DOI:
10.1039/D4MA00806E
(Paper)
Mater. Adv., 2024,
5, 8208-8222
A naphthalene–phenanthro[9,10-d]imidazole-based π-conjugated molecule with a self-assembly-induced tuneable multiple fluorescence output exhibits artificial light-harvesting properties†
Received
9th August 2024
, Accepted 14th September 2024
First published on 16th September 2024
Abstract
Among promising new materials, π-conjugated organic molecules are considered an attractive platform for the design and development of a wide range of self-assembled superstructures with desirable optical and electrical properties necessary for use in organic optoelectronics applications. The optical and electrical properties of π-conjugated organic molecules and their possible applications are usually determined by their primary molecular structure and their intermolecular interactions in the self-assembled state. However, satisfying the structural requirements for achieving tuneable optical properties is a difficult task, which makes the design and development of novel high-performance π-conjugated organic systems for nano-optoelectronics a considerable challenge. In this paper, we report on the design and synthesis of a naphthalene–phenanthro[9,10-d] imidazole-based π-conjugated Schiff base molecule (L1) that exhibits aggregation-induced tunable luminescence properties facilitated by solvent polarity. Upon varying the medium polarity of the self-assembly medium, L1 self-assembles into various superstructures with distinct morphologies and generates multiple tunable emission colours (blue–green–yellow–white). In a highly polar THF
:
water = 1
:
9 medium, it displays aggregation-induced white light emission. These single component-based white-light emitters attract broad attention due to their potential applications in lighting devices and display media. Computational studies incorporating full geometry optimization, time-dependent density functional theory (TDDFT) calculations and molecular dynamics (MD) simulations were utilized to elucidate the enhanced π–π interaction influenced by increasing solvent polarity and orbitals involved in electronic transitions associated with different self-assembled states. More importantly, we constructed a highly efficient artificial light-harvesting system in a THF
:
water = 1
:
1 medium based on self-assembled L1 and rhodamine B (RhB), where L1 acts as an energy donor and RhB acts as an acceptor, exhibiting a strong antenna effect at a substantial donor/acceptor ratio. Our findings provide a novel versatile approach for developing efficient artificial light-harvesting systems based on the supramolecular self-assembly of suitably designed π-conjugated organic molecules with tuneable multiple emission properties.
Introduction
The primary energy source for living organisms is photosynthesis, a process utilized by green plants, algae and certain bacteria to convert solar energy into chemical energy.1,2 In the first stage of photosynthesis, light is employed to generate high-energy molecules. The energy harvested in this stage is stored inside the cell in the form of adenosine triphosphate (ATP) and then converted into chemical energy.3 Inspired by this, researchers have mimicked this natural process and developed various artificial light-harvesting systems (LHSs).4 These systems exhibit light-harvesting properties mediated by Förster resonance energy transfer (FRET).5–8 There are two essential preconditions for this process to take place: First, the donor should be densely packed without any considerable self-quenching effect, and secondly, the donor/acceptor ratio should be sufficiently high. When these preconditions are met, excitation energy can be transferred with minimum energy loss. In the FRET process, energy is usually transferred between a donor and an acceptor through covalent bonds or non-covalent interactions. Among the LHSs being developed, those based on non-covalent interactions are particularly intriguing due to their ease of synthesis, tuneable optical properties, energy transfer efficiency and solution processability, which offer several possibilities for the utilization of solar light in different fields. With the aim of developing clean and sustainable energy technologies, several artificial LHSs have been developed.9–12 Among them, self-assembled supramolecular systems have received substantial attention not only due to their tuneable and functional molecular structure, but also because effective energy transfer between chlorophyll and protein in natural systems also depends on supramolecular self-assembly.4,13–16 For example, Yang et al. reported an extremely effective self-assembled organic nanocrystal-based light-harvesting system able to mimic chlorosomes.17 However, detailed investigations of these artificial LHSs provide insights into fundamental processes and help to explore exciting applications in photovoltaics, photocatalysis and photopolymerization.5,6,11,18–21 The appropriate choice of a supramolecular scaffold, which can be a dendrimer, polymer or metal–organic framework, which can avoid fluorescence quenching with a corresponding FRET pair, is essential for achieving a promising light-harvesting system.22–27 Prof. Stang and his co-workers have developed a highly efficient light-harvesting system based on self-assembled fluorescent Pt(II) metallacycles.6 Recently, small organic functional π-conjugated molecules have received considerable interest due to their ability to self-assemble,28,29 their tuneable optical behaviour30,31 and their favourable charge transfer properties. These characteristics improve the efficiency of solar cells and provide promising advancements in organic photovoltaic and light-emitting diode technologies.32–34
The unique and varied functionalities of self-assembled π-conjugated molecules are promising for applications in molecular switches, photo-controllable smart materials, and photo-patterning.35–37 In this context, Chai et al. have developed a series of π-conjugated multifunctional molecules. Upon exposure to UV irradiation, one of them exhibits aggregation-induced emission (AIE) in an aqueous medium, associated with a reversible change in emission colour, and forms an effective light-harvesting system with rhodamine B.38 Herein we report on the design and synthesis of the naphthalene–phenanthro[9,10-d]-imidazole-based π-conjugated organic Schiff base compound named L1. We synthesized this probe via a condensation reaction of naphthalene-1,5-diamine with a π-conjugated aldehyde ligand, 4-(1H-phenanthro[9,10-d]-imidazole-2-yl) benzaldehyde (A1). We then investigated the solvent polarity-induced optical properties and the aggregation behaviour of this newly synthesized optical probe. Our results show that L1 exhibits tuneable emission properties that are induced by aggregation facilitated by favourable solvent polarity, which helps to understand the self-assembly of L1 into different superstructures varying in morphology depending on the polarity of the medium. Furthermore, we have successfully employed the self-assembled superstructures generated from L1 in a THF
:
water = 1
:
1 mixture to develop a highly effectual LHSs utilizing the commercial fluorophore rhodamine B (RhB). In this system, aggregated forms of L1 serve as donors whereas rhodamine B functions as an acceptor. Overall, our results clearly reflect the potentiality of using self-assembled L1 in sensing, bioimaging and the development of optoelectronic devices.
Results and discussion
Synthesis
We synthesized the π-conjugated Schiff base molecule L1via a straightforward coupling reaction between naphthalene-1,5-diamine with a π-conjugated aldehyde ligand, 4-(1H-phenanthro[9,10-d]-imidazole-2-yl)-benzaldehyde (A1), in a methanol:acetonitrile mixture (1
:
1) at room temperature (RT), as illustrated in Scheme 1. Details of the synthesis procedure are provided in Scheme S1 and S2 in the Experimental section of the ESI.† We then characterized all the synthesized products using standard analytical techniques (Fig. S1–S3, ESI†).
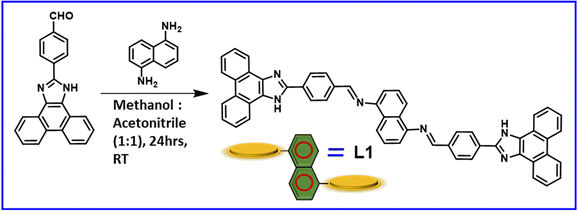 |
| Scheme 1 Schematic representation of the synthesis of L1. | |
Spectral analysis
We examined whether and how changes in solvent polarity affect the optical properties of L1. To this end, we first recorded the UV-Vis absorption spectra of L1 (20 μM) in a THF:water mixture with the percentage of water ranging from 0% to 90% (v/v). The absorption spectra of L1 (20 μM) in a pure THF medium exhibited an absorption peak at 378 nm (ε = 3.8 × 104 M−1 cm−1) and a broad band with an absorption maximum at 476 nm (ε = 2.3 × 103 M−1 cm−1). The higher energy absorption band at 382 nm is attributed to the intramolecular π–π* charge transfer (ICT) transition whereas the broad band absorption maximum at 476 nm is presumably due to the intramolecular charge transfer (CT) transition (Fig. 1A). Upon a gradual increase in the percentage of water (from 0% to 90%) in the THF:water mixture, we observed a noticeable bathochromic shift of the absorption maximum at 382 nm with reduced intensity. In a THF
:
water = 1
:
9 mixture, the absorption maximum shifted to 394 nm with an eventual distinctive red shift of 16 nm. This kind of bathochromic shift of the CT absorption band can be anticipated for J-type aggregation.30 Furthermore, for a better understanding of the polarity-induced aggregation process, we recorded the steady-state emission spectra of L1 in a THF:water mixture of varying polarity by altering the percentage of water from 0 to 90%. In pure THF, the steady-state photoluminescence spectra of L1 (20 μM) exhibited emission maxima at 533 nm that were observed upon excitation at 382 nm (Fig. 1B). Upon varying the water content in the THF:water mixture, we observed a significant change in the emission spectral pattern of L1. As we increased the polarity of the medium from 0 to 50% (THF
:
water = 1
:
1), the emission maxima underwent a significant bathochromic shift with enhanced emission intensity (Fig. 1B). A further increase in medium polarity (THF
:
water = 4
:
6 to 1
:
9) effectively decreased the emission intensity with red-shifted emission maxima and eventually caused a distinctive red shift of the emission maximum by ∼72 nm (Fig. 1B).
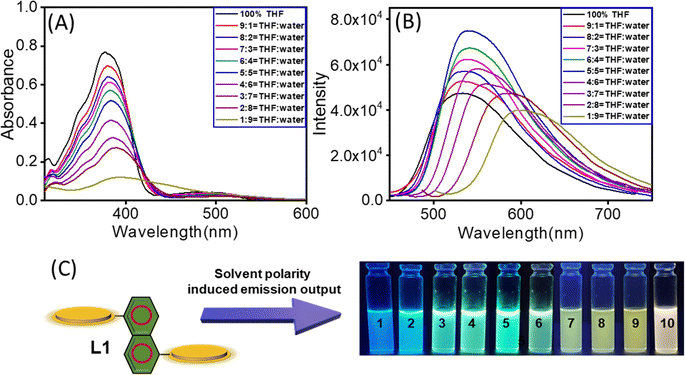 |
| Fig. 1 (A) UV-Vis and (B) emission spectra of L1 in THF and different THF:water mixtures. (C) Photographs showing the change in fluorescence colours of L1 upon irradiation with 365 nm light at different ratios of the THF:water mixtures. | |
It is obvious that an increase in medium polarity modifies the non-covalent intermolecular interactions responsible for molecular aggregation. This alters the pattern of molecular assembly, which determines the emissive properties corresponding to particular aggregated states.39,40 The emission spectral analysis of L1 exhibited a gradual red shift of the emission maximum with elevated emission intensity as the concentration of water in the THF:water mixture increased from 0 to 50%. This was mainly due to the aggregation-induced emission (AIE) mechanism.14,30,41 However, upon a further increase in water content in the THF:water mixture (60% to 90%), there was a considerable drop in emission intensity with a steady redshift of the emission maximum, indicating that the aggregation-caused quenching (ACQ) process took place.42,43 This noticeable steady red shift of the emission maximum with increasing medium polarity confirmed that the J-aggregation pattern of L1 changed with increasing medium polarity.39,40 The head-to-tail molecular arrangement due to J-type aggregation enhances the intermolecular electronic communications resulting in strong excitonic coupling and delocalization of the excited states across the molecules. This type of aggregation influences the electronic properties of the aggregates, specifically reduces the energy gap associated with the absorption and emission process with increasing intermolecular noncovalent interaction including π–π interactions and leads to a significant redshift of the corresponding absorption and emission maxima.44–47 This red shift of the ICT-based emission maximum with increasing medium polarity is due to the stabilization of the lowest unoccupied molecular orbital (LUMO) in the polar medium, which effectively reduces the energy gap associated with that CT transition.44 Additionally, we investigated the visually detectable luminescence colours generated by L1 in various THF:water mixtures with varying percentages of water. We observed that the emission colour changed remarkably from blue to green, then to yellow, and finally to white upon varying the water percentage from 0 to 90% in our THF:water mixture (Fig. 1C). The fluorescence spectra obtained for the THF
:
water = 1
:
9 mixture almost covered the entire visible region and exhibited white light emission. The above results indicate that the different aggregated states of this π-conjugated system based on L1 in different THF:water mixed solvent media varying in polarity account for the multiple luminescence colours observed.31L1 can adopt different molecular conformations consisting of several combinations of C–C or even C
N imine bond isomerizations with a wide range of torsional angles. In a non-polar and less polar solvent medium with a low water content (THF
:
water = 9
:
1), this type of molecular conformation is expected to prefer a non-radiative deactivation pathway leading to a minimum luminescence quantum yield for L1 (2.15%). In contrast, in a polar solvent with an increased percentage of water (THF
:
water = 1
:
1) the monomeric building blocks arrange themselves into a well-organized structure with increased luminescence quantum yield (2.50%) and a red-shifted emission maximum. More importantly, this bathochromic shift of the characteristic ICT-based emission maximum with increasing medium polarity, associated with both the AIE and the ACQ process, could originate from a single aggregated state (same ground state structure) or several aggregated states with different packing systems of the L1 molecular building block.48 A polar solvent with a greater percentage of water helps the L1 molecule to self-assemble with specific conformations through appropriately tuned operative non-covalent interactions, including π–π stacking and intermolecular H-bonding. Such a self-assembly process49 is expected to enforce certain restrictions on intermolecular torsional motion, which promotes a somewhat more planar conformation of the L1 molecule. This fact may be responsible for the lowering of the excited state energy and redshift of the characteristic ICT-based emission maxima of L1. Therefore, our UV-Vis and steady-state fluorescence spectral analysis of L1 in different solvent media of varying polarity revealed that in highly polar media with a higher percentage of water, conformations of L1 with enhanced planarity and conjugation were stabilized in their self-assembled state and accounted for the red-shifted white light emission.50,51
Morphological variation
The above results indicate that with increasing polarity of the solvent medium, L1 emits light of multiple colours associated with different self-assembled states of L1. We therefore expected the self-assembled L1-based structures obtained under varying medium polarity to differ in morphology. For this reason, we triggered the self-assembly of L1 in THF:water mixtures with a varied percentage of water. In a THF
:
water = 7
:
3 mixture, L1 self-assembled into thin fibrillar structures that formed a fibrillar network (Fig. 2A and B). In a THF
:
water = 4
:
6 mixture, L1 self-assembled into a fibrillar network consisting of thicker fibrils (Fig. 2C and D). In a highly polar THF
:
water = 1
:
9 mixture, L1 self-assembled into an aggregated network of needle-shaped nanorods (Fig. 2E and F). The emission spectra of L1 displayed a noticeable red shift of the characteristic emission maximum as the percentage of water in the THF:water mixture increased from 30% to 90% and produced luminescence of different colours ranging from green to yellow and to white (Fig. 2G–I). Fig. 2K shows the formation of different self-assembled superstructures in THF mixed solvents varying in water content. These morphologically varied L1-based self-assembled superstructures account for the aggregation-induced multi-colour luminescence described above. Morphological analysis helps to predict the mechanism by which the polarity of the medium induces the aggregation of L1. Initially, in a less polar medium (THF
:
water = 7
:
3 mixture) L1 self-assembles into thin fibrils. In a THF
:
water = 4
:
6 mixture, L1 self-assembles into a thicker fibrillar network due to enhanced hydrophobic interactions with increasing water content. When the percentage of water in the THF:water mixture reaches 90%, L1 self-assembles into needle-shaped nanorods with enhanced dimensions. In a highly polar self-assembly medium with a higher percentage of water, the molecular aggregation process tends to minimize hydrophobic interactions with water as well as the surface energy of self-assembled structures, giving rise to morphological variation in aggregated superstructures in solvents differing in water content (Fig. 2J and K). We carried out our quantitative morphological analysis of the self-assembled superstructures of L1 obtained in a THF:water mixture of varying polarity based on the distribution of width and length obtained from HR-SEM images (Fig. S4, ESI†). The L1 fibrils obtained with the THF
:
water = 7
:
3 mixture and THF
:
water = 4
:
6 mixture were homogeneously distributed, as shown in the SEM images captured at lower magnification. However, the population of fibres varied with the polarity of the self-assembly medium. The length of L1-derived fibrils obtained with 7
:
3 and 4
:
6 THF:water mixtures had an average length of 272 ± 10.1 μm and 255 ± 8.6 μm, respectively. The average length of the L1-based self-assembled needle-shaped nanorods obtained in a THF
:
water = 1
:
9 mixture was 174 ± 12.8 μm. In contrast, the width of the L1-based self-assembled fibrils and nanorods varied noticeably. The thinner fibrils obtained with a THF
:
water = 7
:
3 mixture self-assembly medium had an average diameter of ∼216 nm whereas the average diameter of the thicker fibril obtained in an THF
:
water = 4
:
6 mixture was ∼360 nm.
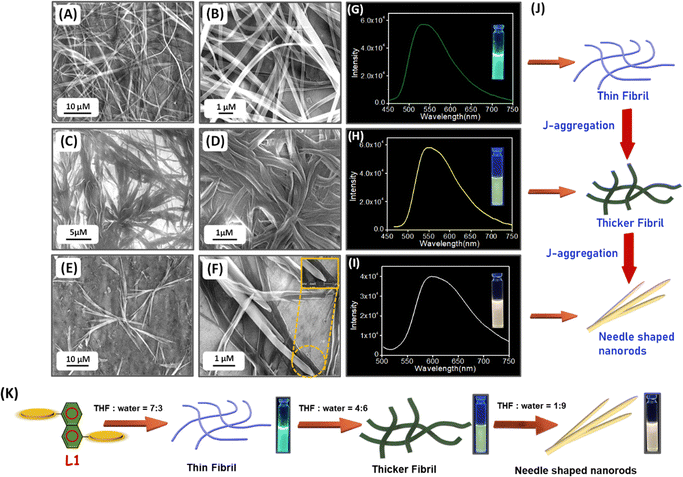 |
| Fig. 2 HR-SEM micrographs of the self-assembled structures formed by L1 in THF : water = 7 : 3 (A) and (B), THF : water = 4 : 6 (C) and (D) and THF : water = 1 : 9 mixtures (E) and (F). Emission spectra and luminescence colours of self-assembled L1 were obtained in THF : water = 7 : 3 (G), THF : water = 4 : 6 (H) and THF : water = 1 : 9 mixtures (I). (J) and (K) Pictorical representation of the morphologically different self-assembled structures formed by L1 building block in THF:water mixtures of varying polarity. | |
With L1-derived needle-shaped nanorods, the diameter varied in the range of ∼2.5 μm. We further performed dynamic light scattering (DLS) analysis of the self-assembled L1 with various morphologies obtained from different solvent systems of varying polarity (THF
:
water = 7
:
3, 4
:
6, and 1
:
9) and the results obtained from the DLS analysis is in good agreement with the morphological analysis through SEM (Fig. S5, ESI†).48 We subsequently aimed to investigate changes in intermolecular non-covalent forces associated with the different self-assembled states of L1 in different solvent media of varying polarity. In this context, we recorded Fourier transform infrared (FT-IR) spectra L1 self-assembled in THF
:
water = 7
:
3 and 1
:
9 medium respectively. The FT-IR spectra of L1 (solid mass) isolated from a THF
:
water = 7
:
3 mixture exhibited characteristic peaks at 1602 (νC=N(imine)), 1493 (νC=C(aromatic)), 1454 (νC–C(aromatic)), 1428 (νC–N(aromatic)), 2884 (νC–H(aromatic)) and 3213 cm−1 (νN–H(imidazole)). The FT-IR spectra of solid mass obtained with the THF
:
water = 1
:
9 mixture displayed noticeable changes in the position and intensity of peaks corresponding to νC=N(imine), νC=C(aromatic), νC–N(aromatic), νC–C(aromatic) and νC–H(aromatic) which unequivocally confirms the varied π–π stacking interactions during the self-assembly of L1 with changing polarity of the self-assembly medium.44 On the other hand, a substantial change in the peak position and peak intensity of the characteristic peak corresponding to νN–H(imidazole) signifies the further involvement of imidazole (N–H) through intermolecular hydrogen bonding in the molecular self-assembly process of L1 upon an increase in medium polarity (Fig. 3A and B).50,52 For further confirmation, we have also recoded the FT-IR spectra of monomeric L1 (unassembled state, Fig. S6, ESI†). The significant difference in the peak positions and peak intensity of the characteristic peaks compared with the self-assembled sate of L1 confirmed the involvement of π–π stacking interaction and intermolecular H-bonding in the self-assembled state.
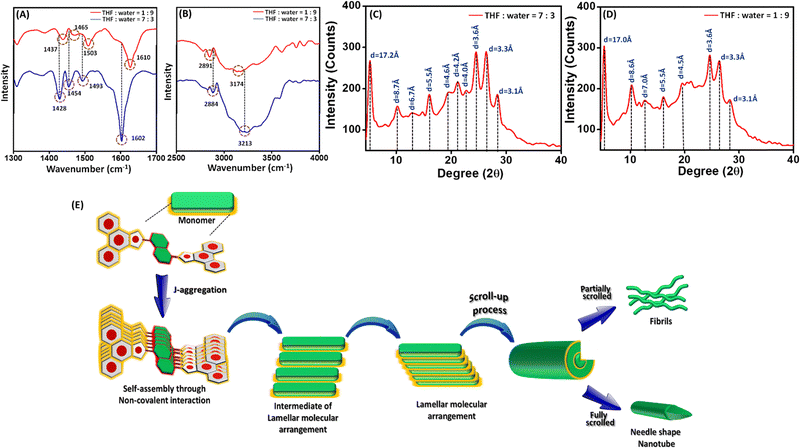 |
| Fig. 3 (A) and (B) FT-IR spectra of the dried mass of L1 obtained with a THF : water = 7 : 3 mixture (blue) and THF : water = 1 : 9 mixture (red). A PXRD pattern of the dried mass of L1 obtained with (C) THF : water = 7 : 3 mixture and (D) THF : water = 1 : 9 mixture. (E) Schematic illustration of the formation of fibril- and needle-shaped nanorods by self-assembly of L1 (via J-aggregation) through intermediate lamellar molecular arrangement followed by layer closure or a scroll-up process in THF:water mixtures with varied polarity. | |
The modes of molecular aggregation of L1 in various self-assembled states (obtained by varying the polarity of the self-assembly medium) were investigated further using powder X-ray diffraction (PXRD) analysis of the dried mass of L1 obtained with the THF
:
water = 7
:
3 and 1
:
9 mixtures, respectively (Fig. 3C and D). PXRD analysis of the dried mass of L1 obtained with the THF
:
water = 7
:
3 mixture revealed diffraction peaks at 19.2 and 24.4° (with d spacing values of 4.6 and 3.6 Å, respectively), which suggests the presence of a π–π stacking interaction between the monomeric building blocks of L1. Similar characteristic peaks at 19.4 and 24.5° (with d-spacing values of 4.5 and 3.6 Å) in the PXRD spectrum of dried mass of L1 obtained from a THF
:
water = 1
:
9 mixture reflect the existence of intermolecular π–π stacking interactions.53,54 Furthermore, in the wide-angle region, the diffraction peak appears at 28.5° (with a d-spacing value of 3.1 Å) and 28.3° (with a d-spacing value of 3.1 Å) for the dried mass of L1 obtained with the THF
:
water = 7
:
3 and 1
:
9 mixtures, respectively, anticipated in the presence of intermolecular hydrogen bonding involving the imidazole N–H.53,54 Furthermore, as can be seen from Fig. 3C, diffraction peaks appeared for the thin fibrillar structures self-assembled by L1 in a THF
:
water = 7
:
3 mixture at 5.3°, followed by other characteristic nearly periodically spaced peaks at 10.1, 15.9, 21.1 and 26.4° with corresponding d-spacing values of 17.2, 8.7, 5.5, 4.2 and 3.3 Å, respectively. This sequence of ratios approximately equalling 1/2, 1/3, 1/4 and 1/5 suggests that the formation of a lamellar molecular arrangement during the self-assembly of L1 in the THF
:
water = 7
:
3 mixture yields thin fibrillar superstructures.54 The PXRD spectrum of self-assembled L1 in a highly polar THF
:
water = 1
:
9 mixture displayed a similar characteristic peak at 5.1° followed by other nearly periodically spaced peaks at 10.2, 16.0, 19.4 and 24.5° with corresponding d-spacing values of 17.0, 8.6, 5.5, 4.5 and 3.6 Å, respectively (Fig. 3D). This periodic ratio is consistent with the lamellar molecular arrangement of L1 during self-assembly, indicating the formation of tubular superstructures through a layered array of L1. To assume an energetically favourable and stable molecular arrangement in a highly polar THF
:
water = 1
:
9 mixture, the layered lamellar arrangements of L1 underwent a scroll-up process and generated a superstructure with nanorod-like morphology (Fig. 3E). This scroll-up process not only protects the π-conjugated aromatic moiety from hydrophobic interactions, but also minimizes the surface energy of the resulting superstructures.53 It is well established that Schiff base compounds are usually unstable in an aqueous medium because of the propensity of the imine bond (C
N) towards hydrolysis in the presence of water. Therefore, there is a possibility that L1 in an aqueous medium can easily dissociate into its aldehyde (A1) and naphthalene diamine precursors (B1), or its mono-condensed intermediate. One detailed 1H NMR study described the formation of imine molecules from several aldehydes and amines in an aqueous medium within the pH range of 7–11.55 The study proposed a three-parameter linear equation correlating the logarithms of imine formation constants with pKa, highest occupied molecular orbital (HOMO) energies of amines, and lowest unoccupied molecular orbital (LUMO) energies of aldehydes, which provide a basis for the search for structural stability correlations conducive to imine formation in an aqueous medium and explain imine formation in such a medium. This result might shed light on different imine formation reactions not only in mixed aqueous media,56 but also in pure water.57 It has also been reported that imine-based porous organic cages exhibit comparably excellent hydrolytic stability.58
To verify the hydrolytic stability of L1, we recorded the UV-Vis absorption and steady-state emission spectra of the precursors imidazole-based aldehyde (A1) and naphthalene diamine (B1) in THF:water mixtures of varying polarity. Noticeable differences in the electronic spectral pattern of the starting aldehyde (A1) and diamine (B1) of L1 suggest that L1 is stable in aqueous media (Fig. S7, ESI†). Furthermore, the emission colour of the parental aldehyde (A1) and the diamine (B1) in a THF:water mixture with varied polarity (Fig. S8, ESI†) confirmed that the visually detectable changes in the emission colour of L1 upon altering the polarity of the medium are due to aggregation induced by changing the medium polarity and not due to hydrolytic decomposition.
DFT studies
To further investigate the proposed self-assembly/aggregation-induced single-molecule fluorescence properties, we performed theoretical geometry optimization and electronic transition calculations for L1 using density functional theory (DFT) and time-dependent DFT (TDDFT) calculations. The optimized geometry of the L1 monomer can exist in two different orientations: One, S1, is the open form and the other, S2, is the folded form resulting from intramolecular π–π interactions (Fig. 4a and b). Due to the presence of intramolecular π–π stacking interactions, S2 is more stable, and the energy gap between the S1 and S2 forms decreases with increasing solvent polarity. The dimeric structures, namely the S1 dimer and the S2 dimer, can consist of both monomeric forms S1 and S2, respectively. In our study, we considered two different types of stacking modes: first, in the S1 dimeric form of L1, there is tight face-to-face stacking where all aromatic ring planes overlap with the adjacent molecule. Secondly, in contrast, in the S2 dimeric form, π-conjugated phenanthroimidazole-2-yl moieties overlap intermolecularly with each other (Fig. 4c and d). Above we discuss the influence of operative non-covalent interactions and the morphological transformation resulting from changes in molecular assembly patterns associated with varying solvent medium polarity. The first conclusion is that the molecules under consideration are more likely to self-assemble in a highly polar solvent. Secondly, the transformation of the more stable S2 monomer into the S1 monomer is greater with increasing medium polarity. Based on these facts, we can predict the formation of the S2 dimeric form of L1 in a 100% THF medium whereas the S1 dimeric form of L1 is preferentially formed in a THF
:
water = 1
:
9 mixture. The optimized geometries show that the phenanthroimidazole-2-yl moieties in the S1 dimer overlap by approximately 3.528 Å whereas in the S2 dimer this distance increases to 3.648 Å.
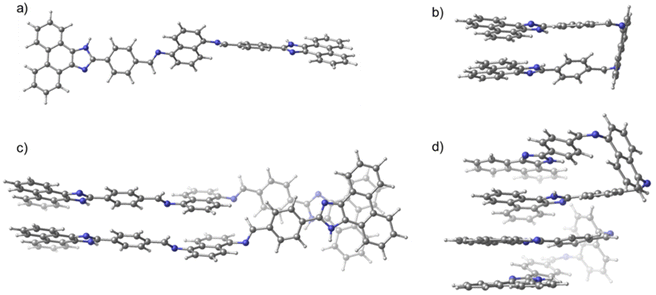 |
| Fig. 4 Optimized geometries of the calculated minima structure of the L1 monomer are shown in two orientations: (a) open (S1) and (b) stacked (S2). The corresponding dimer is also depicted in two orientations: (c) S1 dimer and (d) S2 dimer. [C: grey, N: blue, H: white]. | |
To explore the electronic transitions taking place in the π-stacked systems, we performed time-dependent DFT (TDDFT) calculations. The orbitals associated with specific electronic transitions in all compounds considered are elaborated in Fig. 5 and Fig. S9 (ESI†) and Table 1. In the THF medium, the S2 dimer exhibits two transitions at 328.19 nm and 326.46 nm (Table 1). These transitions primarily involve excitations between frontier molecular orbitals, with significant contributions associated with excitations passing from H−2, H−1 and H to L, L+1 and L+3. These transitions mainly involve excitations transferred from phenanthroimidazole-2-yl moieties to the core phenyl group. With a decreasing percentage of THF and increasing percentage of water, the self-assembly of L1 is enhanced, leading to the formation of an S1 dimeric form of L1, which exhibits strong π–π interactions, as observed previously. In a THF
:
water = 1
:
9 mixture, a bathochromic shift occurs with a transition at 356.66 nm. This red shift of 28–30 nm is in good agreement with our experimental observations. Aggregated dimers in the S1 state of L1, stacked in a tightly bound face-to-face arrangement, lower the energy gap between the HOMO and LUMO levels. The transition involves excitations transferred from phenanthroimidazole-2-yl moieties and the LUMO is located on the core phenyl group.
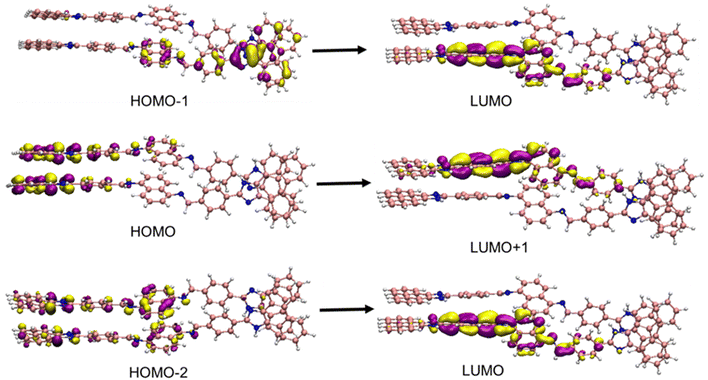 |
| Fig. 5 Frontier molecular orbitals of the calculated minima structure of the L1 dimer involved in the main absorption peak at wB97XD/def2-TZVPP in a THF : water = 1 : 9 mixture. [C: pink, N: blue, H: white]. The density of the molecular orbitals is shown in yellow and violet with an isosurface value of 0.03. | |
Table 1 The transition coefficients and associated eigenvalues of the dominated excitation in L1
|
Medium |
Excitation energy (eV) |
Wavelength (nm) |
Oscillator strength (f) |
Key transitions |
L1 (S1-dimeric form) |
THF : water = 1 : 9 |
3.476 |
356.66 |
4.859 |
HOMO−2 → LUMO |
HOMO−1 → LUMO |
HOMO → LUMO+1 |
L1 (S2-dimeric form) |
100% THF |
3.778 |
328.19 |
1.081 |
HOMO → LUMO+3 |
HOMO−2 → LUMO |
HOMO−2 → LUMO+1 |
3.798 |
326.46 |
1.002 |
HOMO → LUMO+1 |
HOMO−1 → LUMO |
HOMO−1 → LUMO+1 |
Ab initio molecular dynamics
The π-conjugated molecules self-assemble in polar solvents, and solvent polarity-induced morphological differences of their self-assembled state are responsible for their multi-colour luminescence, which is therefore tuneable. We evaluated the dynamic nature and stability of the π-stacked structure using MD simulations at 300 K over a simulation time of 10 ps. The MD simulation of the S1 dimer in water medium indicates the existence of strong π–π interactions throughout the simulation (Fig. 6). The distance between the centres of mass (COM) of the two S1 monomers does not fluctuate significantly after 4 ps and the monomers are bound together by strong inter-molecular π–π interactions. The π-stacked structure forms hydrogen bonds with explicit water molecules, as shown by the number of hydrogen bonds formed during the simulation time in Fig. S10 (ESI†). However, the S2 dimer does not form significant hydrogen bonds with explicit THF. The distance between the centres of mass (COM) of the two S2 monomers remains large throughout the simulation time, as illustrated in Fig. S10 (ESI†).
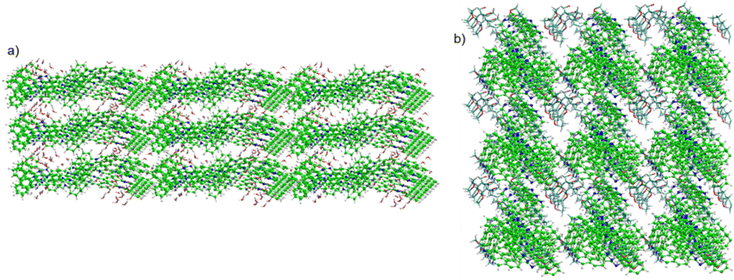 |
| Fig. 6 Snapshots taken at a simulation time of 5 ps for (a) the S1 dimeric form of L1 with water, and (b) the S2 dimeric form of L1 with THF. [C: green, C(THF): cyan, O: red, H: white]. | |
Light-harvesting properties
A mechanistic clarification of this morphological transformation of self-assembled L1 upon varying the polarity of the self-assembly medium is also expected to inspire questions regarding the potential applications of such self-assembled superstructures with characteristic emission properties. Previously it was described that π-conjugated cyano stilbene derivates are able to self-assemble into a wide range of nanostructures with tuneable emission and transport properties.59 Gosh et al. reported on a supramolecular molecular wire encapsulated into π-conjugated organogels which acts as an efficient light-harvesting antenna with colour-tuneable emission.60 Intrigued by this finding, we have set out to explore the possibility of using these L1-based self-assembled fluorescent superstructures as light-harvesting antenna systems. An efficient Förster resonance energy transfer (FRET) system relies on two critical factors for it to be operational. First, there must be a substantial overlap between the emission spectrum of the donor fluorophore and the absorption spectrum of the acceptor fluorophore. Secondly, the distance between the donor and acceptor fluorophores should ideally be within approximately 10 nm.59 This spatial closeness allows for efficient energy transfer between these two fluorophores. In this study, to set up an efficient FRET process, we chose self-assembled L1-based superstructures obtained from a THF
:
water = 1
:
1 mixture as a donor unit and the commercially available fluorescent dye rhodamine B (RhB) as an acceptor unit. RhB exhibits a sharp absorption peak at 560 nm and emits at 580 nm in a THF
:
water = 1
:
1 mixture (Fig. 7A). On the other hand, L1 exhibits a broad emission spectrum with an emission maximum at 539 nm (λExt = 382 nm) in a THF
:
water = 1
:
1 mixture (Fig. 7A and B). Noticeable spectral overlap between the emission spectrum of L1 and the absorption spectrum of RhB suggests that these compounds are an ideal FRET pair for an effective energy transfer process (Fig. 7B). In order to check this, we recorded the emission spectra of three different solutions: (i) self-assembled L1 (20 μM), (ii) self-assembled L1 (20 μM) in the presence of RhB (200 nM), and (iii) RhB only (200 nM) in a THF
:
water = 1
:
1 mixture (λExt = 382 nm; Fig. 7B). The emission spectrum recorded for the solution of self-assembled L1 in the presence of RhB in a THF
:
water = 1
:
1 mixture produced a strong emission band with an emission maximum at 598 nm (Fig. 7B, red line). In contrast, the spectrum recorded for the solution containing only 20 nM of RhB exhibited insignificant emission (Fig. 7B, pink line). These findings confirm that self-assembling L1 functions as a light-harvesting antenna and effectively transfers its excitation energy to an RhB acceptor unit. With the gradual addition of greater molar percentages (10–200 nM) of RhB, the emission intensity of the self-assembling L1 is progressively quenched (Fig. 7C). Interestingly, the emission intensity of the RhB increases steadily with a significant redshift of its emission maximum (Fig. 7C). The linear increase of the fluorescence response ratio (Fc/F0) with increasing concentration of RhB is presented in the inset of Fig. 7D. For further confirmation of this effective energy transfer process, we also recorded the emission spectra of solutions of RhB in varying concentrations from 10 to 200 nM (Fig. 8B). Self-assembled L1 (the donor) under an increasing concentration of RhB (the acceptor) effectively increases the characteristic emission intensity of RhB (8.2-fold) compared to when it is absent (1.9-fold; see the Fig. 7D). These results clearly demonstrate the efficiency of the energy transfer process between self-assembled L1 and RhB. The progressive quenching of the characteristic emission of self-assembled L1 with the gradual addition of RhB (10 to 200 nM) is due to the effective transfer of excitation energy from self-assembled L1 to RhB.
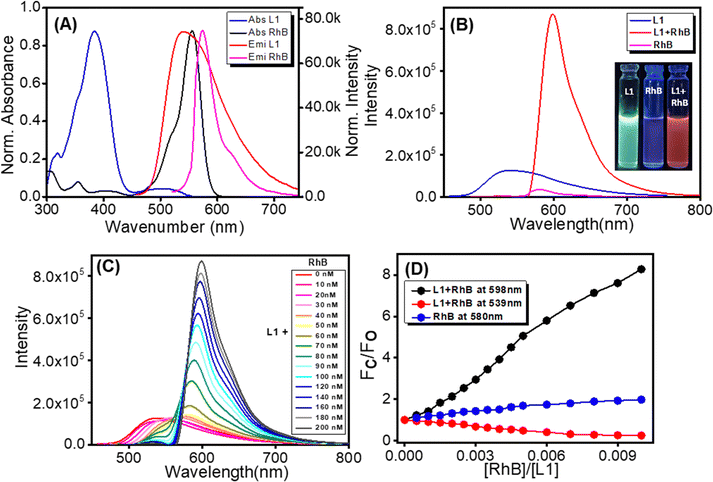 |
| Fig. 7 (A) Absorption spectra of L1 (blue) and RhB (black) and emission spectra of L1 (red) and RhB (pink) in the THF : water = 1 : 1 mixture. (B) Emission spectra of L1 (20 μM, blue), RhB (200 nM, pink) and L1 (20 μM) + RhB (200 nM) (red; λExt = 382 nm); inset: photograph of the emission colour of L1 (20 μM), RhB (200 nM) and L1 (20 μM) +RhB (200 nM). (C) Emission spectra of L1 (20 μM) in the THF : water = 1 : 1 mixture with varying concentrations of RhB. (D) Fluorescence intensity changes at 539 and 598 nm of L1 + RhB and 580 nm of RhB. | |
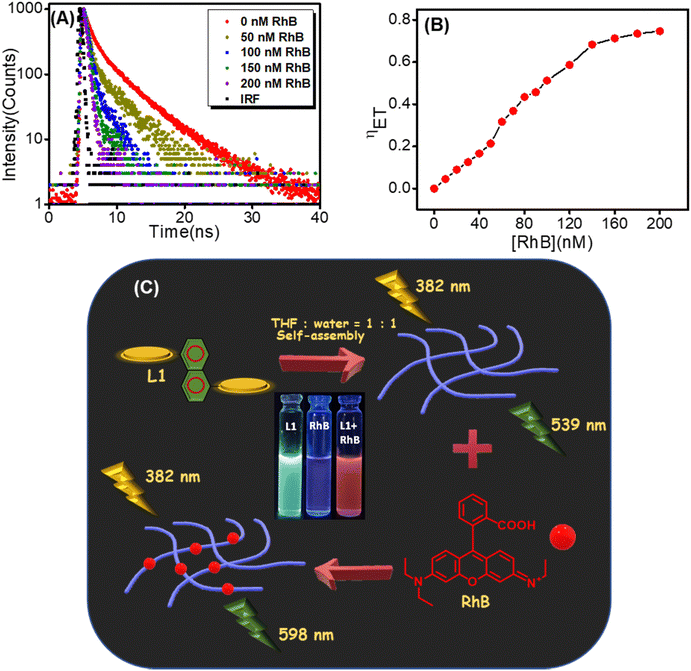 |
| Fig. 8 (A) Fluorescence lifetime decay profiles (λExt = 382 nm, monitored at 539 nm) of L1 upon the addition of different concentrations of RhB (0–200 nM). IRF – instrument response time. (B) Energy transfer efficiency as a function of [RhB]. (C) Schematic representation of the self-assembled L1/RhB-based light-harvesting system. | |
Furthermore, we performed time-resolved emission studies to confirm that the energy transfer process outlined above actually takes place. Emission decay profiles of the self-assembled L1 (λExt = 382 nm, λMon = 539 nm) and self-assembled L1 with different amounts of acceptor RhB are presented in Fig. 8A. As the concentration of the acceptor RhB increases from 0 to 200 nM, gradually faster emission decay of self-assembled L1 is observed along with a drop in calculated average lifetime (τav) from 4.8 ns to 1.25 ns. This result strongly suggests that effectual energy transfer from self-assembled L1 to RhB does take place. We have also investigated the energy transfer efficiency (ηET) and the antenna effect (AE) of self-assembled L1/RhB systems, as these are crucial parameters for assessing the light-harvesting ability of any donor–acceptor system. The efficiency of energy transfer is quantified based on relative changes in emission intensity of self-assembled L1 as the concentration of RhB increases from 0 to 200 nM (Fig. 8B) using eqn (1).7,61,62 In the presence of 200 nM of RhB, the energy transfer efficiency reached approximately 74%. The antenna effect (AE) is also quantified by the ratio of emission intensity at the emission maximum of the acceptor in the presence of the donor (λExt = 382 nm) (Fig. 7C) to the emission intensity at the emission maximum of the acceptor in the absence of the donor (λExt = 560 nm) (Fig. S11, ESI†), formalized by eqn (2).63 The calculated value of AE for this L1 (20 μM)/RhB (200 nM)-based donor–acceptor system is 51 (details are given in the ESI† and Table S1),9 which confirms that this self-assembled L1–RhB system is an effective light-harvesting system with a significant antenna effect. Overall, the light-harvesting antenna effect of the donor L1 and the RhB acceptor is schematically illustrated in Fig. 8C.
| AE = FA(382nm)/FA(560nm) | (2) |
Conclusion
We have designed and synthesized a functional small π-conjugated molecule that we call L1 and explored its optical and self-assembly properties affected by the polarity of the medium in which it self-assembles into higher-level structures. L1 self-assembles into different superstructures with morphological heterogeneity affected by the polarity of the self-assembly medium, displaying tuneable aggregation-induced fluorescence properties. Therefore, L1 can be employed for generating multiple fluorescence colours (blue–green–yellow–white) by varying the polarity of the self-assembly medium and, more importantly, can be considered a functional π-conjugated system-based single-component white-light emitter. These significant medium polarity-controlled aggregation-induced emission properties make L1 a promising candidate for organic electronic and energy transfer applications. We utilized computational analyses, including full geometry optimization, TDDFT calculations and MD simulations, to elucidate the enhanced π–π interaction influenced by solvent polarity and the orbitals involved in the electronic transitions associated with different self-assembled states. Furthermore, we explored the potential applicability of L1 as a light-harvesting system. Self-assembled superstructures obtained from L1 in a THF
:
water = 1
:
1 mixture function as light-harvesting antennae and transfer excitation energy to the commercially available dye rhodamine B with substantial efficiency and an antenna effect. This work not only describes the generation of different superstructures with morphological individualities from a single molecular backbone, but also emphasizes the tuneable aggregation-induced ability of L1 to emit multi-colour luminescence. It is because of this ability that L1 might find applications in OLED devices and the fabrication of organic field effect transistors (OFETs). Additionally, the practical applications of this artificially engineered light-harvesting system can be extended to various other technological domains, including photovoltaics, photocatalysis and photopolymerization. The results of our research therefore potentially significantly contribute to the advancement of materials science and hold substantial promise in the field of organic electronics.
Materials and methods
Materials
All the chemicals and solvents used in the research reported here are commercially available and were used as received without further purification. 9,10-Phenanthrenequinone, naphthalene 1,5-diamine and sodium hydrogen carbonate were purchased from AVRA. Ammonium acetate was purchased from Sisco Research Laboratories (SRL) Pvt. Ltd, India. Glacial acetic acid, acetonitrile and methanol were purchased from Finar Ltd. Terephthaldehyde was purchased from Sigma-Aldrich. Other regular laboratory chemicals were purchased from Sisco Research Laboratory and Loba Chemicals (Mumbai, MH, India).
Synthesis of L1
The detailed protocol utilized to synthesize L1 is available in the Experimental section of the ESI† (Schemes S1, S2 and Fig. S1–S3, ESI†).
Self-assembly of L1
A fresh stock solution of L1 was prepared by dissolving this compound in THF to a concentration of 50 mg mL−1. We blended these solutions in several different proportions and diluted them with, THF
:
water = 7
:
3, THF
:
water = 4
:
6 and THF
:
water = 1
:
9 mixtures to achieve the desired concentrations of this building block for self-assembly. The polarized solvent allowed the molecules to self-assemble.
High-resolution scanning electron microscopy (HR-SEM)
A 10 μL drop of a self-assembled solution of L1 in each of the different solvent media was placed on a glass coverslip and allowed to dry at RT. SEM analysis was performed using a high-resolution scanning electron microscope (HR-SEM, Thermo Scientific Apreo S) operating at 18 kV.
Dynamic light scattering (DLS) analysis
DLS analysis (Zetasizernano ZS, Malvern Instruments, Malvern, UK) was carried out to find the average size distribution of L1 in THF:water mixtures with varying the solvent polarity.
Fourier transform infrared spectroscopy (FT-IR)
Fourier transform infrared spectra were recorded using an IRTracer-100 FT-IR spectrometer (Shimadzu) with a deuterated lanthanum α-alanine-doped triglycine sulphate (DLaTGS) detector. FT-IR for the dried mass of self-assembled L1 was carried out in powder form. The measurements were taken at 4 cm−1 resolution with an average of 1000 scans. Minimal transmittance minimal values were determined using the software IR Tracer supplied with the spectrometer.
UV-vis spectroscopy
UV-vis absorption spectra of the synthesized L1 were recorded in different solvent media with varying polarity using a UV-vis spectrophotometer (Agilent Cary 50 UV-vis double-beam absorption spectrophotometer).
Fluorescence spectroscopy
Fluorescence measurements were performed at RT using a fluorescence spectrophotometer (Edinburgh Instruments, FLS 1000). Emission spectra of the synthesized L1 were recorded in different solvent media with varying polarity using the appropriate excitation wavelengths.
X-ray diffraction (XRD) analysis
The PXRD pattern of samples was recorded by using a PANalytical X’Pert Pro Powder X-ray diffractometer. Data collection was carried out at room temperature using Cu Kα radiation (1.5406 Å, 40 kV, 30 mA) as the X-ray source in 2θ continuous scan mode (Bragg–Brentano geometry) in the range of 2–50° at a scan rate of 1 scan per minute and a time per step of 0.5 s.
DFT calculations
Monomer geometries were fully optimized using the ωB97XD/def2-TZVPP level whereas those of dimers were optimized at the ωB97XD/def2-SVP level.64,65 In the solvent, the geometries were optimized with the continuous COSMO solvation model.66 The solvent was modelled with a dielectric constant of ε = 7.4 (tetrahydrofuran) and ε = 76.44 (10%tetrahydrofuran). All calculations were performed using the Gaussian 16 package.67
Time-dependent (TD)-DFT calculations of the complex geometries were performed at the ωB97XD/def2-TZVPP level. The hybrid meta-GGA functional ωB97XD has a 100% fraction of HF exchange at long-range in addition to about 22% at short-range and also contains empirical dispersion terms.64 Unlike PBE0, the long-range-corrected functional ωB97XD properly describes the ground and excited state properties of complex molecules.68 The importance of long-range corrected functionals for charged systems and for describing CT states is explained in previous studies.69,70 The ten lowest vertical excitation energies were calculated based upon time-dependent density functional theory (TDDFT), utilizing optimized geometries.
Periodic boundary condition calculations were performed using the Vienna Ab initio Simulation Package (VASP) using the plane-wave basis set.71,72 All calculations were done on the PBE level of theory using Grimme empirical dispersion.73Ab initio MD simulations were performed using the isothermal–isobaric (NPT) ensemble until equilibrium was achieved.74,75 The MD simulation was performed at 300 K using the Langevin thermostat and standard pressure, and the timestep was set to 1 fs.
Author contributions
Priya Rana: analysis and interpretation of data and writing and modification of the manuscript. Mallayasamy Siva: analysis and interpretation of data and writing and modification of the manuscript. Rabindranath Lo: conceptualisation, methodology, and design acquisition of data, analysis and interpretation of data, and writing and editing of the manuscript. Priyadip Das: conceptualisation, methodology, and design acquisition of data, analysis and interpretation of data, writing and editing of the manuscript, study supervision, funding acquisition, and project administration.
Data availability
Characterization data for the compound along with further supporting data referenced in the manuscript are available in the ESI.†.
Conflicts of interest
There is no conflict of interest to declare.
Acknowledgements
P. D. acknowledges the Council of Scientific and Industrial Research (CSIR) (File No. 01(3077)/21/EMR-II) of the Government of India for research funding and the support of the Interdisciplinary Institute of Indian System of Medicine (IIISM), Nano Research Centre (NRC) of the SRM Institute of Science & Technology for allowing us to use its NMR facility. R. L. thanks Prof. Pavel Hobza from the Institute of Organic Chemistry and Biochemistry of the Czech Academy of Sciences (IOCB Prague) for enabling access to services of the institute's infrastructure for computational chemistry.
References
- G. D. Scholes, G. R. Fleming, A. Olaya-Castro and R. van Grondelle, Lessons from nature about solar light harvesting, Nat. Chem., 2011, 3, 763–774 CrossRef CAS PubMed.
- R. Croce and H. van Amerongen, Natural strategies for photosynthetic light harvesting, Nat. Chem. Biol., 2014, 10, 492–501 CrossRef CAS PubMed.
- G. McDermott, S. M. Prince, A. A. Freer, A. M. Hawthornthwaite-Lawless, M. Z. Papiz, R. J. Cogdell and N. W. Isaacs, Crystal structure of an integral membrane light-harvesting complex from photosynthetic bacteria, Nature, 1995, 374, 517–521 CrossRef CAS.
- Q. Zou, K. Liu, M. Abbas and X. Yan, Peptide-Modulated Self-Assembly of Chromophores toward Biomimetic Light-Harvesting Nanoarchitectonics, Adv. Mater., 2016, 28, 1031–1043 CrossRef CAS PubMed.
- J. Lalevée, M. Tehfe, F. Dumur, D. Gigmes, B. Graff, F. Morlet-Savary and J. Fouassier, Light-Harvesting Organic Photoinitiators of Polymerization, Macromol. Rapid Commun., 2013, 34, 239–245 CrossRef PubMed.
- K. Acharyya, S. Bhattacharyya, H. Sepehrpour, S. Chakraborty, S. Lu, B. Shi, X. Li, P. S. Mukherjee and P. J. Stang, Self-Assembled Fluorescent Pt(II) Metallacycles as Artificial Light-Harvesting Systems, J. Am. Chem. Soc., 2019, 141, 14565–14569 CrossRef CAS PubMed.
- S. Kundu and A. Patra, Nanoscale Strategies for Light Harvesting, Chem. Rev., 2017, 117, 712–757 CrossRef CAS PubMed.
- T. Xiao, C. Bao, L. Zhang, K. Diao, D. Ren, C. Wei, Z. Y. Li and X. Q. Sun, An artificial light-harvesting system based on the ESIPT–AIE–FRET triple fluorescence mechanism, J. Mater. Chem. A, 2022, 10, 8528–8534 RSC.
- T. Xiao, D. Ren, L. Tang, Z. Wu, Q. Wang, Z. Y. Li and X. Q. Sun, A temperature-responsive artificial light-harvesting system in water with tunable white-light emission, J. Mater. Chem. A, 2023, 11, 18419–18425 RSC.
- T. Xiao, X. Li, L. Zhang, K. Diao, Z. Y. Li, X. Q. Sun and L. Wang, Artificial stepwise light harvesting system in water constructed by quadruple hydrogen bonding supramolecular polymeric nanoparticles, Chin. Chem. Lett., 2024, 35, 108618 CrossRef CAS.
- J. Li, Y. Chen, J. Yu, N. Cheng and Y. Liu, A Supramolecular Artificial Light-Harvesting System with an Ultrahigh Antenna Effect, Adv. Mater., 2017, 29, 1701905 CrossRef PubMed.
- Z. Zhang, Z. Zhao, Y. Hou, H. Wang, X. Li, G. He and M. Zhang, Aqueous Platinum(II)-Cage-Based Light-Harvesting System for Photocatalytic Cross-Coupling Hydrogen Evolution Reaction, Angew. Chem., Int. Ed., 2019, 58, 8862–8866 CrossRef CAS PubMed.
- N. Aizawa, C. J. Tsou, I. S. Park and T. Yasuda, Aggregation-induced delayed fluorescence from phenothiazine-containing donor-acceptor molecules for high-efficiency non-doped organic light-emitting diodes, Polym. J., 2017, 49, 197–202 CrossRef CAS.
- Y. Li, Y. Dong, L. Cheng, C. Qin, H. Nian, H. Zhang, Y. Yu and L. Cao, Aggregation-Induced Emission and Light-Harvesting Function of Tetraphenylethene-Based Tetracationic Dicyclophane, J. Am. Chem. Soc., 2019, 141, 8412–8415 CrossRef CAS PubMed.
- A. Arrigo, F. Puntoriero, G. La Ganga, S. Campagna, M. Burian, S. Bernstorff and H. Amenitsch, Aggregation-Induced Energy Transfer in a Decanuclear Os(II)/Ru(II) Polypyridine Light-Harvesting Antenna Dendrimer, Chem, 2017, 3, 494–508 CAS.
- S. Guo, Y. Song, Y. He, X. Hu and L. Wang, Highly Efficient Artificial Light-Harvesting Systems Constructed in Aqueous Solution Based on Supramolecular Self-Assembly, Angew. Chem., 2018, 130, 3217–3221 CrossRef.
- P. Chen, Y. Weng, L. Niu, Y. Chen, L. Wu, C. Tung and Q. Yang, Light-Harvesting Systems Based on Organic Nanocrystals To Mimic Chlorosomes, Angew. Chem., Int. Ed., 2016, 55, 2759–2763 CrossRef CAS PubMed.
- B. Sun, K. Tao, Y. Jia, X. Yan, Q. Zou, E. Gazit and J. Li, Photoactive properties of supramolecular assembled short peptides, Chem. Soc. Rev., 2019, 48, 4387–4400 RSC.
- J. Lalevée, F. Dumur, C. R. Mayer, D. Gigmes, G. Nasr, M.-A. Tehfe, S. Telitel, F. Morlet-Savary, B. Graff and J. P. Fouassier, Photopolymerization of N-Vinylcarbazole Using Visible-Light Harvesting Iridium Complexes as Photoinitiators, Macromolecules, 2012, 45, 4134–4141 CrossRef.
- Z. Zhang, Z. Zhao, Y. Hou, H. Wang, X. Li, G. He and M. Zhang, Aqueous Platinum(II)-Cage-Based Light-Harvesting System for Photocatalytic Cross-Coupling Hydrogen Evolution Reaction, Angew. Chem., 2019, 131, 8954–8958 CrossRef.
- J. Yuan, Y. Zhang, J. Yuan, Y. Zhang, L. Zhou, G. Zhang, H. Yip, T. Lau and X. Lu, Single-Junction Organic Solar Cell with over 15% Efficiency Using Fused-Ring Acceptor with Electron-Deficient Core Single-Junction Organic Solar Cell with over 15% Efficiency Using Fused-Ring Acceptor with Electron-Deficient Core, Joule, 2017, 3, 1140–1151 CrossRef.
- Q. Hongwei, T. Xiao, R. B. P. Elmes and L. Wang, Construction of a sequential light-harvesting system via supramolecular copolymerization, Chin. Chem. Lett., 2023, 34, 108185 CrossRef.
- R. T. Gao, S. Y. Li, B. H. Liu, Z. Chen, N. Liu, L. Zhou and Z. Q. Wu, One-pot asymmetric living copolymerization-induced chiral self-assemblies and circularly polarized luminescence, Chem. Sci., 2024, 15, 2946–2953 RSC.
- N. Liu, R. T. Gao and Z. Q. Wu, Helix-Induced Asymmetric Self-Assembly of π-Conjugated Block Copolymers: From Controlled Syntheses to Distinct Properties, Acc. Chem. Res., 2023, 56(21), 2954–2967 CrossRef CAS PubMed.
- C. Wang, L. Xu, L. Zhuo, N. Liu and Z. Q. Wu, Asymmetric Living Supramolecular Polymerization: Precise Fabrication of One-Handed Helical Supramolecular Polymers, Angew. Chem., Int. Ed., 2022, 61, e202207028 CrossRef CAS PubMed.
- Y. X. Li, L. Xu, S. M. Kang, L. Zhou, N. Liu and Z. Q. Wu, Helicity- and Molecular-Weight-Driven Self-Sorting and Assembly of Helical Polymers towards Two-Dimensional Smectic Architectures and Selectively Adhesive Gels, Angew. Chem., Int. Ed., 2021, 60, 7174–7179 CrossRef CAS PubMed.
- L. Xu, C. Wang, Y. X. Li, X. H. Xu, L. Zhou, N. Liu and Z. Q. Wu, Crystallization-Driven Asymmetric Helical Assembly of Conjugated Block Copolymers and the Aggregation Induced White-light Emission and Circularly Polarized Luminescence, Angew. Chem., Int. Ed., 2020, 59, 16675–16682 CrossRef CAS PubMed.
- S. Ghosh, B. Jana, A. Ghosh, D. M. Guldi and A. Patra, The Impact of Aggregation of Quaterthiophenes on the Excited State Dynamics, J. Phys. Chem. Lett., 2021, 12, 3424–3430 CrossRef CAS PubMed.
- X. Yan, P. Zhu and J. Li, Self-assembly and application of diphenylalanine-based nanostructures, Chem. Soc. Rev., 2010, 39, 1877–1890 RSC.
- A. Maity, F. Ali, H. Agarwalla, B. Anothumakkool and A. Das, Tuning of multiple luminescence outputs and white-light emission from a single gelator molecule through an ESIPT coupled AIEE process, Chem. Commun., 2015, 51, 2130–2133 RSC.
- T. Singha Mahapatra, H. Singh, A. Maity, A. Dey, S. K. Pramanik, E. Suresh and A. Das, White-light-emitting lanthanide and lanthanide-iridium doped supramolecular gels: modular luminescence and stimuli-responsive behaviour, J. Mater. Chem. C, 2018, 6, 9756–9766 RSC.
- D. Braun, Semiconducting polymer LEDs, Mater. Today, 2002, 5, 32–39 CrossRef CAS.
- M. Más-Montoya and R. A. J. Janssen, The Effect of H- and J-Aggregation on the Photophysical and Photovoltaic Properties of Small Thiophene-Pyridine-DPP Molecules for Bulk-Heterojunction Solar Cells, Adv. Funct. Mater., 2017, 27, 1605779 CrossRef.
- Y.-J. Lee, J. Wang and J. W. P. Hsu, Surface photovoltage characterization of organic photovoltaic devices, Appl. Phys. Lett., 2013, 103, 173302 CrossRef.
- X. Gu, E. Zhao, T. Zhao, M. Kang, C. Gui, J. W. Y. Lam, S. Du, M. M. T. Loy and B. Z. Tang, A Mitochondrion-Specific Photoactivatable Fluorescence Turn-On AIE-Based Bioprobe for Localization Super-Resolution Microscope, Adv. Mater., 2016, 28, 5064–5071 CrossRef CAS PubMed.
- X. Gu, E. Zhao, J. W. Y. Lam, Q. Peng, Y. Xie, Y. Zhang, K. S. Wong, H. H. Y. Sung, I. D. Williams and B. Z. Tang, Mitochondrion-Specific Live-Cell Bioprobe Operated in a Fluorescence Turn-On Manner and a Well-Designed Photoactivatable Mechanism, Adv. Mater., 2015, 27, 7093–7100 CrossRef CAS PubMed.
- R. Xing, C. Yuan, W. Fan, X. Ren and X. Yan, Biomolecular glass with amino acid and peptide nanoarchitectonics, Sci. Adv., 2023, 9, eadd8105 CrossRef CAS PubMed.
- J. Chai, Y. Wu, B. Yang and B. Liu, The photochromism, light harvesting and self-assembly activity of a multi-function Schiff-base compound based on the AIE effect, J. Mater. Chem. C, 2018, 6, 4057–4064 RSC.
- P. Rana, G. Marappan, S. Sivagnanam, V. J. Surya, Y. Sivalingam and P. Das, Self-assembly induced tunable multiple fluorescence output from a white light-emitting functionalized single π-conjugated molecule and implication in VOC sensing applications, Mater. Chem. Front., 2022, 6, 1421–1436 RSC.
- P. Rana, A. Jennifer G, S. Rao T, S. Mukhopadhyay, E. Varathan and P. Das, Polarity-Induced Morphological Transformation with Tunable Optical Output of Terpyridine–Phenanthro[9,10- d]imidazole-Based Ligand and Its Zn(II) Complexes with I – V Characteristics, ACS Omega, 2023, 8, 48855–48872 CrossRef CAS PubMed.
- Y. Okazawa, K. Kondo, M. Akita and M. Yoshizawa, Polyaromatic Nanocapsules Displaying Aggregation-Induced Enhanced Emissions in Water, J. Am. Chem. Soc., 2015, 137, 98–101 CrossRef CAS PubMed.
- W. Z. Yuan, P. Lu, S. Chen, J. W. Y. Lam, Z. Wang, Y. Liu, H. S. Kwok, Y. Ma and B. Z. Tang, Changing the Behavior of Chromophores from Aggregation-Caused Quenching to Aggregation-Induced Emission: Development of Highly Efficient Light Emitters in the Solid State, Adv. Mater., 2010, 22, 2159–2163 CrossRef CAS PubMed.
- M. Belletête, J. Bouchard, M. Leclerc and G. Durocher, Photophysics and solvent-induced aggregation of 2,7-carbazole-based conjugated polymers, Macromolecules, 2005, 38, 880–887 CrossRef.
- J. Luo, Z. Xie, Z. Xie, J. W. Y. Lam, L. Cheng, H. Chen, C. Qiu, H. S. Kwok, X. Zhan, Y. Liu, D. Zhu and B. Z. Tang, Aggregation-induced emission of 1-methyl-1,2,3,4,5-pentaphenylsilole, Chem. Commun., 2001, 1740–1741 RSC.
- H. Piwonski, S. Nozue, H. Fujita, T. Michinobu and S. Habuchi, Organic J-Aggregate Nanodots with Enhanced Light Absorption and Near-Unity Fluorescence Quantum Yield, Nano Lett., 2021, 21(7), 2840–2847 CrossRef CAS PubMed.
- N. J. Hestand and F. C. Spano, Expanded Theory of H- and J-Molecular Aggregates: The Effects of Vibronic Coupling and Intermolecular Charge Transfer, Chem. Rev., 2018, 118(15), 7069–7163 CrossRef CAS PubMed.
- S. B. Anantharaman, J. Kohlbrecher, G. Raino, S. Yakunin, T. Stoferle, J. Patel, M. Kovalenko, R. F. Mahrt, F. A. Nuesch and J. Heier, Enhanced Room-Temperature Photoluminescence Quantum Yield in Morphology Controlled J-Aggregates, Adv. Sci., 2021, 8(4), 1903080 CrossRef CAS PubMed.
- S. Banerjee, A. K. Both and M. Sarkar, Probing the Aggregation and Signaling Behavior of Some Twisted 9,9′-Bianthryl Derivatives: Observation of Aggregation-Induced Blue-Shifted Emission, ACS Omega, 2018, 3(11), 15709–15724 CrossRef CAS PubMed.
- H. Zhang, K. Liu, S. Li, X. Xin, S. Yuan, G. Ma and X. Yan, Self-Assembled Minimalist Multifunctional Theranostic Nanoplatform for Magnetic Resonance Imaging-Guided Tumor Photodynamic Therapy, ACS Nano, 2018, 12, 8266–8276 CrossRef CAS PubMed.
- D. C. Lee, K. Jang, K. K. McGrath, R. Uy, K. A. Robins and D. W. Hatchett, Self-assembling asymmetric bisphenazines with tunable electronic properties, Chem. Mater., 2008, 20, 3688–3695 CrossRef CAS.
- L. Zhao, X. Ren and X. Yan, Assembly Induced Super-Large Red-Shifted Absorption: The Burgeoning Field of Organic Near-Infrared Materials, CCS Chem., 2021, 3, 678–693 CrossRef.
- C. Zhao, X. Qiao, Z. Yi, Q. Guan and W. Li, Active centre and reactivity descriptor of a green single component imidazole catalyst for acetylene hydrochlorination, Phys. Chem. Chem. Phys., 2020, 22, 2849–2857 RSC.
- A. Maity, A. Dey, M. Gangopadhyay and A. Das, Water induced morphological transformation of a poly(aryl ether) dendron amphiphile: Helical fibers to nanorods, as light-harvesting antenna systems, Nanoscale, 2018, 10, 1464–1473 RSC.
- A. Arul, P. Rana, K. Das, I. Pan, D. Mandal, A. Stewart, B. Maity, S. Ghosh and P. Das, Fabrication of self-assembled nanostructures for intracellular drug delivery from diphenylalanine analogues with rigid or flexible chemical linkers, Nanoscale Adv., 2021, 3, 6176–6190 RSC.
- C. Godoy-Alcántar, A. K. Yatsimirsky and J. M. Lehn, Structure-stability correlations for imine formation in aqueous solution, J. Phys. Org. Chem., 2005, 18, 979–985 CrossRef.
- V. Goral, M. I. Nelen, A. V. Eliseev and J. M. Lehn, Double-level “orthogonal” dynamic combinatorial libraries on transition metal template, Proc. Natl. Acad. Sci. U. S. A., 2001, 98, 1347–1352 CrossRef CAS PubMed.
- O. Ramström, S. Lohmann, T. Bunyapaiboonsri and J. Lehn, Dynamic Combinatorial Carbohydrate Libraries: Probing the Binding Site of the Concanavalin A Lectin, Chem. – A Eur. J., 2004, 10, 1711–1715 CrossRef PubMed.
- T. Hasell, M. Schmidtmann, C. A. Stone, M. W. Smith and A. I. Cooper, Reversible water uptake by a stable imine-based porous organic cage, Chem. Commun., 2012, 48, 4689 RSC.
- B. K. An, J. Gierschner and S. Y. Park, π-Conjugated Cyanostilbene Derivatives: A Unique Self-Assembly Motif for Molecular Nanostructures with Enhanced Emission and Transport, Acc. Chem. Res., 2012, 45, 544–554 CrossRef CAS PubMed.
- A. Ajayaghosh, V. K. Praveen, C. Vijayakumar and S. J. George, Molecular Wire Encapsulated into π Organogels: Efficient Supramolecular Light-Harvesting Antennae with Color-Tunable Emission, Angew. Chem., 2007, 119, 6376–6381 CrossRef.
- G. Sun, M. Zuo, W. Qian, J. Jiao, X. Y. Hu and L. Wang, Highly efficient artificial light-harvesting systems constructed in aqueous solution for supramolecular photocatalysis, Green Synth. Catal., 2021, 1, 32–37 CrossRef.
- T. Tsukamoto, T. Shimada and S. Takagi, Artificial Photosynthesis Model: Photochemical Reaction
System with Efficient Light-Harvesting Function on Inorganic Nanosheets, ACS Omega, 2018, 13, 18563–18571 CrossRef.
- L. Olejko and I. Bald, FRET efficiency and antenna effect in multi-color DNA origami-based light harvesting systems, RSC Adv., 2017, 7, 23924–23934 RSC.
- J. Chai, M. Head-gordon, J. Chai and M. Head-gordon, Systematic optimization of long-range corrected hybrid density functionals, J. Chem. Phys., 2008, 8, 084106 CrossRef PubMed.
- M. Alipour and P. Fallahzadeh, First principles optimally tuned range-separated phosphorus – hydrogen spin – spin coupling, Phys. Chem. Chem. Phys., 2016, 18, 18431–18440 RSC.
- A. Klamt and G. Schuurmann, COSMO: A New Approach to Dielectric Screening in Solvents with Explicit Expressions for the Screening Energy and its Gradient, J. Chem. Soc., Perkin Trans. 2, 1993, 799–805 RSC.
-
M. J. Frisch, G. W. Trucks, H. B. Schlegel, G. E. Scuseria, M. A. Robb, J. R. Cheeseman, G. Scalmani, V. Barone, G. A. Petersson, H. Nakatsuji, X. Li, M. Caricato, A. V. Marenich, J. Bloino, B. G. Janesko, R. Gomperts, B. Mennucci, H. P. Hratchian, J. V. Ortiz, A. F. Izmaylov, J. L. Sonnenberg, D. Williams-Young, F. Ding, F. Lipparini, F. Egidi, J. Goings, B. Peng, A. Petrone, T. Henderson, D. Ranasinghe, V. G. Zakrzewski, J. Gao, N. Rega, G. Zheng, W. Liang, M. Hada, M. Ehara, K. Toyota, R. Fukuda, J. Hasegawa, M. Ishida, T. Nakajima, Y. Honda, O. Kitao, H. Nakai, T. Vreven, K. Throssell, J. A. Montgomery, Jr., J. E. Peralta, F. Ogliaro,, M. J. Bearpark, J. J. Heyd, E. N. Brothers, K. N. Kudin, V. N. Staroverov, T. A. Keith, R. Kobayashi, J. Normand, K. Raghavachari, A. P. Rendell, J. C. Burant, S. S. Iyengar, J. Tomasi, M. Cossi, J. M. Millam, M. Klene, C. Adamo, R. Cammi, J. W. Ochterski, R. L. Martin, K. Morokuma, O. Farkas, J. B. Foresman, and D. J. Fox, Gaussian 16, Revision C.02, Gaussian, Inc., Wallingford CT, 2019 Search PubMed.
- Z. Chen, Y. Li, Z. He, Y. Xu and W. Yu, Theoretical investigations on charge transport properties of tetrabenzo [a, d, j, m] coronene derivatives using different density functional and wB97XD), J. Chem. Res., 2019, 43, 293–303 CrossRef CAS.
- L. Zhu, C. Yang, Y. Yi and Z. Wei, Effective Modulation of Exciton Binding Energies in Polymorphs of a Small-Molecule Acceptor for Organic Photovoltaics, J. Phys. Chem. Lett., 2020, 11, 10227–10232 CrossRef CAS PubMed.
- D. A. Links, Lowest excited states and optical absorption spectra of donor–acceptor copolymers for organic photovoltaics: a new picture emerging from tuned long-range corrected density functionals, Phys. Chem. Chem. Phys., 2012, 14, 14243–14248 RSC.
- G. Kresse and J. Furthmiiller, Efficiency of ab-initio total energy calculations for metals and semiconductors using a plane-wave basis set, Comput. Mater. Sci., 1996, 6, 15–50 CrossRef CAS.
- G. Kresse and D. Joubert, From ultrasoft pseudopotentials to the projector augmented-wave method, Phys. Rev. B: Condens. Matter Mater. Phys., 1999, 59, 11–19 Search PubMed.
- S. Grimme, S. Ehrlich and L. Goerigk, Effect of the Damping Function in Dispersion Corrected Density Functional Theory, J. Comput. Chem., 2011, 38, 1456–1465 CrossRef PubMed.
- A. R. M. Parrinello, Crystal structure and pair potential: A Molecular-Dynamics study, Phys. Rev. Lett., 1980, 45, 1196 CrossRef.
- M. Parrinello and A. Rahman, Polymorphic transitions in single crystals: A new molecular dynamics method, J. Appl. Phys., 1981, 52, 7182–7190 CrossRef CAS.
Footnotes |
† Electronic supplementary information (ESI) available: UV spectra, emission spectra, length and width distribution graph, fluorescence colour photographs, minima structure of dimers, and bond length variations in monomers. See DOI: https://doi.org/10.1039/d4ma00806e |
‡ Equal contribution |
|
This journal is © The Royal Society of Chemistry 2024 |
Click here to see how this site uses Cookies. View our privacy policy here.