irregular and random; therefore, the microscopic surface height of the object to be measured is often predicted to be normally distributed. Referring to ISO 4287 and ISO 13565, the profile is usually described by one or more of Ra, Rz, Rq, and Rk.84 From a computational point of view, they represent the arithmetical mean deviation of the roughness profile, the maximum height of the roughness profile, the root mean square deviation of the roughness profile, and the core roughness depth, respectively.83,84
Past research has shown that the topographical structure and chemical energy of a solid surface affect its wettability,85 which represents the ability of a liquid to spread or adhere to a solid surface. Naat et al. investigated the improvement of surface wettability by increasing surface roughness through structured textures. The bionic textures inspired by the tree frog (TF) and fish scale (FS) lead to super-wetting of the surface and enhanced bond strength.86 Surface wetting characteristics, including hydrophilicity and hydrophobicity, play an important role in the adhesion properties of the coatings.87 Barreau et al. reported that fibrillar gecko-inspired adhesive structures can form good bonds with rough surfaces. The use of fibrillar adhesives increased the adhesion to rough substrates by a maximum of four times compared to smooth surfaces without patterns.88 This operation of improving adhesion by modifying roughness has also been observed in studies of μNDs. Common pyramid or cone μNDs also exhibit a “stepped” microscopic appearance using high magnification SEM (Fig. 3).57,89 This edge-ridge mismatch is a manifestation of the stair-stepping effect. Krieger et al. set up three print layer heights (25, 50, and 100 μm) and found that increasing the print layer height significantly reduced the surface finish, as shown in Fig. 3. This is because the edges at an angle to the Z-axis are rougher, making the projected surface rougher.57
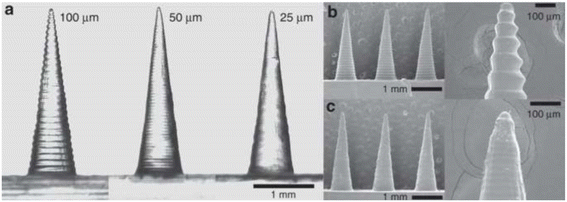 |
| Fig. 3 Images of μNDs printed at different layer heights. (a) Comparison of 100, 50, and 25 μm layer height μNDs; (b) and (c) SEM images of 100 μm and 25 μm layer height μNDs. Image was adopted from ref. 57 with permission. | |
Contact and non-contact measuring instruments are often used to measure surface roughness. The contact measurement method is based on the movement of a conical probe, usually made of diamond, over the surface of the object, obtaining topographical information about the joint profile. The stylus profilometer is a representative contact measuring instrument with low cost and high portability.90 According to the Olympus manual, since the radius of the stylus is approximately 2 μm, when the stylus is used to measure the surface roughness of less than 2 μm, the measurement accuracy is limited, and a certain systematic error occurs. Additionally, contact measuring instruments have the potential to damage the surface of an object during physical contact with the object. Another disadvantage of this method is that it needs to be performed in a strict measurement environment, which is difficult to operate and can only be used for off-line measurements.91,92 Several different approaches to non-contact measurement are proposed in the literature, respectively based on ultrasound, optics, scanning tunneling microscopy (STM), and machine vision technology. Due to expensive equipment and the requirements of the measurement range and size of the test object, the application of the first three methods has been limited. On the contrary, the measurement strategy based on machine vision is favored by researchers because of its high efficiency, high precision, collection of large amounts of information, high-cost performance, and non-contact feature.91
3.2 Porosity
Porosity refers to the proportion of the total volume occupied by the non-solid pore space in an object. The pore morphology affects the physical properties of the material, including density, mechanical strength, and thermal conductivity.93 According to the literature, the methods of porosity detection have been divided into two categories based on the order of processing, namely i) post-processing detection, i.e., the examination of pores present in the finished object that is the most common, and ii) the inspection of pores being molded during the manufacturing process.94
Porosity is also an important property for applying μNDs. Porous μNDs (PμNDs) are a novel drug-loading platform with a rich microporous structure, characterised by a high surface area. Because of their unique structural features, PμNDs can utilise the capillary action generated by interconnected pores to deliver drugs and extract dermal interstitial fluid (ISF).95 According to the scale of pore structure PμNDs have different mechanical strengths, the capacity for drug loading, and the capacity for extraction,96 which makes them have a wide range of application besides drug delivery such as biosensing.
Many measurement methods have been developed and improved to measure porosity, such as porosimetry respectively based on Archimedes' principle, mercury and helium pycnometry, and lastly, image analysis methods.93,97 Archimedeans and mercury porosimetry are widely used in science. Archimedeans porosimetry is cost-effective and convenient to operate, but it can only quantify porosity and provide limited pore information. Mercury porosimetry has high detection accuracy, but toxic high-concentration mercury residues may occur during the detection process.93,98 Helium pycnometry measures porosity using helium gas penetrating the pores, which is highly accurate due to the high diffusivity of helium as an inert gas. But because helium gas cannot reach the closed pore regions, it can only measure the open porosity of the sample.22 Image analysis methods are promising due to their ability to collect large amounts of data without generating toxic residues and are broadly divided into two-dimensional (2D) image analysis (optical microscopy and SEM) and three-dimensional (3D) image analysis (X-ray microtomography).98Table 2 lists the methods or instruments used in the research to measure the surface roughness and porosity of objects.
Table 2 Methods of measuring surface roughness and porosity
Classification |
Measurement methods/instrument |
Advantage/disadvantages |
Ref. |
Contact roughness |
KLA-Tensor P-15 long scan contact stylus profiler |
Inexpensive and portable device |
99
|
Commercial scanning probe |
The presence of systematic errors |
100
|
Tactile surface profilometer (DektakXT Stylus Profiler) |
Possibility of damaging the surface |
101
|
Surface profilometry (Surfcorder SE 1700) |
Strict measurement environment |
102
|
Limitations of offline measurements |
Non-contact roughness |
Scanning electron microscopy (SEM) analysis |
High efficiency and precision |
99
|
Confocal laser scanning microscopy (CLSM), Olympus LEXT OLS3100 |
Collection of large amounts of information |
103
|
White-light profilometer (ALTISURF 500) |
Non-destructive technique |
104
|
Focus variation (FV) microscopy (Alicona InfiniteFocusSL) |
Expensive equipment |
105
|
Talyscan-150 optical profiler (Taylor Hobson Precision Ltd) |
Limitations of the measurement range and size of the test object |
106
|
X-ray computed tomography (CT) system (Nikon Metrology MCT 225) |
107
|
Archimedeans porosimetry |
Measure the weight of the specimen before and after imbibition based on the Archimedes buoyancy principle |
Cost-effective and easy to operate |
93
|
Provide limited pore information |
Mercury porosimetry |
Hg-porosimeter (Pascal 140/240 Thermo Fisher) |
High detection accuracy |
108
|
Toxic high levels of mercury residues |
Helium pycnometry |
Micromeritics Tristar 3020 automated surface area and pore size analyser (Norcross, GA, USA) |
High detection accuracy |
109
|
Cannot measure the closed porosity |
Computed micro-tomography (CMT, VersaXRM-500, Xradia, Zeiss, Jena, Germany) and helium pycnometer |
High detection accuracy |
22
|
Measure both open and closed porosity |
Image analysis method |
Stereo and scanning electron microscopy |
Collect large amounts of data |
110
|
Optical microscope (OM) and JEOL 7000 FEG-SEM (scanning electron microscope) |
No toxic residues |
102
|
X-ray computed tomography (XCT) technique (Nikon Metrology 225/320 kV custom bay system) |
Non-destructive technology |
105
|
Micro-computed tomography (microCT; Bruker AXS GmbH) |
Relatively expensive equipment |
111
|
Using ImageJ (version 1–50) to analyse image |
100
|
3.3 Surface area
The shape and size of μNDs affect the application area, mechanical strength, and drug loading amount of μNDs (Fig. 4). This correlation has been extensively covered in past publications by Coulman et al.,112 Prausnitz et al.,113,114 De Martino et al.,115 Donnelly et al.,116,117 Liu et al.,4 Gill et al.,11 and very recently in our publication, Ali et al.28 These reviews have sufficiently compiled a repository of research articles that demonstrates how different μND geometries influence the mechanical performance, skin penetration, and adhesion of coating formulations, as well as the efficiency of drug delivery systems, including the minimally invasive delivery of macromolecules and plasmid DNA. Additionally, they comprehensively review microfabrication, drug delivery mechanisms, safety considerations, material science, manufacturing processes, and the commercial development of μNDs, with a focus on the pivotal role of μND geometry in enhancing drug delivery and diagnostic applications.
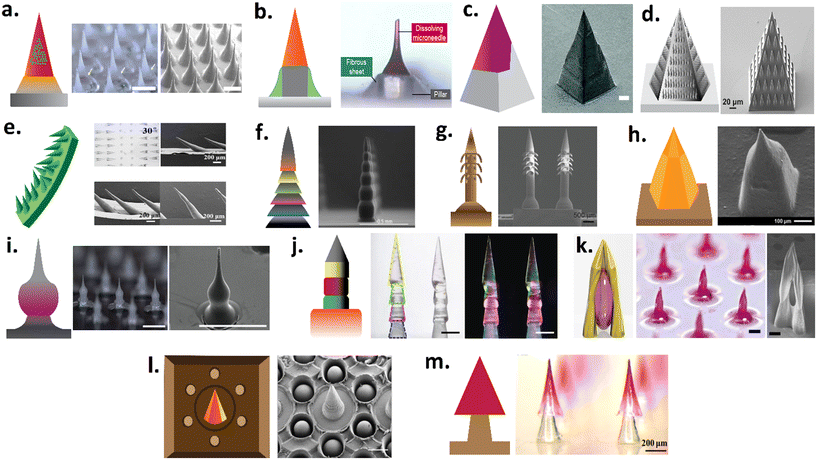 |
| Fig. 4 Examples of various geometrical structures of μNDs. (a) Rapidly separating μND with a bubble like structure between each μND and the patch backplate allowing for snapping off using shearing force,118 (b) rapid implantation of dissolving μND tip into the skin by manual application (tearing through vibration), (c) square pyramidal tips designed to immediately separate from their base, which is a truncated square with a wall on one side, upon skin insertion,119 (d) bug-inspired droplet-like structures ornamented on the lateral sides of pyramidal μND,120 (e) serrated microstructure of mantises' forelegs inspired flexible μNDs on supporting base with clamping-like feature upon skin insertion that has rotational like movement,121 (f) a 3D printed μND consisting of square pyramidal top and multi-faceted bottom with increased surface area for coating drugs,76 (g) backward facing barbed of microhooks of parasites- inspired μND shaft that hooks/interlocks onto tissue upon skin insertion,122 (h) miniature μND tip which tappers down to a wider μND body that achieves both insertion and loading of biotherapeutics,123 (i) a tissue interlocking dissolving μNDs with a sharp tip, wide body, and narrow neck geometry,124 (j) pagoda-like multilayered microstructure of feet or sting of an insect μND shaft for tissue fixation and interlocking,125 (k) a microscale multi-pillar that converges to form sharp and an open cavity for drug trapping,126 (l) inspired by mussel byssi and octopus tentacles suction-cup-structured concave chamber encircling each μND which acts as tissue adhesion cups,127 (m) rapidly separating arrowhead dissolving μND tip mounted on a biodegradable polymer base that breaks and implants into the skin upon insertion.128 Note, figure have been reproduced with permission from ref. 28. | |
The length and number of projections on the μND patches are positively correlated with their drug loading amount because of the simultaneous change in surface area. Increasing the height of the μNDs can increase the maximum load capacity by increasing the surface area, which, on the contrary, can also lead to increased pain.77 The special projection shape may affect the drug loading efficiency more obviously.9 To achieve high drug loading, Caudill et al. compared the surface area and drug loading of square pyramidal μND and faceted μND with horizontal grooves. The results showed that the surface area of faceted μND increased by 21.3% and drug loading increased by 36% compared with Square pyramidal μND (Fig. 4f).76 Uddin et al. designed a cross shaped μNDs with a thousand-fold increase in coating loading (from the microgram level to the milligram level) due to the increase in surface area.89 Li et al. systematically investigated various needle geometries of μND (cone, cone-cylinder, rectangular pyramid, and hexagonal pyramid) for the effective loading and transcutaneous delivery of ovalbumin (OVA).129 They demonstrated that conical needles exhibited structural mechanical integrity, maximum insertion efficiency (∼100% of the needles inserted from one array), and a maximum in vivo immune response even compared to hypodermic needle-based injection. Such findings demonstrate the role of μND geometry and thus the surface area's role in the amount of drug loading and delivery.
Due to the micron size of μNDs, the loading and transdermal delivery of therapeutically relevant doses of drugs is challenging for potent therapeutics.77 Therefore, most clinical trials involving μNDs have so far focused on the transdermal delivery of hormones and vaccines, where only microgram levels are required to produce a therapeutic response.77 Increasing the μND height increases the surface area, thus achieving maximum drug loading, however, that can also lead to heightened pain sensation,77 while increasing the number of μNDs in an array or patch can improve the drug loading, but it consequently increases skin puncture force due to the bed of nails effect.130 Nonetheless, the surface area of the μNDs is an important design criterion that dictates the amount of therapeutic drug that can be loaded.
4. μND coating methods
Although the benefit of room temperature coating may seem advantageous, the issues of insufficient therapeutic delivery have been documented due to the decreased μND sharpness as a result of coating, consequently leading to wastage and loss of delivery into the skin.18,114 Researchers have utilised multiple approaches to overcome therapeutic wastage and loss, variable coating thickness, and inaccurate drug dosage.
Coating approaches, such as dip-coating, gas-jet drying, spray coating, electrohydrodynamic atomisation (EHDA), and ink-jet printing, have been widely explored (Fig. 5).18,19 The simplest coating approach is the dip-coating method, which involves dipping the μND array into a drug formulation and, subsequently, creating a thin film over the μND surface, which eventually dries into solid particles coating the surface of the μNDs.11 This technique offers cost-effectiveness and simplicity but lacks uniformity and precision, leading to drug loss and potentially diminishing the ability of μNDs to penetrate the skin The gas-jet approach disperses a small amount of coating solution over the μND surface while removing and drying the coating solution simultaneously, enabling a faster drying process than dip-coating methods.19 It allows for a faster drying process compared to dip-coating, and by adjusting the gas-jet angle, it ensures proper drying without moving the coating to the base of the μND array. However, it requires precise control of the gas-jet angle and other parameters to avoid defects. Spray coating atomises microdroplet formulations onto μNDs using fluid pressure, forming a thin film over the μNDs.131 This technique provides more controlled and uniform coating compared to dip-coating but may require specialised equipment and fine-tuning to achieve optimal results. The EHDA coating produces an atomised microdroplet formulation using a large electrical potential difference, which is then sprayed onto the μND tips.132 While it produces very fine and uniform droplets for coating, it involves complex instrumentation and high precision requirements. And lastly, ink-jet printing sprays microdroplet formulations using a piezoelectric dispenser onto the μND surface for a more uniform, accurate and reproducible coating.19
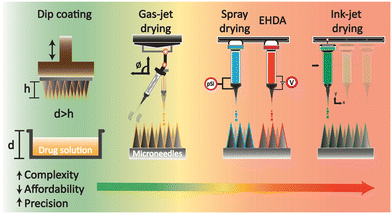 |
| Fig. 5 Schematic illustration of drug-coated strategies on μNDs. 1) Dip coating, 2) gas-jet drying, 3) spray drying and single nozzle EHDA (while shown in the same illustration, they are separate coating strategies), and 4) single nozzle ink-jet drying. Coating strategies were redrawn from ref. 18 and 19. | |
Ink-jet printing can overcome the challenges of uniform coating on the needle tips of CμNDs while ensuring the accuracy of drug dosage.7,133 Additionally, unlike dip coating, which can produce inconsistent and copious coating, ink-jet printing has proven to produce medicated films that are relatively smooth. This smooth coating film eliminates drug loss during μND insertion into the skin.20 Also, this avoids diminishing the ability of μNDs to penetrate the skin.89 However, issues still persist, such as high precision requirements for instruments, unstable uniform coating, and the requirement of more than one coating cycle.11 Despite requiring high precision instruments and possibly needing multiple coating cycles to achieve desired results, ink-jet printing is often considered superior due to its ability to produce uniform and smooth coatings, which ensure accurate drug dosages and maintain the penetration ability of the μNDs. Its advantages in producing high-quality, reproducible coatings make it a preferred method in many applications.
4.1 Considerations for coating formulations when using nozzle technology
Multiple analytical formulas and numerical simulations provide insights into the drop formation mechanisms in the nozzle spray technology, which is the main motive for suppressing unwanted satellite drop formation as well as controlling the properties of the jetted drop.134 Four different drop formation methods have been identified in the literature: i) misting from the breakup of the secondary tail, ii) reproducible slow satellite droplets from pinch-off of the primary tail from the head of the drop, iii) fast reproducible satellite droplets from an initial high acceleration, and iv) Rayleigh breakup of the primary tail.134 These factors become more complicated with the introduction of high-speed drop for Rayleigh break up of long filamentous tail of viscous fluid.135,136 Studies using various simulation methods suggest that the underlying mechanism behind the Rayleigh breakup of long filaments lies in the onset of capillary waves.137–139
The main stages in the droplet formation start with the liquid ejection from the nozzle exit, stretching of the long filamentous liquid thread, pinch-off of the liquid thread from the nozzle exit, contraction of the liquid thread (capillary wave effect due to the surface tension),139 breaking of the liquid thread in the main droplet into one or several satellites, and lastly, the recombination of the main drop with the satellite droplets (provided the satellite droplet has a higher velocity than the main droplet) (Fig. 6a).140–145 The difference in velocity is created by the retraction of the initial thread during the pinch-off process, which is prevalent in low viscous fluid/ink,143 as seen in Fig. 6a.140 The filamentous thread, however, increases with the viscosity of the liquid, which consequently reduces the internal surface energy, thus drastically reducing the possibility of multiple satellite droplets from the original filamentous thread.139 This influences the velocity and the jetting pathway of the tiny satellite droplets significantly if they experience multiple later air drag forces and can drift away from the jetting axis.145 Therefore, to eliminate the formation of multiple satellites, passive measures are taken to reduce the lateral drag force by creating constant air current flow, and secondly, increasing the capillary energy provided by the main satellite, which, then, increases the velocity of the smaller satellites.
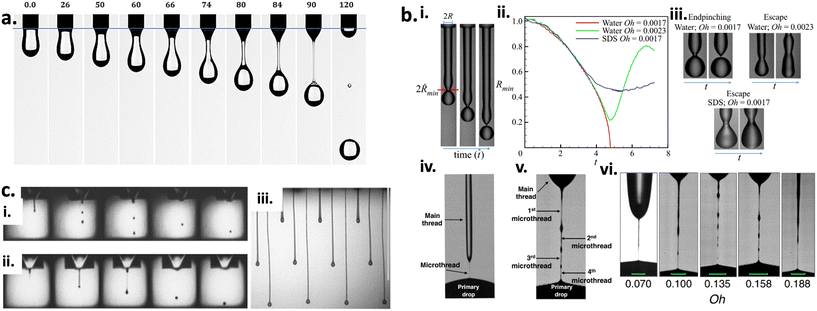 |
| Fig. 6 (a) Demonstration graph of the droplet formation and the evolution of the neck filament of silicon oil in air. The images were captured from the movies at different time in the interval = [0, 120] ms, v0 = 10 mm s−1.139 (b) Experimental results showing endpinching and escape from endpinching.146,147 (i) Image showing the generation or formation of a filament from a nozzle and the evolution in time t of the filament's shape. In the same image, the filament at the earliest time shows twice the radius of the filament 2R when it exits the nozzle and twice the minimum radius of the filament's neck (2 min). (ii) Variation with normalised time vs. normalised minimum filament radius (n = 3). Red curve: water, Oh = 0.0017. Green curve: water, Oh = 0.0023. Blue curve: water plus SDS (labelled as SDS), Oh = 0.0017. (iii) As also shown by the images adjacent to the graph, the filament of water with Oh = 0.0017 endpinches whereas the other two filaments escape from endpinching. Sets of images showing the filaments at two instants in time. (iv) Endpinching: water filament with Oh = 0.0017 prior to and (v) at the incipience of endpinching. (vi) cluster of images showing escape (water): water filament of Oh = 0.0023 appearing on the cusp of breaking, and then shortly after it has escaped endpinching, while the other two images show escape (SDS): surfactant-covered filament of Oh = 0.017 appearing on the verge of endpinching, and then after it has undergone surfactant-driven escape from endpinching. (c) (i) Image sequence illustration from ref. 148 showing the drop formation of ink containing glycerol (50%) and water (50%) created by 50 V pulse/79 μs between frames, while (ii) shows images of drop formation for a non-Newtonian formulation (50 ppm of 300 k PEO in glycerol/water). (iii) Images showing higher polymer concentration formulations leading to the formation of long ligaments. Vertical scale bar set to 1 mm. | |
The main goal of the nozzle spray process is precisely controlling the droplet ejection of a minuscule amount of liquid. One way to achieve that is by adding excipients (surfactant or polymers) to control the droplet ejection from the nozzle, which consequently affects the droplet geometry.134 Surfactants, especially, can change the inks' internal interface energies, which can lead to a complicated interaction between convection (air drying), diffusion onto the target surface, and nozzle or target surface adsorption, and all these factors determine the evolution of the final droplet geometry.134 During the jetting process, the surfactant causes high surface tension in the droplet, which is physically measured via the oscillating droplet.149 For non-Newtonian fluids/ink, the frequency of the oscillation is a property to measure the surface tension of the droplet, whereas the damping of the oscillation is a measure to measure the droplet viscosity (typically a time scale of 1 ms).148,150 For most commercially available fluid/ink, measuring the oscillation frequency properties of the liquid is limited to time scales longer than 100 ms. The viscosity of the droplet is yet another important parameter to optimise to understand the behavior of the ejected droplet. Kamat et al. studied the influence of surfactant (within the filamentous thread post nozzle ejection) on the pinch-off behavior of fluid/ink with both low and high viscosity.146,147 As illustrated in Fig. 6bi–iii,for both viscous and non-viscous filamentous thread, the escape from end-pinching is driven by the action of Marangoni stress originating from the non-uniform spread of surfactant along the surface of the about-to-pinching filamentous thread. Additionally, Marangoni stresses near but not at the pinch-point give rise to the formation of multiple micro-filamentous threads (Fig. 6bv and vi), and this is different from the pinch-off of surfactant-free filamentous thread (Fig. 6biv). In other words, surfactant causes the formation of multiple micro-filamentous threads, thereby eliminating the formation of individual satellite droplets. The nozzle radius also plays an important role in filamentous thread breakage. The studies performed by Kamat et al. involved nozzles with a millimeter-sized orifice. Recent studies140,151 have shown the elimination of higher-order tails by the addition of surfactants. Additionally, higher-order tails consequently lead to the formation of femtoliter droplets, which is a pollution source.
On the other hand, the addition of long-chain macromolecular polymers can dramatically affect the breakup of filamentous threads into satellite droplets as it aids towards the viscoelasticity transforming the fluid from Newtonian fluids to non-Newtonian form.142 The shift in states give rise to complex fluid dynamics behavior, such as shear thinning or strain hardening, either in shear-dominated or extension-dominated flows. Hoath et al. reported the use of shear-thinning PEDOT: PSS polymer to suppress the formation of multiple satellite droplets.148 This polymer solution has been shown to exhibit low viscosity upon exiting the nozzle over a range of exit/jetting speeds while rapidly recovering to a higher viscosity once the jet has formed, thus preventing multiple satellite formation. However, adding a higher concentration of polymer in the fluid/ink can lead to the formation of extremely long filamentous thread.152 Balancing the amount of polymer added to the fluid/ink dictates the fluid dynamics of the filamentous thread formation, which either retracts toward the droplet at the nozzle end or toward the mother droplet away from the nozzle end, as seen in Fig. 6c.152
5. Coating formulation attributes
5.1 Surface wetting properties
Surface wetting is a fundamental principle crucial for achieving coating adherence on μND surfaces.153 A reliable method for assessing substrate wetting involves measuring the contact angle between the wet coating droplet and the μND surface.154,155 This contact angle serves as a direct indicator of the μND surface energy, defined as the angle between the liquid tangent line and the surface to be measured.154–157Table 3 presents the contact angle of water droplets on various commonly used polymer materials utilised in μND fabrication. A smaller contact angle suggests better wetting and, consequently, improved spreading of the coated solution onto the surface.128
Table 3 Contact angle of water droplet on various cured polymer solids. Images reproduced with permission from the reference in the table below
Materials |
Contact angle |
Water-droplet contact on the material |
Ref. |
Polycarbonate |
70–80 |
|
158
|
Acrylonitrile butadiene styrene |
70–80 |
|
159
|
Poly L-lactic acid |
110–120 |
|
160
|
Polyurethane |
70–80 |
|
161
|
Polystyrene |
80–90 |
|
162
|
Polyvinyl alcohol |
30–40 |
|
Polyethylene terephthalate |
50–60 |
|
163
|
Poly(methyl methacrylate) |
60–70 |
|
164
|
Epoxy |
103–110 |
|
165
|
Contact angles are typically divided into static and dynamic. Static contact angle of the coating formulation droplet on a surface determines the hydrophobicity of the contact surfaces,128 whereas dynamic contact angles are measured using the difference between the advancing θa and receding θr contact angles, respectively166,167 (Fig. 7ai and ii), also known as the contact angle hysteresis168,169 (Fig. 7bi and ii). Contact angle hysteresis is a fundamental principle for the adherence of coating formulation on surfaces, without which coating would not be possible on inclined surfaces.168,169 Essentially, a lower contact angle of hysteresis is achieved on high-energy surface, which leads to better wetting and thus improved spreading of coating formulations over the surface.166,170 Conversely, a low-energy surface results in a higher contact angle hysteresis, indicating poor wetting and thus limited spreading of the coated formulation.166,170 Since energy (free Gibbs energy ΔG) is involved in the coating process, the coating droplet has to travel through a series of energy barriers, and as the kinetic energy of the coating droplet reduces due to the surface energy barrier, the coating droplet reaches its static wetting state, thus drying to form a thin film (Fig. 7bi).170 Moreover, surface energy arises from surface roughness, which also dictates the contact angle of hysteresis of the coating formulation, i.e., the rougher the surface, the higher the surface energy, the lower the angle of hysteresis, the better the wetting, and vice versa (Fig. 7bii).171 Therefore, contact angles of coating formulation on surfaces, and the surface energy of surfaces are related to each other, defining coating attributes such as wetting, spreadability, and adhesion. Butt et al. have compiled a comprehensive list of experimental techniques used to measure the contact angle of hysteresis on surface at various angles.166
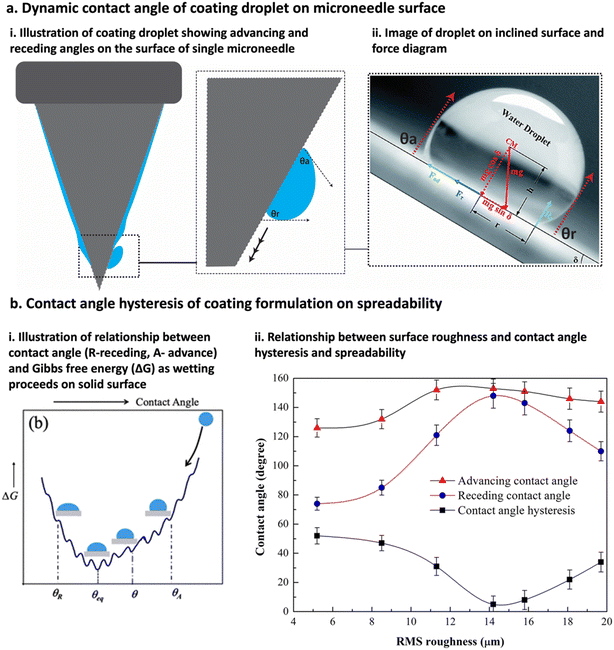 |
| Fig. 7 Contact angle attributes of coating formulation droplet on material surface. (a) Contact angle of coating formulation droplet on inclined surface (μND) is considered as dynamic contact angle as illustrated in (i) and an image of water droplet on inclined surface (ii), illustration was redrawn from and image taken from ref. 166 and 167. (b) Relationship between the contact angle hysteresis (difference between contact angle advance and receding) of coating formulation and surface, and the coating formulation spreadability. (i) Relationship between free Gibbs energy (ΔG) of surface and the coating angle hysteresis determining spreadability, as the coating droplet moves though the surface energy barrier, the kinetic energy of coating droplet reduces thus reducing ΔG.170 (ii) Relationship between surface roughness and contact angle hysteresis determining spreadability, the rougher the surface the lower the angle of hysteresis the better the wetting, and vice versa.171 | |
5.2 Surface energy
The surface energy of surfaces can't be measured directly but through the contact angle of the coating formulation droplet and, recently, through the capillary rise method.157,172 The capillary rise method is common in dip coating of μND that uses the surface energy of the μND tip to pull the coating formulation upon contact.157,172 As a result, a meniscus-like deformation is formed around the contact point between the μND tip and the coating solution (Fig. 8a).172 The shape deformation occurs due to the balance of forces, surface forces (surface tension) and viscoelastic resistance. The shape of the deformation drastically changes as the drying proceeds (Fig. 8b and c),172i.e., when the needle tip touches the coating solution, a large surface rise is formed, here the surface acts as a viscous liquid. As the drying proceeds, the deformation is concentrated near the small region of the tip, and the solvent evaporation of the coating formulation layer on the μND leads to a change from liquid phase to soft viscoelastic phase, eventually turning into dry film with increasing finite viscoelasticity (Fig. 8b and c).172 Additionally, Hsiao et al. successfully demonstrated the dip coating method of μNDs by decreasing the speed of the dipping (Fig. 8d) and increasing the viscosity of the coating solution (Fig. 8e), which increased the amount of drug coated on the surface of the μND without reaching the base of the μND (Fig. 8f).157 Particularly the surface tension of the coating solution and the surface energy of the μND surface play a crucial role in pulling the coating formulation due to capillary action, which is dependent on the viscosity of the formulation, i.e., the more viscous the coating formulation, the longer it takes to reach the base due to the reduced surface tension of the coating formulation. The capillary rise method has proven to be quite versatile in understanding the fundamental thermodynamics of the coating process, however, further studies predicting the dynamic surface wetting and capillary interactions between each μND in an array are necessary to better predict the drug loading amount theoretically.
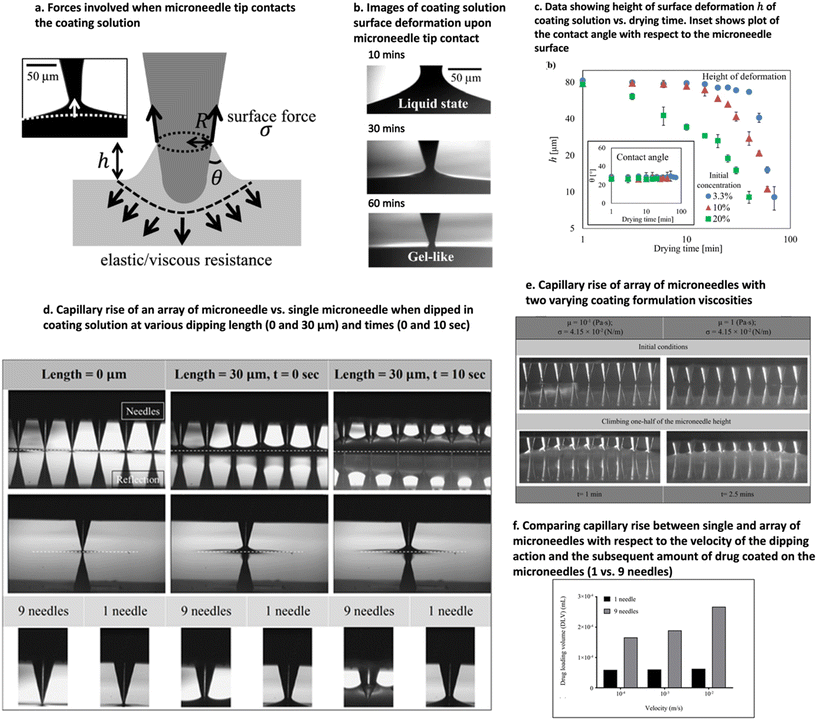 |
| Fig. 8 Illustration of capillary rise method to measure the surface energy of the μND surface. (a) Illustration of the forces involved when μND tip touches the surface of the coating formulation. (b) Elapsed time of the μND tip touching the coating formulation from t = 10–30 min, showing how the viscosity of the coating formulation shape changes over time. (c) Data showing the reduction in the height ‘h’ of the deformation once the tip touched coating formulation surface with increasing drying time. The capillary effect of the coating formulation when the μND tip was dipped into it, showing how the speed of the dipping (d) and the viscosity of the coating formulation (e) affects the amount of drug coated on the μND tips (single vs. array of μND) (f). Images reproduced from ref. 157 and 172. | |
As mentioned previously, the adhesion force of the coating solution onto the μND surface increases with the surface energy of the μNDs.164,173 However, it is imperative to avoid wetting the base of the μND array, as this can lead to inefficient coating and subsequent loss of drug delivery into the skin. To regulate the surface energy of μND surfaces, various surface treatment methods can be employed, including mixing 3D printed materials with,174,175 surface chemical modifications,176,177 organic polymers,176,178 and metal oxide176,179–181 inks. It is essential to consider that the addition of such materials to the ink may render it susceptible to organic solvents and ultraviolet light,140 making it incompatible with traditional photolithography (Stereolithography) but suitable for nozzle technology (fused deposition model – FDM™).141–143 Nonetheless, surface energy is a pivotal functional property that must be considered to achieve efficient adhesion of coating film layers on micro-surfaces.164,173–186
5.3 Interfacial adhesion strength
To achieve uniform coating on surfaces, there must be sufficient bonding strength between the coated solution and the substrate surface. One way to assess the bonding strength is by examining the adhesion properties of the coating solution. There are rheological aspects of the coating formulations that are crucial for the appropriate functioning of coating adhesion. Coating solutions must be optimised in such a manner as to enable the formation of a thin layer/film on the surface of the μNDs, which is a vital feature that can be defined through rheological tests (Fig. 9).172,187–192 This is due to the coating solutions' viscosity properties, which exhibit viscous flow under increasing shear rates, demonstrating a shear-thinning behavior (Fig. 9a), indicating that the coating solution can be easily coated on μND surfaces, formulation can be easily injected.172,190 However, after the coat is adhered to the μND surface (Fig. 9bii), it should recover/retain its original viscoelastic nature (microstructural rearrangement), which is known as the thixotropic phenomenon (Fig. 9biii).172,190 Before the coating, the coating solution has an inherent viscosity (Fig. 9bi), and during the coating process, the coating solution experiences a higher mechanical strain, which results in a decrease in its viscosity (Fig. 9bii), and after the coating process, the coating solution should recover to its original viscosity (Fig. 9biii), demonstrating a thixotropic behavior.172,190 Subsequently, once the coating film adheres to the μND site, it undergoes hardening, which is of paramount importance for coating film layer formation. This transition in the phases should be meticulously controlled, as it should not happen too quickly or too slowly (Fig. 9c).172 Once the coating film is adhered to the μND surface, the interfacial adhesion test, specifically the peel test, can determine the strength of the adhesion to the surface.110,193,194 The larger the strength required to peel off the coating film layer, the stronger the adhesion.110,193,194
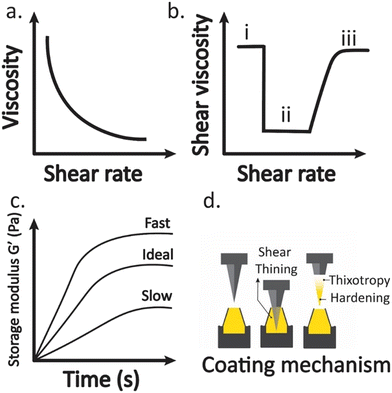 |
| Fig. 9 Illustration of rheology tests used to assess the coating attributes such as: a) shear thinning curve, b) thixotropy behavior under three states (i) initially at rest with low shear rates; (ii) flow under high shear rates; (iii) recovery of coating solution at low shear rates; (c) coating hardening as a function of time, with the coating mechanism process illustrated in (d). The graphs were deduced from the following literature.110,172,187–194 | |
5.4 Additional factors affecting coating of μND surfaces
The main topographical factors that affect coating efficiency are surface roughness, porosity, surface area and energy. Surface energy of the μND topography also complements with the surface energy of the coating solution. These interconnecting concepts aids towards understanding the behavior of coating formulation on μND surfaces, and further studies are required to have a comprehensive understanding of the μND surface coating phenomena. Thus, herein, possible considerations will be highlighted that will help researchers understand the relevant parameters in the coming years.
i. Marangoni effect.
Another relevant thermocapillary phenomenon is the Marangoni effect, which promotes fluid flow at surface interfaces due to surface tension gradients resulting from temperature gradients. Slippery and lubricant-impregnated surfaces have higher surface energy thus creating temperature gradients, which them promotes the migration of coating formulation droplets out of the textured surface, leading to contact between the liquid to be repelled and the solid surface.195 This phenomenon becomes very relevant for coating droplets containing embedded therapeutics and particles (micro/nano) movement on surfaces.196
ii. The interaction between droplets and solid surfaces.
The interaction between droplets and solid surfaces is influenced not only by the properties of the coating formulation fluid but also by surface characteristics such as angle and chemistry. Surface roughness plays a significant role in determining droplet splashing, with factors like the splashing ratio and dynamic contact angle also contributing to this phenomenon. Moreover, chemical reactions and cross-linking can be initiated by droplet impact, presenting opportunities for conducting reactions with minimal volumes or for the controlled formation of materials. For instance, in the context of natural polymeric surface coatings, compound drops, such as aqueous drops of alginate colliding with a calcium-ion-based liquid surface, can lead to the generation of complex shapes and controlled composition.197
iii. Impact dynamics of coating formulation on inclined surfaces.
Most coating formulation droplet impact investigations focus on normal incidence (flat surface) drop impact in still air. Impact dynamic studies should also include other external forces, such as aerodynamic or tangential gravity forces respectively, as well as the tangential velocity of droplets at impact, thus breaking the axisymmetric nature of normal droplet impacts. It was not until recently, attention shifted towards the testing of such conditions, mainly due to the experimental complexity.198–200 The complexity of testing droplet impacts rises as soon as the surface tilt angle increases, and this, consequently, increases the tangential impact velocity while reducing the normal impact velocity.201 Additionally, the droplet consists of an inertial effect, i.e., the tangential kinetic energy which is related to the gravitational effects, i.e., the external force (mgsin
α, whereas α is the tilting angle).201 Proposed alternative solutions include moving the surface on rotating wheels or linearly moving surface targets.202–204 Moreover, quantifying such parameters requires careful setting up of experimental protocols such as: i) synchronising the droplet fall with the surface motion, ii) selection of either spatial resolution or the observation window size that follows the droplet evolution post impact with the moving surface.201 Nevertheless, such experimental setups would shed light on a wider range of impact velocities, as well as help understand the mechanism of droplet disintegration/shedding which is promoted by external forces, such as gravity and aerodynamic drag, which overcome adhesion (capillary action) forces.201
iv. Air effects.
Air effects have been shown to have a strong impact on the initial stages of coating formulation droplet impact onto surfaces. Droplet splashing upon impact can be suppressed by reducing the environmental pressure.205,206 Moreover, the presence of air creates an entrapment of an air disk below the impacting droplet, as air needs to be drained from underneath the drop before the liquid can touch the surface.207 Additionally, a recent study has demonstrated that air compressibility influenced the geometry and dynamics of this air film.208 Future studies should examine the effect of airflow dynamics on droplets' impact close to the bottom interface of the droplet with the surface.
v. Coating uniformity.
Coating uniformity is crucial in the dip coating of μNDs, wherein the capillary rise method utilises surface energy to draw coating formulations onto μND tips. This method offers flexibility in adjusting coating formulation loading by varying parameters like dipping speed and coating solution viscosity. Surface treatment methods are employed to regulate surface energy, enhancing adhesion force between the coating solution and μND surface to optimise coating efficiency and drug delivery while ensuring uniformity. Achieving uniform coating relies on sufficient bonding strength between the coated solution and substrate surface, where rheological properties such as shear-thinning behavior and thixotropic nature play a pivotal role in proper coating formation and hardening. Interfacial adhesion tests, including peel tests, serve as valuable tools to assess the strength of adhesion between the coating film layer and the surface, ensuring optimal coating performance.
6. Conclusion
In conclusion, the integration of 3D printed μNDs with specific surface characteristics, such as roughness, surface area, and porosity, presents a promising avenue for enhancing the performance and efficacy of transdermal drug delivery systems. By carefully controlling these surface parameters, it becomes possible to modulate various aspects of the μND-skin interaction, including drug diffusion kinetics and tissue penetration efficiency. Moreover, the selection and optimisation of coating formulations play a crucial role in dictating the overall performance of coated μNDs. Attributes such as coating uniformity, contact angle, surface energy, viscosity, and surface tension significantly influence the coating process and subsequent drug release kinetics. Achieving uniform coating distribution and proper wetting properties are essential for ensuring consistent drug delivery across different skin types and application scenarios. Future research efforts should focus on further elucidating the relationships between 3D printed μND surface properties and coating formulation attributes. By gaining a deeper understanding of these interdependencies, researchers can develop more sophisticated strategies for optimising transdermal drug delivery systems, ultimately leading to enhanced therapeutic outcomes and patient experiences.
Data availability
This article is a review of existing literature, and as such, no new data were generated or analysed in this study. All data discussed are available from the original publications cited within the article.
Author contributions
Yanling Yan and Masood Ali wrote the original manuscript draft and editing. Ayyah Abdoh edited the manuscript. Yousuf Mohammed conceptualised the idea, supervised and provided support with manuscript editing and resources, submission, and management of the article.
Conflicts of interest
The authors declare that they have no known competing financial interests or personal relationships that could have appeared to influence the work reported in this paper.
Acknowledgements
This work was based on a part of M. A.'s PhD thesis and Y. Y.'s Master thesis. M. A. is thankful and acknowledges the support of RTP (Research Training Program) scholarship from the University of Queensland. M. A., Y. Y., A. A. and Y. M. are thankful to the Translational Research Institute (TRI) for the resources and infrastructure for this review article.
References
- I. N. Haridass, J. C. J. Wei and Y. H. Mohammed,
et al., Cellular metabolism and pore lifetime of human skin following microprojection array mediation, J. Controlled Release, 2019, 306, 59–68 CrossRef CAS PubMed.
- A. M. Rodgers, M. T. C. McCrudden and E. M. Vincente-Perez,
et al., Design and characterisation of a dissolving microneedle patch for intradermal vaccination with heat-inactivated bacteria: A proof of concept study, Int. J. Pharm., 2018, 549(1), 87–95 CrossRef CAS PubMed.
- Z. Faraji Rad, P. D. Prewett and G. J. Davies, An overview of microneedle applications, materials, and fabrication methods, Beilstein J. Nanotechnol., 2021, 12, 1034–1046 CrossRef PubMed.
- G.-S. Liu, Y. Kong and Y. Wang,
et al., Microneedles for transdermal diagnostics: Recent advances and new horizons, Biomaterials, 2020, 232, 119740 CrossRef CAS PubMed.
- M. Ali, S. Namjoshi and H. A. E. Benson,
et al., Dissolvable polymer microneedles for drug delivery and diagnostics, J. Controlled Release, 2022, 347, 561–589 CrossRef CAS PubMed.
- S. Henry, D. V. McAllister and M. G. Allen,
et al., Microfabricated microneedles: A novel approach to transdermal drug delivery, J. Pharm. Sci., 1998, 87(8), 922–925, DOI:10.1021/js980042+.
- W. S. Shim, Y. M. Hwang and S. G. Park,
et al., Role of Polyvinylpyrrolidone in Dissolving Microneedle for Efficient Transdermal Drug Delivery: In vitro and Clinical Studies, Bull. Korean Chem. Soc., 2018, 39(6), 789–793, DOI:10.1002/bkcs.11476.
- M. Avcil and A. Çelik, Microneedles in Drug Delivery: Progress and Challenges, Micromachines, 2021, 12(11), 1321 CrossRef PubMed.
- N. W. Kang, S. Kim and J. Y. Lee,
et al., Microneedles for drug delivery: recent advances in materials and geometry for preclinical and clinical studies, Expert Opin. Drug Delivery, 2021, 18(7), 929–947 CrossRef CAS PubMed.
- S. N. Economidou, C. P. Pissinato Pere and M. Okereke,
et al., Optimisation of Design and Manufacturing Parameters of 3D Printed Solid Microneedles for Improved Strength, Sharpness, and Drug Delivery, Micromachines, 2021, 12(2), 117 CrossRef PubMed.
- R. S. J. Ingrole and H. S. Gill, Microneedle Coating Methods: A Review with a Perspective, J. Pharmacol. Exp. Ther., 2019, 370(3), 555–569 CrossRef CAS PubMed.
- I. Mansoor, H. A. Eassa and K. H. A. Mohammed,
et al., Microneedle-Based Vaccine Delivery: Review of an Emerging Technology, AAPS PharmSciTech, 2022, 23(4), 103 CrossRef CAS PubMed.
- M. Sirbubalo, A. Tucak and K. Muhamedagic,
et al., 3D Printing-A “Touch-Button” Approach to Manufacture Microneedles for Transdermal Drug Delivery, Pharmaceutics, 2021, 13(7), 924 CrossRef CAS PubMed.
- J. Xu, D. Xu and X. Xuan,
et al., Advances of Microneedles in Biomedical Applications, Molecules, 2021, 26(19), 5912 CrossRef CAS PubMed.
- E. Kim, G. Erdos and S. Huang,
et al., Microneedle array delivered recombinant coronavirus vaccines: Immunogenicity and rapid translational development, EBioMedicine, 2020, 55, 102743 CrossRef CAS PubMed.
- Y. Yin, W. Su and J. Zhang,
et al., Separable Microneedle Patch to Protect and Deliver DNA Nanovaccines Against COVID-19, ACS Nano, 2021, 15(9), 14347–14359 CrossRef CAS PubMed.
- A. V. Boopathy, A. Mandal and D. W. Kulp,
et al., Enhancing humoral immunity via sustained-release implantable microneedle patch vaccination, Proc. Natl. Acad. Sci. U. S. A., 2019, 116(33), 16473–16478 CrossRef CAS PubMed.
- K. van der Maaden, W. Jiskoot and J. Bouwstra, Microneedle technologies for (trans)dermal drug and vaccine delivery, J. Controlled Release, 2012, 161(2), 645–655 CrossRef CAS PubMed.
- R. Haj-Ahmad, H. Khan and M. S. Arshad,
et al., Microneedle Coating Techniques for Transdermal Drug Delivery, Pharmaceutics, 2015, 7(4), 486–502 CrossRef CAS PubMed.
- S. N. Economidou, C. P. P. Pere and A. Reid,
et al., 3D printed microneedle patches using stereolithography (SLA) for intradermal insulin delivery, Mater. Sci. Eng., C, 2019, 102, 743–755 CrossRef CAS PubMed.
- M. J. Uddin, N. Scoutaris and P. Klepetsanis,
et al., Inkjet printing of transdermal microneedles for the delivery of anticancer agents, Int. J. Pharm., 2015, 494(2), 593–602 CrossRef CAS PubMed.
- P. Cheppudira Thimmaiah, A. K. Panda and U. K. Pandey,
et al., A New Approach to Compute the Porosity and Surface Roughness of Porous Coated Capillary-Assisted Low Pressure Evaporators, Sci. Rep., 2018, 8(1), 11708 CrossRef PubMed.
- R. F. Donnelly, T. R. Raj Singh and A. D. Woolfson, Microneedle-based drug delivery systems: microfabrication, drug delivery, and safety, Drug Delivery, 2010, 17(4), 187–207 CrossRef CAS PubMed.
- S. Henry, D. V. McAllister and M. G. Allen,
et al., Microfabricated microneedles: a novel approach to transdermal drug delivery, J. Pharm. Sci., 1998, 87(8), 922–925 CrossRef CAS PubMed.
- J. A. Matriano, M. Cormier and J. Johnson,
et al., Macroflux® microprojection array patch technology: a new and efficient approach for intracutaneous immunization, Pharm. Res., 2002, 19, 63–70 CrossRef CAS PubMed.
- E. M. Cahill and E. D. O'Cearbhaill, Toward biofunctional microneedles for stimulus responsive drug delivery, Bioconjugate Chem., 2015, 26(7), 1289–1296 CrossRef CAS PubMed.
- M. Ali, S. Namjoshi and H. A. E. Benson,
et al., Skin biomechanics: Breaking the dermal barriers with microneedles, Nano TransMed, 2022, 1(1), e9130002 CrossRef.
- M. Ali, S. Namjoshi and H. A. E. Benson,
et al., Dissolvable polymer microneedles for drug delivery and diagnostics, J. Controlled Release, 2022, 347, 561–589 CrossRef CAS PubMed.
- Z. Chen, R. Ye and J. Yang,
et al., Rapidly Fabricated Microneedle Arrays Using Magnetorheological Drawing Lithography for Transdermal Drug Delivery, ACS Biomater. Sci. Eng., 2019, 5(10), 5506–5513 CrossRef CAS PubMed.
- J. Jeong, J. Park and S. Lee, 3D printing fabrication process for fine control of microneedle shape, Micro Nano Syst. Lett., 2023, 11(1), 1 CrossRef.
- Z. Chen, L. Ren and J. Li,
et al., Rapid fabrication of microneedles using magnetorheological drawing lithography, Acta Biomater., 2018, 65, 283–291 CrossRef PubMed.
- H. Yang, S. Kim and G. Kang,
et al., Centrifugal Lithography: Self-Shaping of Polymer Microstructures Encapsulating Biopharmaceutics by Centrifuging Polymer Drops, Adv. Healthcare Mater., 2017, 6(19), 1700326 CrossRef PubMed.
- H. Takahashi, Y. Jung Heo and N. Arakawa,
et al., Scalable fabrication of microneedle arrays via spatially controlled UV exposure, Microsyst. Nanoeng., 2016, 2(1), 16049 CrossRef CAS PubMed.
- S. L. Silvestre, D. Araújo and A. C. Marques,
et al., Microneedle Arrays of Polyhydroxyalkanoate by Laser-Based Micromolding Technique, ACS Appl. Bio Mater., 2020, 3(9), 5856–5864 CrossRef CAS PubMed.
- R. K. Andranilla, Q. K. Anjani and P. Hartrianti,
et al., Fabrication of dissolving microneedles for transdermal delivery of protein and peptide drugs: polymer materials and solvent casting micromoulding method, Pharm. Dev. Technol., 2023, 28(10), 1016–1031 CrossRef CAS PubMed.
- S. C. Ligon, R. Liska and J. Stampfl,
et al., Polymers for 3D Printing and Customized Additive Manufacturing, Chem. Rev., 2017, 117(15), 10212–10290 CrossRef CAS PubMed.
- T. D. Ngo, A. Kashani and G. Imbalzano,
et al., Additive manufacturing (3D printing): A review of materials, methods, applications and challenges, Composites, Part B, 2018, 143, 172–196 CrossRef CAS.
- I. Xenikakis, M. Tzimtzimis and K. Tsongas,
et al., Fabrication and finite element analysis of stereolithographic 3D printed microneedles for transdermal delivery of model dyes across human skin in vitro, Eur. J. Pharm. Sci., 2019, 137, 104976 CrossRef CAS PubMed.
- R. Ali, P. Mehta and M. S. Arshad,
et al., Transdermal Microneedles—A Materials Perspective, AAPS PharmSciTech, 2019, 21(1), 12 CrossRef CAS PubMed.
- A. Ovsianikov, B. Chichkov and P. Mente,
et al., Two photon polymerization of polymer-ceramic hybrid materials for transdermal drug delivery, Int. J. Appl. Ceram. Technol., 2007, 4(1), 22–29 CrossRef CAS.
- S. Liu, G. Roeder and G. Aygun,
et al., Simulation of 3D inclined/rotated UV lithography and its application to microneedles, Optik, 2012, 123(10), 928–931 CrossRef CAS.
- Y.-K. Yoon, J.-H. Park and M. G. Allen, Multidirectional UV lithography for complex 3-D MEMS structures, J. Microelectromech. Syst., 2006, 15(5), 1121–1130 CrossRef.
- H. Yang, S. Kim and G. Kang,
et al., Centrifugal Lithography: Self-Shaping of Polymer Microstructures Encapsulating Biopharmaceutics by Centrifuging Polymer Drops, Adv. Healthcare Mater., 2017, 6(19), 1700326 CrossRef PubMed.
- S. Lee, S. Fakhraei Lahiji and J. Jang,
et al., Micro-Pillar Integrated Dissolving Microneedles for Enhanced Transdermal Drug Delivery, Pharmaceutics, 2019, 11(8), 402 CrossRef CAS PubMed.
- F. Ruggiero, R. Vecchione and S. Bhowmick,
et al., Electro-drawn polymer microneedle arrays with controlled shape and dimension, Sens. Actuators, B, 2018, 255, 1553–1560 CrossRef CAS.
- R. Vecchione, S. Coppola and E. Esposito,
et al., Electro-Drawn Drug-Loaded Biodegradable Polymer Microneedles as a Viable Route to Hypodermic Injection, Adv. Funct. Mater., 2014, 24(23), 3515–3523 CrossRef CAS.
- C. K. Choi, K. J. Lee and Y. N. Youn,
et al., Spatially discrete thermal drawing of biodegradable microneedles for vascular drug delivery, Eur. J. Pharm. Biopharm., 2013, 83(2), 224–233 CrossRef CAS PubMed.
- L. Cai, Z. Luo and H. Chen,
et al., Lithographic Microneedle-Motors from Multimodal Microfluidics for Cargo Delivery, Small, 2023, 19(12), 2206108 CrossRef CAS PubMed.
- J. Y. Tan, Y. Li and F. Chamani,
et al., Experimental Validation of Diffraction Lithography for Fabrication of Solid Microneedles, Materials, 2022, 15(24), 8934 CrossRef CAS PubMed.
-
J. Y. Tan, A. Kim and J. J. Kim, Fabrication and Characterization of Hollow Microneedle Array Using Diffraction UV Lithography, 2021 21st International Conference on Solid-State Sensors, Actuators and Microsystems (Transducers), 2021 Search PubMed.
- Z. Faraji Rad, R. E. Nordon and C. J. Anthony,
et al., High-fidelity replication of thermoplastic microneedles with open microfluidic channels, Microsyst. Nanoeng., 2017, 3(1), 1–11 Search PubMed.
- H. Kathuria, K. Kang and J. Cai,
et al., Rapid microneedle fabrication by heating and photolithography, Int. J. Pharm., 2020, 575, 118992 CrossRef CAS PubMed.
- H. Pitakjakpipop, R. Rajan and K. Tantisantisom,
et al., Facile Photolithographic Fabrication of Zwitterionic Polymer Microneedles with Protein Aggregation Inhibition for Transdermal Drug Delivery, Biomacromolecules, 2022, 23(1), 365–376 CrossRef CAS PubMed.
- H. Roh, Y. J. Yoon and J. S. Park,
et al., Fabrication of High-Density Out-of-Plane Microneedle Arrays with Various Heights and Diverse Cross-Sectional Shapes, Nano-Micro Lett., 2021, 14(1), 24 CrossRef PubMed.
- Y. Han, J. Li and T. Chen,
et al., Modern microelectronics and microfluidics on microneedles, Analyst, 2023, 148(19), 4591–4615, 10.1039/D3AN01045G.
- A. Malek-Khatabi, Z. Faraji Rad and M. Rad-Malekshahi,
et al., Development of dissolvable microneedle patches by CNC machining and micromolding for drug delivery, Mater. Lett., 2023, 330, 133328 CrossRef CAS.
- K. J. Krieger, N. Bertollo and M. Dangol,
et al., Simple and customizable method for fabrication of high-aspect ratio microneedle molds using low-cost 3D printing, Microsyst. Nanoeng., 2019, 5(1), 42 CrossRef PubMed.
- N. Wang, Q. Wang and S. Xu,
et al., Mechanical Stability of PDMS-Based Micro/Nanotextured Flexible Superhydrophobic Surfaces under External Loading, ACS Appl. Mater. Interfaces, 2019, 11(51), 48583–48593 CrossRef CAS PubMed.
- M. Taşdemir, F. Şenaslan and A. Çelik, Investigation of corrosion and thermal behavior of PU–PDMS-coated AISI 316L, e-Polym., 2021, 21(1), 355–365 CrossRef.
- T. S. Radhakrishnan, Thermal degradation of poly(dimethylsilylene) and poly(tetramethyldisilylene-co-styrene), J. Appl. Polym. Sci., 2006, 99(5), 2679–2686 CrossRef CAS.
- M. J. Kim, S. C. Park and B. Rizal,
et al., Fabrication of circular obelisk-type multilayer microneedles using micro-milling and spray deposition, Front. Bioeng. Biotechnol., 2018, 6, 54 CrossRef PubMed.
- U. Detamornrat, E. McAlister and A. R. J. Hutton,
et al., The Role of 3D Printing Technology in Microengineering of Microneedles, Small, 2022, 18(18), 2106392 CrossRef CAS PubMed.
- S. R. Dabbagh, M. R. Sarabi and R. Rahbarghazi,
et al., 3D-printed microneedles in biomedical applications, iScience, 2021, 24(1), 102012 CrossRef CAS PubMed.
- M. A. Luzuriaga, D. R. Berry and J. C. Reagan,
et al., Biodegradable 3D printed polymer microneedles for transdermal drug delivery, Lab Chip, 2018, 18(8), 1223–1230, 10.1039/C8LC00098K.
- A. Kjar and Y. Huang, Application of Micro-Scale 3D Printing in Pharmaceutics, Pharmaceutics, 2019, 11(8), 390 CrossRef CAS PubMed.
- S. Choo, S. Jin and J. Jung, Fabricating High-Resolution and High-Dimensional Microneedle Mold through the Resolution Improvement of Stereolithography 3D Printing, Pharmaceutics, 2022, 14(4), 766 CrossRef PubMed.
- C. P. P. Pere, S. N. Economidou and G. Lall,
et al., 3D printed microneedles for insulin skin delivery, Int. J. Pharm., 2018, 544(2), 425–432 CrossRef CAS PubMed.
- H. Quan, T. Zhang and H. Xu,
et al., Photo-curing 3D printing technique and its challenges, Bioact. Mater., 2020, 5(1), 110–115 Search PubMed.
- E. Mathew, G. Pitzanti and A. L. Gomes Dos Santos,
et al., Optimization of Printing Parameters for Digital Light Processing 3D Printing of Hollow Microneedle Arrays, Pharmaceutics, 2021, 13(11), 1837 CrossRef CAS PubMed.
- W. Yao, D. Li and Y. Zhao,
et al., 3D Printed Multi-Functional Hydrogel Microneedles Based on High-Precision Digital Light Processing, Micromachines, 2019, 11(1), 17 CrossRef PubMed.
- N. El-Sayed, L. Vaut and M. Schneider, Customized fast-separable microneedles prepared with the aid of 3D printing for nanoparticle delivery, Eur. J. Pharm. Biopharm., 2020, 154, 166–174 CrossRef CAS PubMed.
- A. Ovsianikov, B. Chichkov and P. Mente,
et al., Two photon polymerization of polymer–ceramic hybrid materials for transdermal drug delivery, Int. J. Appl. Ceram. Technol., 2007, 4(1), 22–29 CrossRef CAS.
- I. Xenikakis, M. Tzimtzimis and K. Tsongas,
et al., Fabrication and finite element analysis of stereolithographic 3D printed microneedles for transdermal delivery of model dyes across human skin in vitro, Eur. J. Pharm. Sci., 2019, 137, 104976 CrossRef CAS PubMed.
- A. Ovsianikov, B. Chichkov and P. Mente,
et al., Two Photon Polymerization of Polymer–Ceramic Hybrid Materials for Transdermal Drug Delivery, Int. J. Appl. Ceram. Technol., 2007, 4(1), 22–29 CrossRef CAS.
- I. Xenikakis, K. Tsongas and E. K. Tzimtzimis,
et al., Fabrication of hollow microneedles using liquid crystal display (LCD) vat polymerization 3D printing technology for transdermal macromolecular delivery, Int. J. Pharm., 2021, 597, 120303 CrossRef CAS PubMed.
- C. Caudill, J. L. Perry and K. Iliadis,
et al., Transdermal vaccination via 3D-printed microneedles induces potent humoral and cellular immunity, Proc. Natl. Acad. Sci. U. S. A., 2021, 118(39), e2102595118 CrossRef CAS PubMed.
- A. R. Johnson, C. L. Caudill and J. R. Tumbleston,
et al., Single-Step Fabrication of Computationally Designed Microneedles by Continuous Liquid Interface Production, PLoS One, 2016, 11(9), e0162518 CrossRef PubMed.
- Z. Faraji Rad, R. E. Nordon and C. J. Anthony,
et al., High-fidelity replication of thermoplastic microneedles with open microfluidic channels, Microsyst. Nanoeng., 2017, 3(1), 17034 CrossRef CAS PubMed.
- A. Masood, N. Sarika and A. E. B. Heather,
et al., Skin biomechanics: Breaking the dermal barriers with microneedles, Nano TransMed, 2022, 1(1), e9130002 CrossRef.
- S. C. L. Fischer, S. Boyadzhieva and R. Hensel,
et al., Adhesion and relaxation of a soft elastomer on surfaces with skin like roughness, J. Mech. Behav. Biomed. Mater., 2018, 80, 303–310 CrossRef CAS PubMed.
- K. A. Vella, J. Buhagiar and G. Cassar,
et al., The Effect of a Duplex Surface Treatment on the Corrosion and Tribocorrosion Characteristics of Additively Manufactured Ti-6Al-4V, Materials, 2023, 16(5), 2098 CrossRef CAS PubMed.
- O. Lupicka and B. Warcholinski, The Adhesion of CrN Thin Films Deposited on Modified 42CrMo4 Steel, Adv. Mater. Sci. Eng., 2017, 2017, 4064208 CrossRef.
- S. G. Croll, Surface roughness profile and its effect on coating adhesion and corrosion protection: A review, Prog. Org. Coat., 2020, 148, 105847 CrossRef CAS.
- G. Gold and K. Helmreich, A Physical Surface Roughness Model and Its Applications, IEEE Trans. Microwave Theory Tech., 2017, 65(10), 3720–3732 Search PubMed.
- B. Xiong, J. Li and C. He,
et al., Effect of pore morphology and surface roughness on wettability of porous titania films, Mater. Res. Express, 2020, 7(11), 115013 CrossRef CAS.
- N. Naat, Y. Boutar and S. Naïmi,
et al., Influence of bio-inspired surface texture of additively manufactured 17-4 PH stainless steel adherends on the strength of adhesively bonded joints, Int. J. Adhes. Adhes., 2023, 126, 103478 CrossRef CAS.
- K. Zhou, G. Xiao and Y. Huang, Fabricating physicochemical microstructures with super hydrophilicity on Cf/SiC composites surface via picosecond-laser induced ablation, Ceram. Int., 2023, 49(21), 34291–34302 CrossRef CAS.
- V. Barreau, R. Hensel and N. K. Guimard,
et al., Fibrillar Elastomeric Micropatterns Create Tunable Adhesion Even to Rough Surfaces, Adv. Funct. Mater., 2016, 26(26), 4687–4694 CrossRef CAS.
- M. J. Uddin, N. Scoutaris and S. N. Economidou,
et al., 3D printed microneedles for anticancer therapy of skin tumours, Mater. Sci. Eng., C, 2020, 107, 110248 CrossRef CAS PubMed.
- S. Liu, C. Wang and S. Du,
et al., 3D morphology reconstruction of rock joints from 2D profile measurement by a profilograph, Measurement, 2022, 203, 112008 CrossRef.
- J. Liu, E. Lu and H. Yi,
et al., A new surface roughness measurement method based on a color distribution statistical matrix, Measurement, 2017, 103, 165–178 CrossRef.
- T. Jeyapoovan and M. Murugan, Surface roughness classification using image processing, Measurement, 2013, 46(7), 2065–2072 CrossRef.
- Y. Zou and J. Malzbender, Development and optimization of porosity measurement techniques, Ceram. Int., 2016, 42(2, Part A), 2861–2870 CrossRef CAS.
- A. Sola and A. Nouri, Microstructural porosity in additive manufacturing: The formation and detection of pores in metal parts fabricated by powder bed fusion, J. Adv. Manuf. Process., 2019, 1(3), e10021, DOI:10.1002/amp2.10021.
- E. M. Cahill, S. Keaveney and V. Stuettgen,
et al., Metallic microneedles with interconnected porosity: A scalable platform for biosensing and drug delivery, Acta Biomater., 2018, 80, 401–411 CrossRef CAS PubMed.
- G. Gao, L. Zhang and Z. Li,
et al., Porous Microneedles for Therapy and Diagnosis: Fabrication and Challenges, ACS Biomater. Sci. Eng., 2023, 9(1), 85–105 CrossRef CAS PubMed.
-
F. Dullien, Porous Media Fluid Transport and Pore Structure, Elsevier Science, 2012 Search PubMed.
- Porosity Measurement in Carbon-Fiber-Reinforced Polymer Composite Through Optical Microscopy Using ImageJ Software, Advances in Mechanical and Power Engineering, ed. F. Monticeli, H. Voorwald and M. O. Cioffi, Springer International Publishing, Cham, 2023 Search PubMed.
- S. G. Croll and S. A. Payne, Quantifying abrasive-blasted surface roughness profiles using scanning electron microscopy, J. Coat. Technol. Res., 2020, 17(5), 1231–1242 CrossRef CAS.
- E. Jonda, L. Łatka and W. Pakieła, Microstructure and Selected Properties of Cr(3)C(2)-NiCr Coatings Obtained by HVOF on Magnesium Alloy Substrates, Materials, 2020, 13(12), 2775 CrossRef CAS PubMed.
- M. A. Quetzeri-Santiago, A. A. Castrejón-Pita and J. R. Castrejón-Pita, The Effect of Surface Roughness on the Contact Line and Splashing Dynamics of Impacting Droplets, Sci. Rep., 2019, 9(1), 15030 CrossRef PubMed.
- C. Qiu, C. Panwisawas and M. Ward,
et al., On the role of melt flow into the surface structure and porosity development during selective laser melting, Acta Mater., 2015, 96, 72–79 CrossRef CAS.
- J. P. B. van Dam, S. T. Abrahami and A. Yilmaz,
et al., Effect of surface roughness and chemistry on the adhesion and durability of a steel-epoxy adhesive interface, Int. J. Adhes. Adhes., 2020, 96, 102450 CrossRef CAS.
- S. Zouari, H. Ghorbel and C. Langlade,
et al., Painting Process Design and Characterization of Polymer Coatings on Brass, J. Mater. Eng. Perform., 2022, 31(1), 180–190 CrossRef CAS.
- Z. Zhu, S. Lou and C. Majewski, Characterisation and correlation of areal surface texture with processing parameters and porosity of High Speed Sintered parts, Addit. Manuf., 2020, 36, 101402 Search PubMed.
- K. Markandan and C. Q. Lai, Enhanced mechanical properties of 3D printed graphene-polymer composite lattices at very low graphene concentrations, Composites, Part A, 2020, 129, 105726 CrossRef CAS.
- S. Carmignato, V. Aloisi and F. Medeossi,
et al., Influence of surface roughness on computed tomography dimensional measurements, CIRP Ann., 2017, 66(1), 499–502 CrossRef.
- N. Fusi and J. Martinez-Martinez, Mercury porosimetry as a tool for improving quality of micro-CT images in low porosity carbonate rocks, Eng. Geol., 2013, 166, 272–282 CrossRef.
- P. Pei, F. Yang and J. Liu,
et al., Composite-dissolving microneedle patches for chemotherapy and photothermal therapy in superficial tumor treatment, Biomater. Sci., 2018, 6(6), 1414–1423 RSC.
- A. Ullah, C. M. Kim and G. M. Kim, Porous polymer coatings on metal microneedles for enhanced drug delivery, R. Soc. Open Sci., 2018, 5(4), 171609 CrossRef PubMed.
- H. Vallhov, W. Xia and H. Engqvist,
et al., Bioceramic microneedle arrays are able to deliver OVA to dendritic cells in human skin, J. Mater. Chem. B, 2018, 6(42), 6808–6816 RSC.
- S. A. Coulman, D. Barrow and A. Anstey,
et al., Minimally invasive cutaneous delivery of macromolecules and plasmid DNA via microneedles, Curr. Drug Delivery, 2006, 3(1), 65–75 CrossRef CAS PubMed.
- H. S. Gill and M. R. Prausnitz, Coating Formulations for Microneedles, Pharm. Res., 2007, 24(7), 1369–1380 CrossRef CAS PubMed.
- Y. C. Kim, J. H. Park and M. R. Prausnitz, Microneedles for drug and vaccine delivery, Adv. Drug Delivery Rev., 2012, 64(14), 1547–1568 CrossRef CAS PubMed.
- S. De Martino, M. Battisti and F. Napolitano,
et al., Effect of microneedles shape on skin penetration and transdermal drug administration, Biomater. Adv., 2022, 142, 213169 CrossRef CAS PubMed.
- R. F. Donnelly, T. R. Raj Singh and A. D. Woolfson, Microneedle-based drug delivery systems: microfabrication, drug delivery, and safety, Drug Delivery, 2010, 17(4), 187–207 CrossRef CAS PubMed.
- E. Larrañeta, R. E. M. Lutton and A. D. Woolfson,
et al., Microneedle arrays as transdermal and intradermal drug delivery systems: Materials science, manufacture and commercial development, Mater. Sci. Eng., R, 2016, 104, 1–32 CrossRef.
- W. Li, J. Tang and R. N. Terry,
et al., Long-acting reversible contraception by effervescent microneedle patch, Sci. Adv., 2019, 5(11), eaaw8145 CrossRef CAS PubMed.
- H. Jun, M. H. Ahn and I. J. Choi,
et al., Immediate separation of microneedle tips from base array during skin insertion for instantaneous drug delivery, RSC Adv., 2018, 8(32), 17786–17796 RSC.
- C. Plamadeala, S. R. Gosain and F. Hischen,
et al., Bio-inspired microneedle design for efficient drug/vaccine coating, Biomed. Microdevices, 2019, 22(1), 8 CrossRef PubMed.
- X. Zhang, F. Wang and Y. Yu,
et al., Bio-inspired clamping microneedle arrays from flexible ferrofluid-configured moldings, Sci. Bull., 2019, 64(15), 1110–1117 CrossRef CAS PubMed.
- D. Han, R. S. Morde and S. Mariani,
et al., 4D Printing of a Bioinspired Microneedle Array with Backward-Facing Barbs for Enhanced Tissue Adhesion, Adv. Funct. Mater., 2020, 30(11), 1909197 CrossRef CAS.
- J. W. Lee, S.-O. Choi and E. I. Felner,
et al., Dissolving Microneedle Patch for Transdermal Delivery of Human Growth Hormone, Small, 2011, 7(4), 531–539 CrossRef CAS PubMed.
- J. Ohn, M. Jang and B. M. Kang,
et al., Dissolving Candlelit Microneedle for Chronic Inflammatory Skin Diseases, Adv. Sci., 2021, 8(14), 2004873 CrossRef PubMed.
- X. Zhang, G. Chen and L. Cai,
et al., Bioinspired pagoda-like microneedle patches with strong fixation and hemostasis capabilities, Chem. Eng. J., 2021, 414, 128905 CrossRef CAS.
- J. Lim, D. Tahk and J. Yu,
et al., Design rules for a tunable merged-tip microneedle, Microsyst. Nanoeng., 2018, 4, 29–29 CrossRef PubMed.
- X. Zhang, G. Chen and Y. Yu,
et al., Bioinspired Adhesive and Antibacterial Microneedles for Versatile Transdermal Drug Delivery, Research., 2020, 2020, 3672120 CAS.
- D. D. Zhu, Q. L. Wang and X. B. Liu,
et al., Rapidly separating microneedles for transdermal drug delivery, Acta Biomater., 2016, 41, 312–319 CrossRef CAS PubMed.
- Y. Li, X. Hu and Z. Dong,
et al., Dissolving Microneedle Arrays with Optimized Needle Geometry for Transcutaneous Immunization, Eur. J. Pharm. Sci., 2020, 151, 105361 CrossRef CAS PubMed.
- O. Olatunji, D. B. Das and M. J. Garland,
et al., Influence of Array Interspacing on the Force Required for Successful Microneedle Skin Penetration: Theoretical and Practical Approaches, J. Pharm. Sci., 2013, 102(4), 1209–1221 CrossRef CAS PubMed.
- X. Ning, C. Wiraja and D. C. S. Lio,
et al., A Double-Layered Microneedle Platform Fabricated through Frozen Spray-Coating, Adv. Healthcare Mater., 2020, 9(10), 2000147 CrossRef CAS PubMed.
- P. Rai, N. Gautam and H. Chandra, An Experimental Approach of Generation of Micro/Nano Scale Liquid Droplets by Electrohydrodynamic Atomization (EHDA) Process, Mater. Today: Proc., 2017, 4(2, Part A), 611–620 Search PubMed.
- S. N. Economidou and D. Douroumis, 3D printing as a transformative tool for microneedle systems: Recent advances, manufacturing considerations and market potential, Adv. Drug Delivery Rev., 2021, 173, 60–69 CrossRef CAS PubMed.
-
H. Wijshoff, Drop formation mechanisms in piezo-acoustic inkjet, Proc Nanotech 2007, 2007, vol. 3, p. 448 Search PubMed.
- A. A. Castrejón-Pita, J. R. Castrejón-Pita and I. M. Hutchings, Breakup of Liquid Filaments, Phys. Rev. Lett., 2012, 108(7), 074506 CrossRef PubMed.
- J. R. Castrejón-Pita, A. A. Castrejón-Pita and S. S. Thete,
et al., Plethora of transitions during breakup of liquid filaments, Proc. Natl. Acad. Sci. U. S. A., 2015, 112(15), 4582–4587 CrossRef PubMed.
- R. F. Day, E. J. Hinch and J. R. Lister, Self-similar capillary pinchoff of an inviscid fluid, Phys. Rev. Lett., 1998, 80(4), 704 CrossRef CAS.
- F. P. Contò, J. F. Marín and A. Antkowiak,
et al., Shape of a recoiling liquid filament, Sci. Rep., 2019, 9(1), 15488 CrossRef PubMed.
- D. Broboana, A.-M. Bratu and I. Magos,
et al., Kinematics of the viscous filament during the droplet breakup in air, Sci. Rep., 2022, 12(1), 1774 CrossRef CAS PubMed.
- A. van der Bos, M.-J. van der Meulen and T. Driessen,
et al., Velocity profile inside piezoacoustic inkjet droplets in flight: comparison between experiment and numerical simulation, Phys. Rev. Appl., 2014, 1(1), 014004 CrossRef.
- S.-H. Kang, S. Kim and D. KeeSohn,
et al., Analysis of drop-on-demand piezo inkjet performance, Phys. Fluids, 2020, 32(2), 022007 CrossRef CAS.
- Y. Zhang, G. Hu and Y. Liu,
et al., Suppression and Utilization of Satellite Droplets for Inkjet Printing: A Review, Processes, 2022, 10(5), 932 CrossRef CAS.
- H. Dong, W. W. Carr and J. F. Morris, An experimental study of drop-on-demand drop formation, Phys. Fluids, 2006, 18(7), 072102 CrossRef.
- J. Dijksman, Hydrodynamics of small tubular pumps, J. Fluid Mech., 1984, 139, 173–191 CrossRef.
-
E. P. Furlani, B. G. Price and G. Hawkins, et al., Thermally induced Marangoni instability of liquid microjets with application to continuous inkjet printing, Proceedings NSTI Nanotechnology Conference, 2006 Search PubMed.
- P. M. Kamat, B. W. Wagoner and S. S. Thete,
et al., Role of Marangoni stress during breakup of surfactant-covered liquid threads: reduced rates of thinning and microthread cascades, Phys. Rev. Fluids, 2018, 3(4), 043602 CrossRef.
- P. M. Kamat, B. W. Wagoner and A. A. Castrejón-Pita,
et al., Surfactant-driven escape from endpinching during contraction of nearly inviscid filaments, J. Fluid Mech., 2020, 899, A28 CrossRef CAS.
- S. D. Hoath, W.-K. Hsiao and G. D. Martin,
et al., Oscillations of aqueous PEDOT: PSS fluid droplets and the properties of complex fluids in drop-on-demand inkjet printing, J. Non-Newtonian Fluid Mech., 2015, 223, 28–36 CrossRef CAS.
- H. J. Staat, A. van der Bos and M. van den Berg,
et al., Ultrafast imaging method to measure surface tension and viscosity of inkjet-printed droplets in flight, Exp. Fluids, 2017, 58, 1–8 CrossRef CAS.
- L. Yang, B. K. Kazmierski and S. D. Hoath,
et al., Determination of dynamic surface tension and viscosity of non-Newtonian fluids from drop oscillations, Phys. Fluids, 2014, 26(11), 113103 CrossRef.
- A. Fraters, R. Jeurissen and M. van den Berg,
et al., Secondary tail formation and breakup in piezoacoustic inkjet printing: Femtoliter droplets captured in flight, Phys. Rev. Appl., 2020, 13(2), 024075 CrossRef CAS.
- S. D. Hoath, O. G. Harlen and I. M. Hutchings, Jetting behavior of polymer solutions in drop-on-demand inkjet printing, J. Rheol., 2012, 56(5), 1109–1127 CrossRef CAS.
- P. Zhu, R. Chen and L. Wang, Topography-Directed Hot-Water Super-Repellent Surfaces. Advanced, Science, 2019, 6(18), 1900798 CAS.
- L. Ha and J. H. Park, 3-Dimensional Coating Polymer Microneedles for Economical and Efficient Transdermal, Drug Delivery, 2014, 38(3), 391–396 Search PubMed.
- Y. Zhu, W. Xu and Z. Cao,
et al., Prediction of Contact Angle for Oriented Hydrophobic Surface and Experimental Verification by Micro-Milling, Coatings, 2023, 13(8), 1305 CrossRef CAS.
- V. Marturano, V. Bizzarro and V. Ambrogi,
et al., Light-Responsive Nanocapsule-Coated Polymer Films for Antimicrobial Active Packaging, Polymers, 2019, 11, 68 CrossRef PubMed.
- H.-F. Y. Meng-Hsuan Hsiao, T.-J. Liu and J. Wang, Drug Loading on Microneedles [Research article], Adv. Chem. Eng. Sci., 2019, 9(2), 204–222 CrossRef.
- P. S. Brown and B. Bhushan, Durable, superoleophobic polymer–nanoparticle composite surfaces with re-entrant geometry via solvent-induced phase transformation, Sci. Rep., 2016, 6(1), 21048 CrossRef PubMed.
- N. H. Che Ismail and H. Md. Akil, Effects of organomodified muscovite on the properties of acrylonitrile–butadiene–styrene nanocomposites, J. Appl. Polym. Sci., 2018, 135(47), 46827 CrossRef.
- E. Thangaraju, N. T. Srinivasan and R. Kumar,
et al., Fabrication of electrospun Poly L-lactide and Curcumin loaded Poly L-lactide nanofibers for drug delivery, Fibers Polym., 2012, 13(7), 823–830 CrossRef CAS.
- S. Y. Lee, Y. Lee and P. Le Thi,
et al., Sulfobetaine methacrylate hydrogel-coated anti-fouling surfaces for implantable biomedical devices, Biomater. Res., 2018, 22(1), 3 CrossRef PubMed.
- P. Muanchan, T. Kurose and H. Ito, Replication of Mesoscale Pore One-dimensional Nanostructures: Surface-induced Phase Separation of Polystyrene/Poly(vinyl alcohol) (PS/PVA) Blends, Polymers, 2019, 11(6), 1039 CrossRef PubMed.
- C.-S. Park, E. Y. Jung and H. J. Jang,
et al., Synthesis and Properties of Plasma-Polymerized Methyl Methacrylate via the Atmospheric Pressure Plasma Polymerization Technique, Polymers, 2019, 11(3), 396 CrossRef PubMed.
- K. Tokuda, T. Ogino and M. Kotera,
et al., Simple method for lowering poly(methyl methacrylate) surface energy with fluorination, Polym. J., 2015, 47(1), 66–70 CrossRef CAS.
- W. Shang and H. Jiang, Preparation and properties of a novel fluorinated epoxy resin/DGEBA blend for application in electronic materials, High Perform. Polym., 2020, 32(7), 793–800 CrossRef CAS.
- H.-J. Butt, J. Liu and K. Koynov,
et al., Contact angle hysteresis, Curr. Opin. Colloid Interface Sci., 2022, 59, 101574 CrossRef CAS.
- B. S. Yilbas, A. Al-Sharafi and H. Ali,
et al., Dynamics of a water droplet on a hydrophobic inclined surface: influence of droplet size and surface inclination angle on droplet rolling, RSC Adv., 2017, 7(77), 48806–48818 RSC.
- A. Pockels, Über Randwinkel und Ausbreitung von Flüssigkeiten auf festen Körpern, Phys. Z., 1914, 15, 39–46 CAS.
- H. B. Eral, D. t Mannetje and J. M. Oh, Contact angle hysteresis: a review of fundamentals and applications, Colloid Polym. Sci., 2013, 291, 247–260 CrossRef CAS.
- K.-Y. Law, Contact Angle Hysteresis on Smooth/Flat and Rough Surfaces. Interpretation, Mechanism, and Origin, Acc. Mater. Res., 2022, 3(1), 1–7 CrossRef CAS.
- J. Wang, Y. Wu and Y. Cao,
et al., Influence of surface roughness on contact angle hysteresis and spreading work, Colloid Polym. Sci., 2020, 298(8), 1107–1112 CrossRef CAS.
- T. Kajiya, D. Sawai and K. Miyata,
et al., Simple method to measure rheological properties of soft surfaces by a micro-needle contact, Eur. Phys. J. E: Soft Matter Biol. Phys., 2022, 45(9), 76 CrossRef CAS PubMed.
- B. Cabanillas, A. Mallma-Medina and M. Petkova-Gueorguieva,
et al., Influence of the Surface Energy of Different Brands of Polymethyl Methacrylate on the Adherence of Candida albicans: An In Vitro Study, J. Int. Soc. Prev. Community Dent., 2021, 11(1), 6–12 CrossRef PubMed.
- A. Mazzotta, A. Gabbani and M. Carlotti,
et al., Invisible Thermoplasmonic Indium Tin Oxide Nanoparticle Ink for Anti-counterfeiting Applications, ACS Appl. Mater. Interfaces, 2022, 14(30), 35276–35286 CrossRef CAS PubMed.
- K. Parate, S. V. Rangnekar and D. Jing,
et al., Aerosol-Jet-Printed Graphene Immunosensor for Label-Free Cytokine Monitoring in Serum, ACS Appl. Mater. Interfaces, 2020, 12(7), 8592–8603 CrossRef CAS PubMed.
- M. Borghetti, M. Serpelloni and E. Sardini,
et al., Mechanical behavior of strain sensors based on PEDOT:PSS and silver nanoparticles inks deposited on polymer substrate by inkjet printing, Sens. Actuators, A, 2016, 243, 71–80 CrossRef CAS.
- J. Lee, J. Kim and J. Park,
et al., Characterization of in situ sintering of silver nanoparticles on commercial photo papers in inkjet printing, Flexible Printed Electron., 2018, 3(2), 025001 CrossRef.
- D. Lv, W. Chen and W. Shen,
et al., Enhanced flexible room temperature ammonia sensor based on PEDOT: PSS thin film with FeCl3 additives prepared by inkjet printing, Sens. Actuators, B, 2019, 298, 126890 CrossRef CAS.
- M. Shariq, S. Chattopadhyaya and R. Rudolf,
et al., Characterization of AuNPs based ink for inkjet printing of low cost paper based sensors, Mater. Lett., 2020, 264, 127332 CrossRef CAS.
- D. M. Stanković, M. Ognjanović and M. Jović,
et al., Disposable Biosensor Based on Amidase/CeO2/GNR Modified Inkjet-printed CNT Electrodes-droplet Based Paracetamol Detection in Biological Fluids for “Point-of-care” Applications, Electroanalysis, 2019, 31(8), 1517–1525 CrossRef.
- D. Bugakova, V. Slabov and E. Sergeeva,
et al., Comprehensive characterization of TiO2 inks and their application for inkjet printing of microstructures, Colloids Surf., A, 2020, 586, 124146 CrossRef CAS.
- A. Kowalski and Z. Czech, The effects of substrate surface properties on tack performance of acrylic Pressure-Sensitive Adhesives (PSAs), Int. J. Adhes. Adhes., 2015, 60, 9–15 CrossRef CAS.
- S. Wang, J. Xu and W. Wang,
et al., Skin electronics from scalable fabrication of an intrinsically stretchable transistor array, Nature, 2018, 555(7694), 83–88 CrossRef CAS PubMed.
- M. J. Kim, S. C. Park and S.-O. Choi, Dual-nozzle spray deposition process for improving the stability of proteins in polymer microneedles, RSC Adv., 2017, 7(87), 55350–55359, 10.1039/C7RA10928H.
- U. Angkawinitwong, A. J. Courtenay and A. M. Rodgers,
et al., A Novel Transdermal Protein Delivery Strategy via Electrohydrodynamic Coating of PLGA Microparticles onto Microneedles, ACS Appl. Mater. Interfaces, 2020, 12(11), 12478–12488 CrossRef CAS PubMed.
- C. O'Mahony, A. Bocchino and M. J. Haslinger,
et al., Piezoelectric inkjet coating of injection moulded, reservoir-tipped microneedle arrays for transdermal delivery, J. Micromech. Microeng., 2019, 29(8), 085004 CrossRef.
- S. Bakshi, P. Pandey and Y. Mohammed,
et al., Porous silicon embedded in a thermoresponsive hydrogel for intranasal delivery of lipophilic drugs to treat rhinosinusitis, J. Controlled Release, 2023, 363, 452–463 CrossRef CAS PubMed.
- X. Jin, S. E. Alavi and A. Shafiee,
et al., Metamorphosis of Topical Semisolid Products—Understanding the Role of Rheological Properties in Drug Permeation under the “in Use” Condition, Pharmaceutics, 2023, 15(6), 1707 CrossRef CAS PubMed.
- M. Dabbaghi, S. Namjoshi and B. Panchal,
et al., Viscoelastic and Deformation Characteristics of Structurally Different Commercial Topical Systems, Pharmaceutics, 2021, 13(9), 1351 CrossRef PubMed.
- E. E. Peters, M. Ameri and X. Wang,
et al., Erythropoietin-Coated ZP-Microneedle Transdermal System: Preclinical Formulation, Stability, and Delivery, Pharm. Res., 2012, 29(6), 1618–1626 CrossRef CAS PubMed.
- H. Kathuria, D. Lim and J. Cai,
et al., Microneedles with Tunable Dissolution Rate, ACS Biomater. Sci. Eng., 2020, 6(9), 5061–5068 CrossRef CAS PubMed.
- Q. Jing, H. Ruan and J. Li,
et al., Keratinocyte membrane-mediated nanodelivery system with dissolving microneedles for targeted therapy of skin diseases, Biomaterials, 2021, 278, 121142 CrossRef CAS PubMed.
- S. W. T. Chew, A. H. Shah and M. Zheng,
et al., A self-adhesive microneedle patch with drug loading capability through swelling effect, Bioeng. Transl. Med., 2020, 5(2), e10157 CrossRef CAS PubMed.
- Z. Zhu, J. Wang and X. Pei,
et al., Blue-ringed octopus-inspired microneedle patch for robust tissue surface adhesion and active injection drug delivery, Sci. Adv., 2023, 9(25), eadh2213 CrossRef CAS PubMed.
- N. Bjelobrk, H.-L. Girard and S. B. Subramanyam,
et al., Thermocapillary motion on lubricant-impregnated surfaces, Phys. Rev. Fluids, 2016, 1(6), 063902 CrossRef.
- J. B. Brzoska, F. Brochard-Wyart and F. Rondelez, Motions of droplets on hydrophobic model surfaces induced by thermal gradients, Langmuir, 1993, 9(8), 2220–2224 CrossRef CAS.
- E. Mele, D. Fragouli and R. Ruffilli,
et al., Complex architectures formed by alginate drops floating on liquid surfaces, Soft Matter, 2013, 9(27), 6338–6343 RSC.
- J. Hao, J. Lu and L. Lee,
et al., Droplet splashing on an inclined surface, Phys. Rev. Lett., 2019, 122(5), 054501 CrossRef CAS PubMed.
- J. Hao, J. Lu and Z. Zhang,
et al., Asymmetric droplet splashing, Phys. Rev. Fluids, 2020, 5(7), 073603 CrossRef.
- P. García-Geijo, G. Riboux and J. M. Gordillo, Inclined impact of drops, J. Fluid Mech., 2020, 897, A12 CrossRef.
- N. Blanken, M. S. Saleem and M.-J. Thoraval,
et al., Impact of compound drops: a perspective, Curr. Opin. Colloid Interface Sci., 2021, 51, 101389 CrossRef CAS.
- J. Hao and S. I. Green, Splash threshold of a droplet impacting a moving substrate, Phys. Fluids, 2017, 29(1), 012103 CrossRef.
- H. Almohammadi and A. Amirfazli, Understanding the drop impact on moving hydrophilic and hydrophobic surfaces, Soft Matter, 2017, 13(10), 2040–2053 RSC.
- S. Buksh, H. Almohammadi and M. Marengo,
et al., Spreading of low-viscous liquids on a stationary and a moving surface, Exp. Fluids, 2019, 60, 1–12 CrossRef.
- D. A. Burzynski and S. E. Bansmer, Role of surrounding gas in the outcome of droplet splashing, Phys. Rev. Fluids, 2019, 4(7), 073601 CrossRef.
- Z. Jian, C. Josserand and S. Popinet,
et al., Two mechanisms of droplet splashing on a solid substrate, J. Fluid Mech., 2018, 835, 1065–1086 CrossRef CAS.
- C. Josserand and S. T. Thoroddsen, Drop impact on a solid surface, Annu. Rev. Fluid Mech., 2016, 48, 365–391 CrossRef.
- E. Li and S. T. Thoroddsen, Time-resolved imaging of a compressible air disc under a drop impacting on a solid surface, J. Fluid Mech., 2015, 780, 636–648 CrossRef.
Footnote |
† Co-first authors: Masood Ali and Yanling Yang. |
|
This journal is © The Royal Society of Chemistry 2024 |
Click here to see how this site uses Cookies. View our privacy policy here.