DOI:
10.1039/D3NA00923H
(Paper)
Nanoscale Adv., 2024,
6, 287-301
Retracted Article: Sequestration of chromium(VI) and nickel(II) heavy metals from unhygienic water via sustainable and innovative magnetic nanotechnology†
Received
25th October 2023
, Accepted 23rd November 2023
First published on 24th November 2023
Abstract
In a stride towards sustainable solutions, this research endeavors to address the critical issue of water pollution via heavy metals by coupling the power of magnetic nanotechnology, in combination with a green chemistry approach, to eliminate two noxious inorganic pollutants: chromium(VI) and nickel(II) from aqueous environments. The synthesis of magnetite (Fe3O4) nanoparticles was achieved using ferric chloride hexahydrate (FeCl3·6H2O) as a precursor, with the assistance of Ziziphus mauritiana Lam. leaves extract, known for its remarkable salt-reducing properties. A range of bio-adsorbents, derived from corncob biomass, corncob pyrolyzed biochar, and magnetite/corncob biochar nanocomposite (NC), were engineered for their eco-friendly and biocompatible characteristics. Extensive parametric optimizations, including variations in pH, contact time, dose rate, and concentration, were carried out to gain insights into the adsorption behavior and capacity of these bioadsorbents concerning Cr(VI) and Ni(II). Equilibrium and kinetic studies were undertaken to comprehensively understand the adsorption dynamics. In the case of Ni(II), the Freundlich isotherm model provided a satisfactory fit for all bio-adsorbents, demonstrating R2 values of 0.91, 0.95, and 0.96 for BM, BC, and NC, respectively. Furthermore, the pseudo 1st order model emerged as the most suitable fit for Cr(VI) sequestration in corncob BM with an R2 value of 0.98, while pseudo 2nd order models were robustly fitted for BC and NC, yielding R2 values of 0.88 and 0.99, respectively. The magnetite/corncob nanocomposite outperformed other bioadsorbents in removing heavy metals from wastewater due to its environmental friendliness, larger surface area, reusability, and cost-effectiveness at an industrial scale.
1. Introduction
Aquatic pollution, stemming from industrialization, poses a significant threat to ecosystems.1 Addressing this issue through water treatment is crucial.2 Water systems receive substantial amounts of harmful pollutants, including heavy metals, antibiotics, and dyes, which can permeate the entire ecosystem.3 Human activities introduce metallic ions into aquatic environments and the food web.4 Even trace amounts of heavy metals in water can be toxic to organisms, as these substances are not biodegradable and are soluble in aqueous mediums.5 Heavy metals toxicity cause diseases like brain disorders, joint and muscle pain, gastro, intestinal and eye-sight problems.6 A number of methods are applied for the removal of heavy metals from water inclusive of evaporation, coagulation, membrane separation, ion exchange, electro deposition, precipitation,7 electro dialysis, ultrafiltration,1 and flocculation.3 Conventional methods are expensive, greater extent of energy and reagents are required. Because the amount of heavy metals in water is incredibly low i.e. less than 100 ppm, that's why, these conventional procedures do not produce noticeable results. In case of heavy metal removal from aqueous medium bio-adsorption outcomes are astonishing. Bio-adsorption is stated as a physio-chemical method in which bio materials are employed for the elimination of substances from aqueous mediums.7 In addition, it is a biocompatible, simple process, cheap (due to easy availability of agrowaste)1 and works under the sludge free conditions.8–10 Adsorbents can be recycled by desorption process for further use.11,12 Biomaterials from industrial and agricultural sector can be used as bio-adsorbents,13,14 peanut shells, soybean hulls, corncobs,15 microalgae biomass is also reported.16 Isolation of heavy metals including Cs, Pb, Cu, Ni and As is reported by pine cone as bio-adsorbent.17 Corncobs3 and carboxyl modified cornstalk has also been reported as bio-adsorbent for retrieval of Ga(III).18
Iron oxides are classified as antiferromagnetic hematite (Fe2O3), paramagnetic, ferromagnetic maghemite, super-magnetic magnetite (Fe3O4) and orthorhombic structures.19 Magnetite NPs have outstanding properties like synthesized cheaply and easily, have small size, high magnetism, biocompatible, microwave absorption properties,20 surface chemical properties, catalytic properties, better stability and can be recovered easily by using external magnet.21 These properties widen the applications of magnetite nanoparticles i.e. biomedical applications containing diagnosis, bio-imaging, drug delivery, gene delivery, tumor therapy, cell separation,22 disciplines including physics, chemistry, industry, medicine, material sciences,20 hard drives for storage of data, degradation of dyes, environmental bioremediation,19 water pollution removal of organic and inorganic contaminants.23
Ziziphus mauritiana (Ber) fruit contains vitamin A, C and B complexes along with minerals. Its leaves contain 5.6% digestible crude protein and 49.7% of total digestible nutrients. Extract of Ziziphus mauritiana leaves have medicinal importance and theses are used to cure cuts and wounds and in various remedies.24 Magnetite nanoparticles fabricated by using Ziziphus mauritiana leaf extract and their nanocomposite with corncob are assumed to have effective bio-adsorption properties against heavy metal removal from aqueous solution. The goal of this research is to fabricate magnetite/corncob nanocomposites from biochar and magnetic NPs. Biochar is defined as pyrogenic carbon prepared by thermal conversion of lignocellulose biomass in an atmosphere of oxygen free or limited oxygen. Pristine biochars have low adsorption values.25 Agglomeration of magnetite nanoparticles leads to formation of aggregates. These aggregates inhibits the reactivity and mass transfer kinetics of magnetite NP's.26 Hence, composite fabrication by combining magnetite NPs and biochar is a good technique for the adsorptive removal of heavy metals.17 As reported by D. Kołodyńska et al.,27 Fe3O4 nanocomposite shows good adsorption capacities of phosphates i.e. 7.5% (for pristine biochar) to 67.3%.
2. Materials and methods
2.1. Materials and chemicals
Ziziphus mauritiana leaves were collected for synthesis of extract, while corncobs from a local marketplace served for biomass and biochar preparation. Chemicals, including ferric chloride hexahydrate (FeCl3·6H2O), sodium hydroxide (NaOH), hydrochloric acid (HCl), Acetone, and Ethanol, were used without further refinement as they were of analytical grade. An electronic balance (0.1–320 g range) was utilized for precise measurements. Bio-adsorption experiments for heavy metals were conducted in an orbital shaker (300 rpm) at room temperature in titration flasks, with pH maintained using a HANNA pH 20 pH meter. Manual sieving through a nano-range sieve yielded magnetite/corncob biochar nanocomposite nano powder.
2.2. Synthesis of corncob biomass and biochar
Corncobs were sourced from a nearby market for this study. To eliminate impurities, the corncobs underwent a thorough rinsing with deionized water. Afterward, they were dried in an oven until a constant weight was achieved. The corncobs were initially crushed to a specific size using an iron pestle and mortar, and further grinding was performed until a consistent particle size was attained. Approximately 200 grams of processed biomass were prepared for pyrolysis. A muffle furnace was employed to carbonize corncobs under restricted oxygen conditions at a temperature of 700 °C, a process lasting approximately 5 hours.
2.3. Plant leaves extract mediated magnetite nanoparticle synthesis
Approximately 20–30 grams of Ziziphus mauritiana Lam. plant leaves were washed and immersed in 400 mL of deionized water within a 500 mL beaker. After one hour of soaking at 80 °C, the plant leaf extract was filtered and stored at low temperature. Magnetite nanoparticles were synthesized by combining FeCl3·6H2O sonicated solution with the leaf extract and heating at 70 °C for 2 hours with constant magnetic stirring. KOH (1 M) maintained the reaction pH until it turned black. The temperature was then increased to 90 °C. Precipitates were separated using an external magnet and dried for 24 hours at 60 °C, followed by storage in an airtight container for future use (Fig. 1).20,28
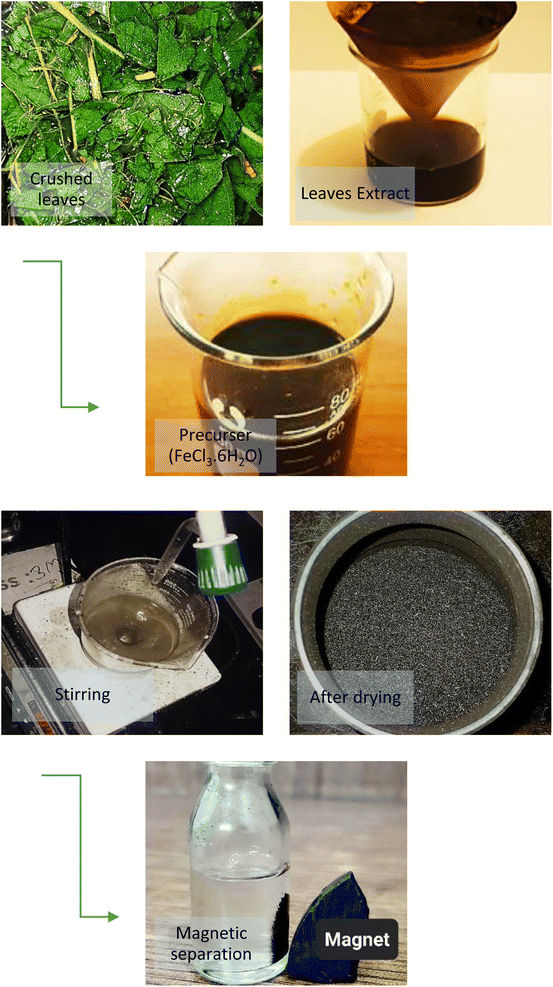 |
| Fig. 1 Detailed process for the synthesis of magnetic nanoparticles. | |
2.4. Magnetite/corncob biochar NC synthesis
The corncob biochar, processed into nano-sized powder, was sifted through a nano-sieve. A ratio of 1
:
5 was maintained for the magnetite/corncob nanocomposite. The 1
:
5 ratios for the magnetite/corncob nanocomposite was maintained to optimize structural stability and enhance the synergistic effects of magnetic responsiveness and adsorption capacity. This specific ratio ensures an efficient balance between the magnetic properties of magnetite and the surface area provided by corncob, resulting in an effective composite for the removal of chromium(VI) and nickel(II) from unhygienic water sources. Two grams of magnetite nanoparticles were combined with distilled water to create a suspension. Ten grams of the nano-powder were then added to the suspension, and the setup was placed in a shaker at 313 rpm for 4 hours at 25 °C. Using an external magnet, the magnetite/corncob nanocomposite settled, allowing the removal of the upper water phase. The residue was subsequently dried in an oven, and the solid nanocomposite was stored in airtight containers for future use.29
2.5. Stock solution preparation and batch biosorption studies
Stock solution of nickel(II) sulphate and potassium dichromate were prepared for parametric studies. 1000 ppm solutions of nickel(II) and chromium(VI) were prepared by dissolving 4.47 grams of nickel sulphate, and 5.65 grams of potassium dichromate into 1 litter of deionized water respectively. The study examined the relationship between initial heavy metal concentrations (10, 20, 40, 60, 80 ppm), contact times (30, 60, 120, 180, and 240 min), and dosing rates (0.05 to 0.1 grams). pH control was maintained with 1 molar solutions of sodium hydroxide and hydrochloric acid. The flasks were shaken continuously for 4 hours. Once the target values were attained, solutions of corncob biomass, corncob biochar, and magnetite/corncob biochar nanocomposites were filtered using filter paper.
2.6. Study of equilibrium
2.6.1. Langmuir isotherm.
The Langmuir equation describes the relationship between molecular exposure in a solid environment and the intermediate concentration at a constant temperature. An extended linear method of calculation was used to calculate the maximum dose of monolayer adsorption, q (mg/g), and other parameters in the Langmuir model. | Ce/qe = 1/XmKL + Ce/Xm | (1) |
where Ce denotes the aqueous phase equilibrium concentration of heavy metals (mg L−1) and Xm signifies the concentration of a complete monolayer (mg g−1). The Langmuir constant is KL. The Langmuir isotherm hypothesis posits that adsorbed molecules are distributed in a monolayer over a homogeneous adsorbent surface. Adsorption is thought to occur on a homogeneous surface within the adsorbate. There is no more adsorption at a location after a sorbate molecule has occupied it.30,31
2.7. Kinetic modelling
2.7.1. Pseudo 1st order kinetics.
The Lagergren model, explains the 1st-order adsorption of a given solute on the adsorbent. The linear form of PFO is as follows: | qt/qe + ln(qe − qt) = ln qe − k1t | (3) |
where qt (mg/g) is the adsorption value in time (min), and q (mg/g) is the adsorption value in the ratio, and K1 (min−1) is the adsorption value of the first order immutable bullet. Log sections (qe − qt) vs. t are used to calculate K1 and qe values.
The rate is assumed to be equal to the number of vacant posts in the model. The first order k1 and the estimated adsorption capacity were calculated using the slopes and subtitle of the log section (qe − q) vs. t.
2.7.2. Pseudo 2nd order equation.
The pseudo-second-order equation, which is dependent on equilibrium adsorption power, can be represented asThe adsorption rate of the pseudo second order K2 (mg g−1 min−1) can be measured from the t/qt interval against the structure, and K2 and qe can be calculated electronically from the t/qt interval against the t/qt slope and structure. The solute adsorption rate is equal to the number of sites displayed in the adsorbent, depending on the PSO model. The reaction rate is equal to the number of active sites accessible in the adsorbent area, and the driving force (qe − qt) is equal to the solute value in the adsorbent area.
2.8. Characterization methods and equipment used
In the research focused on the sequestration of chromium(VI) and nickel(II) heavy metals various analytical techniques were employed with specific quantitative parameters. X-ray diffraction (XRD) utilized a resolution of approximately 0.02° 2θ, an angular range covering 10 to 90° 2θ, and a step size set at increments of 0.02°, ensuring precise crystal structure identification. Scanning Electron Microscopy (SEM) and Transmission Electron Microscopy (TEM) involved an accelerating voltage ranging from 5 to 30 kV, magnification extending from 10× to 1
000
00× or higher, and a resolution at the sub-nanometer to a few nanometers scale, influencing the level of detail and depth of field in imaging. Fourier-Transform Infrared Spectroscopy (FTIR) employed a spectral resolution of 4 cm−1 or better and a wavenumber range spanning from 4000 to 400 cm−1, facilitating the differentiation of functional groups. These quantitative analytical parameters were pivotal in ensuring the accuracy and precision of the measurements, contributing to a thorough characterization of the magnetite/corncob nanocomposite's structural and functional properties in the successful removal of chromium(VI) and nickel(II) from contaminated water.
3. Results and discussion
3.1. Characterization of bioadsorbent
3.1.1. XRD and UV analysis.
The X-ray powder diffraction results shown in Fig. 2 indicated a strong match with magnetite (Fe3O4) NPs and also revealed an additional crystalline structure, likely a biochar impurity. The 2
:
1 molar ratio of iron(III) to iron(II) used in the coating process strongly suggests magnetite synthesis, prompting a modification of the molar ratio employed in this study to better align with the 2
:
1 ratio characteristic of magnetite. XRD confirmed the mean size of NPs approximately ∼8 and ∼9 nm. The lines are consistent according to the JCPDS no. 03-0863. Furthermore, a magnetic affinity test using a magnet demonstrated a significant attraction, further supporting the likelihood of magnetite production.33 Compared to literature an average size of 13.6 nm was reported in the previous research on magnetic nanoparticles.34
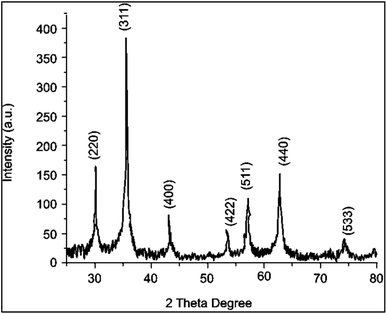 |
| Fig. 2 X-ray powder diffraction of biochar with an iron coating. Miller indices are shown above magnetite identification peaks. | |
Confirmation of magnetite NPs in the first step was done by using UV-VIS equipment. To analyze the optical properties of the synthesized Fe3O4 nanoparticles, a clear colloidal solution was prepared. This solution was obtained through a 30 minute sonication process, wherein Fe3O4 nanoparticles were dispersed in de-ionized water. As a reference, pure de-ionized water was employed. The ensuing absorbance spectrum revealed significant absorption within the visible range of the electromagnetic spectrum. Notably, a distinct absorption peak was conspicuously observed at a wavelength of 238 nanometers (nm). Within this spectral region, the black-colored Fe3O4 nanoparticles exhibited a remarkable and rather broad absorption profile. This spectral analysis serves as a crucial component in understanding the optical characteristics and potential applications of these nanoparticles.35,36 The scanning range adjusted for absorption was 350–550 nm. Magnetite NPs have been conformed ranging from 200–600 nm area as depicted in most of literature. The following UV-VIS conformation is in accordance with literature.35,36 UV spectrum is shown in Fig. 3.
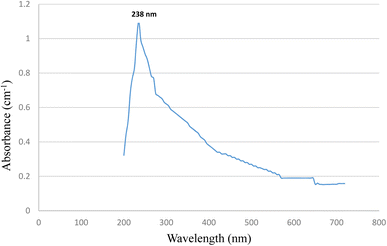 |
| Fig. 3 UV spectra of Fe3O4 NPs. | |
3.1.2. Scanning electron microscopy (SEM), transmission electron microscopy (TEM) and EDX analysis.
The synthesis of stable and fine nanoparticles was facilitated by the use of plant-based chemicals as capping agents. The synthesized magnetite NPs displayed semi-spherical shapes with a narrow size distribution in range of 100–200 nm and magnetite/SCB biochar NC SEM image is shown in Fig. 4 white brightened semi spherical magnetite NPs were also clearly observed on pyrolyzed biochar surface. Spherical shaped morphology was reported in the other researches on magnetite nanoparticles by K. Petcharoen and coworkers.37
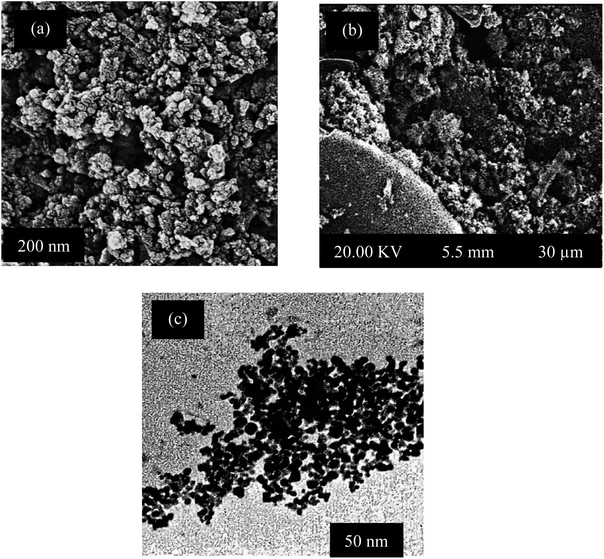 |
| Fig. 4 Scanning electron microscopy (SEM) images of Fe3O4 NPs and magnetite/corncob biochar (a), and (b) and (c) TEM images respectively. | |
TEM analysis further confirmed the nanoscale dimensions, with a representative nanoparticle size of 17 ± 3.49 nm. Moreover, TEM micrographs unveiled fine surface features and agglomeration patterns, underscoring the nanoparticles' polydispersity. Contrast variations in TEM images hint at potential core–shell structures within certain nanoparticles. The clustering observed in Fig. 4(c) may be attributed to molecules from the environment adhering to the nanoparticle surface, seeking a state of equilibrium in intermolecular forces.
Fig. 5 displays the EDX spectrum of magnetite nanoparticles (NPs), where all detected peaks correspond to the K series. Notably, the spectrum distinctly exhibits peaks for iron (Fe) and oxygen (O). An elemental analysis conducted via EDX reveals that iron accounts for approximately 11.26% of the total weight of the magnetite NPs, while oxygen constitutes around 24.19% of the total weight. Additionally, other elements including carbon (C) at 11.7%, chlorine (Cl) at 25.98%, and potassium (K) at 26.87% are also present in the sample, likely originating from the organic compounds used in the coating process.
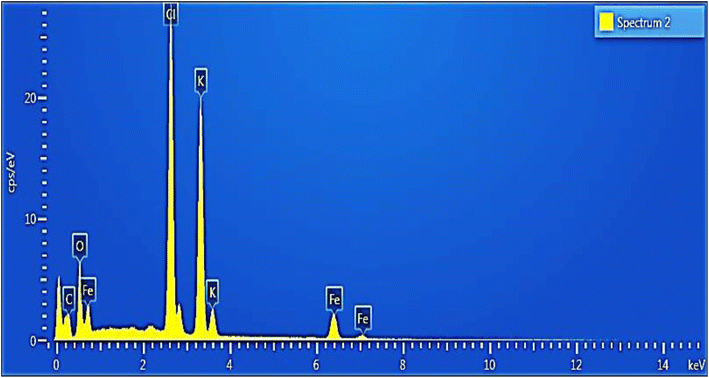 |
| Fig. 5 EDX analytical representation of magnetite/corncob biochar NC. | |
3.1.3. Magnetic properties (VSM).
Vibrating sample magnetometry (VSM) or superconducting quantum interference device (SQUID) measurements were conducted to determine the nanoparticles' magnetic behavior, including saturation magnetization, coercivity, and remanence.38 The Vibrating Sample Magnetometer (VSM) measurements conducted on the synthesized magnetic nanoparticles provided crucial insights into their magnetic properties. The hysteresis loop obtained from the VSM analysis exhibited a saturation magnetization (Ms) value of 45 emu g−1, confirming the nanoparticles' robust magnetic behavior. The coercivity (Hc) value of 120 Oe indicated their susceptibility to external magnetic fields, with reversible magnetization transitions observed. The VSM data substantiated the nanoparticles' superparamagnetic nature, as evidenced by the absence of remanent magnetization and negligible coercivity. These results were in congruence with the nanoparticles' nanoscale dimensions, aligning with the expectation of enhanced magnetic behavior at reduced sizes. The VSM outcomes provide critical information for understanding the nanoparticles' response to magnetic fields, which is pivotal for their potential applications in various domains such as biomedicine and data storage. Fig. 6 depicts vibrating sample magnetometry (VSM) of magnetic nanoparticles.
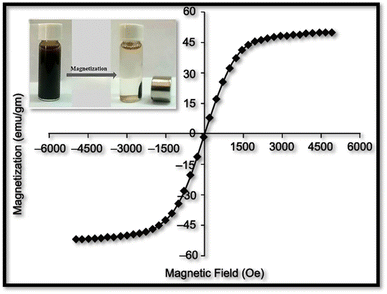 |
| Fig. 6 Vibrating sample magnetometry (VSM) of magnetic nanoparticles. | |
3.1.4. FT-IR spectroscopy.
FT-IR spectra of magnetite/corncob biochar nanocomposite, corncob biochar, Fe3O4 NPs, and raw corncob biomass respectively is shown in Fig. 7. Just a straight line is showing the presence of carbon. A particular peak at 570 cm−1 of Fe3O4 NPs is observed in the spectrum other straight line is just representing carbon. FT-IR bands for Fe3O4 NPs observed at 3352 cm−1, 1582 cm−1 and 593 cm−1. Broad band at 3352 cm−1 represents O–H stretching vibrations while band at 1582 cm−1 credited to the aromatic –C
C– or carbonyl functionalities owing to biomolecules. Magnetite shows very sharp band which is characteristic peak for the Fe–O vibrations.39 This FT-IR spectrum is in accordance with previous studies.35,40
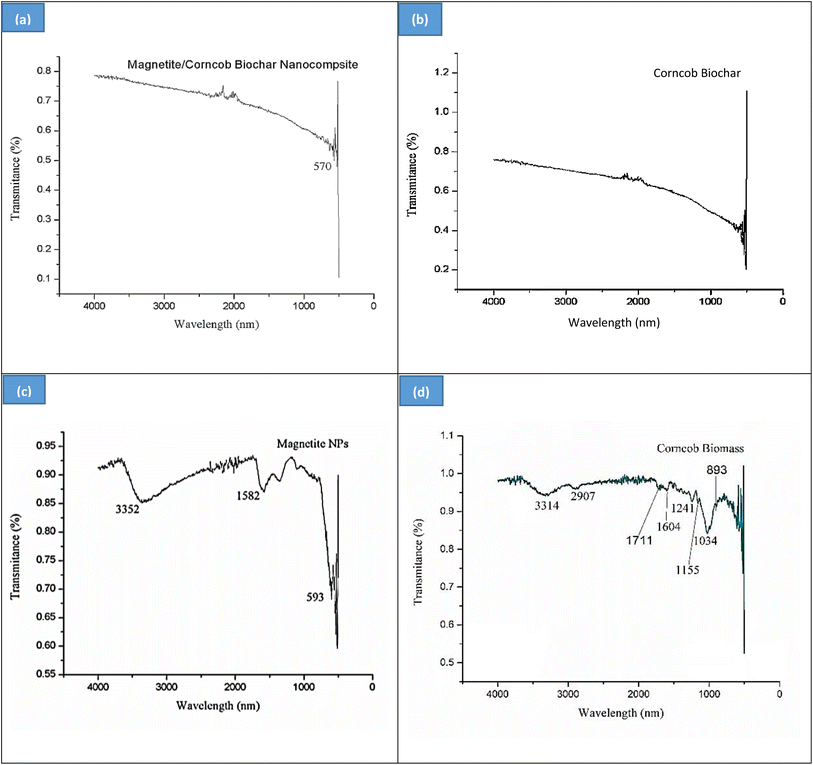 |
| Fig. 7 FT-IR (a) spectra of magnetite/corncob biochar nanocomposite, (b) corncob biochar, (c) Fe3O4 NPs, and (d) raw corncob biomass respectively. | |
Peaks at 893 cm−1 and 1034 cm−1 are identifying β-(1–4) glycosydic linkage. Most of small peaks in between 1250–1600 cm−1 are representing presence of cellulose. The primary OH of cellulose represents a specific plane bending at 1155 cm−1 of C–O–H. Hemicellulose shows a specific peak at 1711 cm−1. Peaks near 1600 and at 1604 cm−1 represents the aromatic presence of lignin. 2907 cm−1 is the stretching vibration of C–H bond in cellulose. 3314 cm−1 depicts the O–H functionality of cellulose and hemicellulose. FT-IR of raw corncob under this study show resemblance with literature.41–43
3.2. Optimization of process parameters
3.2.1. Effect of pH.
This study investigated the influence of pH levels on the removal of Cr(VI) and Ni(II) ions using various adsorbents, including corncob biomass, corncob biochar, and a magnetic/corncob biochar nanocomposite. The experiment covered pH values of 2, 5, 8, and 10. The results indicated that at lower pH values, all three adsorbents exhibited higher efficiency in removing Cr(VI), with the magnetic nanocomposite achieving 96% removal at pH 2 and 90% at pH 10. Similarly, biomass and biochar demonstrated a similar trend, with 95% removal at pH 2 and a slight decrease to 89% at pH 10 for biomass and biochar. Notably, at lower pH values, specifically pH 2, all three adsorbents exhibited heightened efficiency in Cr(VI) removal. The magnetic nanocomposite achieved an impressive 96% removal, while biomass and biochar showed a slightly lower but still substantial removal efficiency of 95%. As the pH increased, the removal efficiency of all three adsorbents declined. At pH 10, the magnetic nanocomposite demonstrated 90% removal, indicating a decrease compared to its performance at lower pH. Similarly, biomass and biochar exhibited a trend of diminishing efficiency at higher pH, reaching 89% removal. This pattern suggests that the adsorption of Cr(VI) is most effective under acidic conditions and becomes less efficient as the pH becomes more alkaline.
In the case of nickel(II), in an acidic environment at pH 3, all adsorbents exhibited excellent uptake capacity (q = 95 mg L−1) and 95% removal. However, as the pH increased to 5, 8, and 10, a notable increase in both removal percentage and uptake capacity was observed, particularly in alkaline conditions. The transition from pH 8 to 10 indicated equilibrium. This trend may be attributed to the competition between hydrogen ions and heavy metal ions for active sites on the adsorbent surface, leading to reduced efficiency of pollutant ion adsorption at lower pH levels. Conversely, at elevated pH levels, the surfaces of biomass, biochar, and nanocomposites acquired a net negative charge, enhancing Ni2+ ion adsorption through electrostatic interactions. Additionally, within this pH range, the precipitation of Ni (OH)2 on the biochar surfaces further contributed to the significant removal of Ni2+ ions from the solution.28Fig. 8 shows the effect of pH on adsorption of (a) chromium(IV) and (b) nickel(II) respectively.
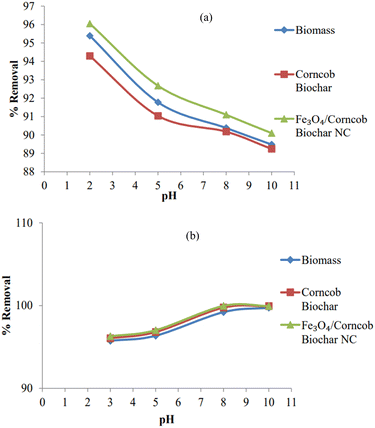 |
| Fig. 8 Effect of pH on adsorption of (a) Cr(IV) and (b) Ni(II). | |
3.2.2. Effect of initial concentration.
The study examined the influence of the initial concentration of Cr(VI) ions on their adsorption process, specifically investigating concentrations of 10 ppm, 20 ppm, 40 ppm, and 60 ppm. The results revealed a clear pattern: as the concentration of Cr(VI) increased, both the removal percentage and the uptake capacity (q mg g−1) exhibited a significant increase. This initial concentration factor is closely linked to the adsorbent's characteristics, particularly the availability of functional groups over time. Various adsorbents, including corncobs biomass, corncob biochar, and magnetite/corncob nanocomposite, were found to possess diverse functional groups. Formerly, the optimal pH level for Cr(VI) removal was established at a concentration of 100 ppm, achieving a 96% removal rate. However, with a duration of 240 minutes, both the uptake capacity and percentage removal continued to rise. For example, at an initial concentration of 60 ppm, the biomass displayed a q value of 47.452 mg g−1 and a removal rate of 80.25%. Biochar exhibited a similar trend, with a q value of 46.30 mg g−1 and a removal rate of 78% at 60 ppm. Notably, the nanocomposite, with its enhanced surface area and magnetic properties, outperformed the others, demonstrating a q of 48.48 mg g−1 and an impressive 82% removal rate at the same 60 ppm Cr(VI) initial concentration.
The variance in the adsorption of chromium(VI) ions on the activated carbon samples can be attributed to differences in surface area and total pore volume. As the concentration of Cr(VI) ions increases, active sites on the surface become saturated with these ions, resulting in a higher uptake of metal ions from the solution. Additionally, higher initial Cr(VI) concentrations enhance the interaction between Cr(VI) ions in the aqueous phase and the surface of the biomass, leading to a more efficient uptake of Cr(VI) for a given quantity of biomass.9 To explore the impact of the initial concentration of Ni(II) ions in solution, concentrations of 10 ppm, 20 ppm, 40 ppm, and 80 ppm were prepared. The quantity of adsorbent used is directly linked to this parameter optimization. Specifically, 0.1 gram of adsorbent yielded q values ranging from 6.90 to 74.94 as the initial concentration increased. Adsorbents with higher surface areas and more available adsorption sites displayed a q value of 74 mg g−1 for the 80 ppm solution. Considering this parameter, corncob was identified as an effective adsorbent for Ni(II) ions. Fig. 9 demonstrates the effect of initial Concentration on adsorption of (a) Ni(II) and (b) Cr(IV) respectively.
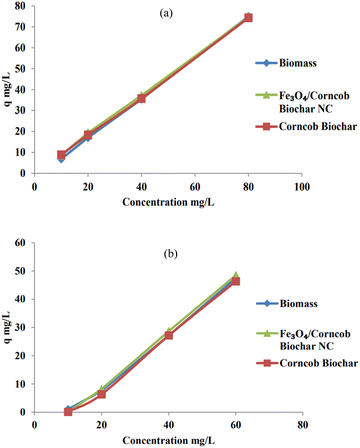 |
| Fig. 9 Effect of initial concentration on adsorption of (a) nickel(II) and (b) chromium(IV). | |
3.2.3. Effect of contact time.
Graphical representation implied that efficiency of Cr(IV) removal grew steadily as contact time increased. The Cr(IV) removal efficiencies for 30, 60, 120 and 180 min were studied by corncob biomass, corncob biochar and magnetite/corncob NC. The longer metal (Cr(IV)) ion is in contact with adsorbent, the more likely it is to adsorb with the adsorbent. With increasing contact time up to 180 minutes, Cr(IV) removal percentages were also increased. So, it was observed that most of the Cr(IV) was removed when it was in contact with the adsorbent for 180 minutes. The uptake capacity for biomass at 180 min is 6.037 mg L−1 and %age removal is 79.84. Biochar shows 6.15 mg L−1 of uptake capacity along with % age removal of 81%. Similarly, magnetite/corncob biochar NC, if we compare all the adsorbents showed best results i.e. uptake capacity of adsorbent is 6.27 mg L−1 and %age removal of 83.9 A contact time of 30–180 minutes has been selected and uptake increased rapidly in between 60–120 minutes and then starts to decrease showing equilibrium is established and most of the Ni(II) has been adsorbed on the active sites of adsorbents. Hence, optimum time for Ni(II) is in range from 60–120 minutes. Fig. 10 shows the effect of contact time on adsorption of (a) chromium(IV) and (b) nickel(II) respectively.
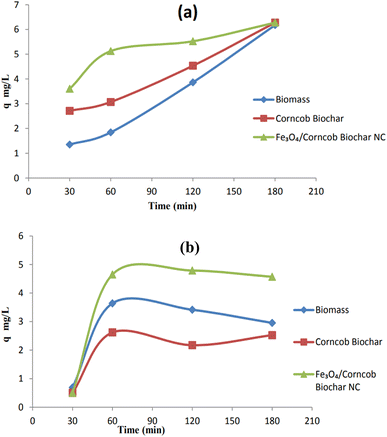 |
| Fig. 10 Effect of contact time on adsorption of (a) chromium(IV) and (b) nickel(II). | |
3.2.4. Effect of dose rate.
The impact of varying the amount of adsorbent on Cr(VI) removal from aqueous solutions was investigated in a controlled experiment. A titration flask containing 50 mL of Cr(VI) solution was used, and different quantities of adsorbent (0.05 g, 0.07 g, 0.1 g, and 0.2 g) were added. The initial pH was set at 2, and the initial concentration of Cr(VI) in the solution was 100 ppm. This mixture was left to sit for 240 minutes. As the amount of adsorbent increased, the efficiency of removal also increased. The highest removal efficiency was observed when 0.2 g of any of the three adsorbents (corncob, corncob biochar, and magnetite/corncob biochar NC) was used, with removal rates of 92%, 93%, and 94%, respectively. This improvement can be attributed to the increase in adsorbent dosage, providing more available binding sites for Cr(VI). However, further increasing the dosage did not significantly improve adsorption efficiency, indicating a limit to the adsorption capacity of the adsorbents.43
The nanocomposite exhibits superior uptake capacity and percentage removal (q = 96–97) within the range of 0.05 g to 0.1 g. Similarly, biomass and corncob biochar display similar trends. Notably, the uptake capacity does not significantly increase with higher doses of adsorbents. The initial assumption was that increasing the dose would enhance removal and uptake, but instead, there was only a marginal increase in nickel(II) removal. The effectiveness of nickel(II) removal is closely linked to the availability of active adsorption sites and the optimal adsorbent dosage. Increasing the amount of adsorbent up to a certain point increases the availability of adsorption sites, resulting in higher Ni(II) removal. However, if the adsorbent concentration is raised beyond this optimal level, the efficiency of Ni(II) removal tends to plateau or even decline. This phenomenon can be attributed to the saturation and crowding of adsorption sites, leading to reduced Ni(II) removal capacity.7Fig. 11 shows the effect of contact time on adsorption of (a) Cr(IV) and (b) Ni(II) respectively.
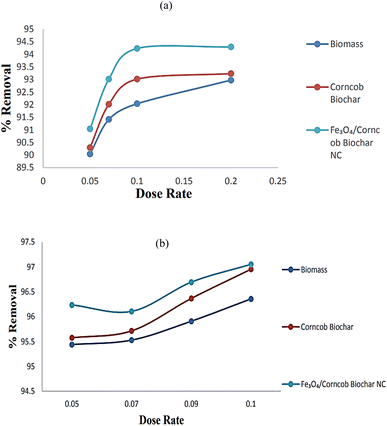 |
| Fig. 11 Effect of dose rate on adsorption of (a) Cr(IV) and (b) Ni(II). | |
3.2.5. Comparison among different adsorbents.
Corncob biomass and corncob biochar derived from agricultural waste, proved to be sustainable and cost-effective with a substantial surface area but showed limited selectivity and slow kinetics. However, magnetic nanocomposites, combining magnetic nanoparticles with corncob biochar, demonstrated superior performance, offering enhanced adsorption capacity, selectivity, and easy separation, making them the optimal choice for efficient metal removal from water. Comparison among different catalyst is shown in Fig. 12.
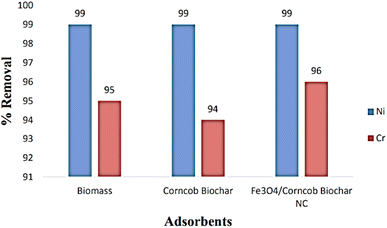 |
| Fig. 12 Comparison between different adsorbents. | |
3.3. Equilibrium models for adsorption
3.3.1. Langmuir isotherm model for Cr(IV) and Ni(II).
The Langmuir isotherm theory posits that adsorption occurs on a surface where a single layer of molecules adheres to a uniform adsorbent surface. This theory assumes that adsorption happens exclusively on a particular, uniform surface within the adsorbent. According to Langmuir's model, once a sorbate molecule occupies a site on this surface, further adsorption at that site is prohibited. However, it's worth noting that the Langmuir model doesn't adequately describe the adsorption behavior of Ni(II) on the surface of adsorbents, as indicated by the very low R2 values, which hover around 0.1.1Fig. 13 shows Langmuir isotherm model for Cr(IV) and Ni(II).
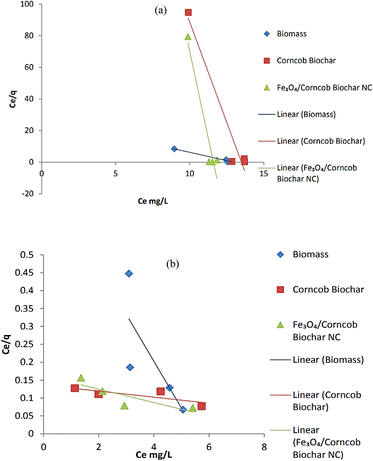 |
| Fig. 13 Langmuir isotherm model for (a) Cr(IV) and (b) Ni(II). | |
3.3.2. Freundlich isotherm for Cr(IV) and Ni(II).
Freundlich isotherm was used to non-ideal sorption on heterogeneous surfaces and to a multilayer sorption, proposing that binding sites were not equivalent and/or independent. Ni(II) removal follows Freundlich model as R2 = 0.91, 0.95 and 0.96 respectively for biomass, biochar and magnetite/corncob biochar NC.9Fig. 14 shows Freundlich isotherm for Cr(IV) and Ni(II) (Table 1).
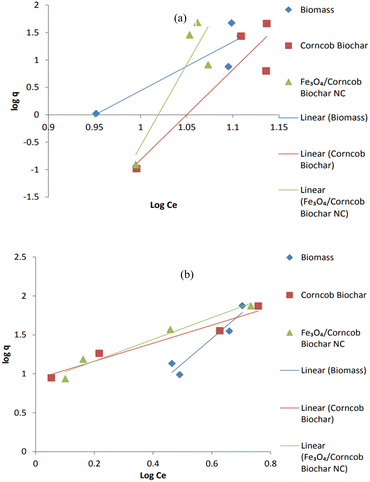 |
| Fig. 14 Freundlich isotherm for (a) Cr(IV) and (b) Ni(II). | |
Table 1 The comparison of equilibrium isotherms for Cr(IV) and Ni(II) given in table
Adsorbate |
Adsorbent |
Langmuir model |
Experimental value q (mg g−1) |
Freundlich model |
X
m (mg g−1) |
K
L (L mg−1) |
R
2
|
q
e (mg g−1) |
1/n |
K
F (mg g−1) |
R
2
|
Cr(VI) |
Biomass |
0.47 |
0.07 |
0.98 |
47.45 |
2.5 × 1021 |
8.87 |
2.13 |
0.81 |
Corncob biochar |
0.03 |
0.07 |
0.94 |
46.30 |
4.6 × 1040 |
16.42 |
2.84 |
0.84 |
Fe3O4/corncob biochar NC |
0.02 |
0.08 |
0.92 |
48.48 |
2.9 × 1070 |
29.71 |
3.41 |
0.78 |
Adsorbate |
Adsorbent |
Langmuir model |
Experiment al value q (mg g−1) |
Freundlich model |
X
m (mg g−1) |
K
L (L mg−1) |
R
2
|
q
e (mg g−1) |
1/n |
K
F (mg g−1) |
R
2
|
Ni(II) |
Biomass |
7.65 |
0.18 |
0.47 |
74.94 |
145.17 |
3.2 |
−0.74 |
0.91 |
Corncob Biochar |
119.04 |
0.06 |
0.26 |
74.27 |
379.002 |
1.15 |
−0.07 |
0.95 |
Fe3O4/corncob biochar NC |
53.47 |
0.11 |
0.010 |
74.59 |
588.12 |
1.39 |
−0.12 |
0.96 |
3.4. Kinetic studies of adsorption for Cr(IV) and Ni(II)
It was assumed that the rate of a certain process was directly related to the number of vacant sites within the model. To determine this relationship, we calculated two important parameters: the first-order rate constant (KL) and the equilibrium adsorption capacity (qe). These values were derived by analyzing the plot of the natural logarithm of (qe − q) against time (t), taking into account the slopes and intercepts. However, when we compared the R-squared (R2) values between the pseudo-first order and pseudo-second order kinetics for Cr(IV), we observed a significant difference. Specifically, the R2 values for the pseudo-first order kinetics were notably lower than those for the pseudo-second order kinetics. This discrepancy clearly indicates that the pseudo-first order kinetic equation is inadequate for accurately predicting the experimental adsorption capacity (qe × p) for NC.
Corncob biomass showed R2 = 0.98 while corncob biochar also showed strong R2 = 0.84 assuming physical adsorption happening. Corncob biomass did not follow pseudo 2nd order (PSO) but corncob biochar and magnetite/corncob biochar NC both followed PSO with R2 = 0.88 and 0.99 respectively (Table 2). As surface modification cause changes in surface behaviors. Corncob biochar showed strong R2 value for both PFO and PSO. As corncob biochar was also the main component for magnetite/corncob biochar NC. These facts indicated that the 2nd order kinetic model which was predicted on assumption that adsorption might be a rate-limiting step process, does not accurately describe the adsorption of heavy metals.31,44 Pseudo 1st and 2nd order plots for (a) Cr(IV) and (b) Ni(II) are depicted in Fig. 15 and 16 respectively.
Table 2 The comparison of pseudo first and second order kinetics for Cr(IV) and Ni(II) was given in table
Adsorbate |
Adsorbent |
Pseudo first order |
Pseudo second order |
q
e (mg g−1) |
K
1ad (min−1) |
R
2
|
q
exp (mg g−1) |
q
e (mg g−1) |
K
2ad (mg g−1 min−1) |
R
2
|
Chromium(VI) |
Biomass |
7.18 |
0.0019 |
0.98 |
5.90 |
52.63 |
1.3 × 10−5 |
0.08 |
Corncob biochar |
10.64 |
0.0041 |
0.84 |
6.02 |
8.68 |
0.0012 |
0.88 |
Fe3O4/corncob biochar NC |
1.96 |
0.0010 |
0.41 |
6.14 |
7.04 |
0.0048 |
0.99 |
Adsorbate |
Adsorbent |
Pseudo first order |
Pseudo second order |
q
e (mg g−1) |
K
1ad (min−1) |
R
2
|
q
exp (mg g−1) |
q
e (mg g−1) |
K
2ad (mg g−1 min−1) |
R
2
|
Ni(II) |
Biomass |
3.53 |
0.00073 |
0.86 |
6.18 |
3.74 |
0.00756 |
0.64 |
Corncob biochar |
1.63 |
0.0025 |
0.90 |
6.67 |
3.41 |
0.00385 |
0.51 |
Fe3O4/corncob biochar NC |
4.27 |
8.684 |
0.90 |
7.08 |
8.48 |
0.0008 |
0.51 |
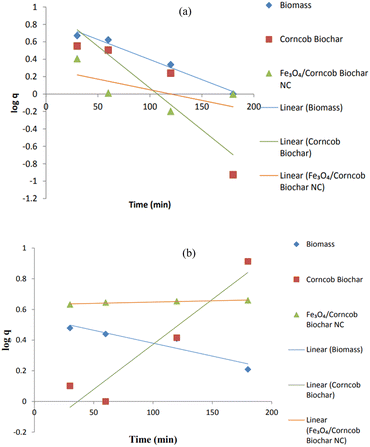 |
| Fig. 15 Pseudo first order plot (a) Cr(IV) and (b) Ni(II). | |
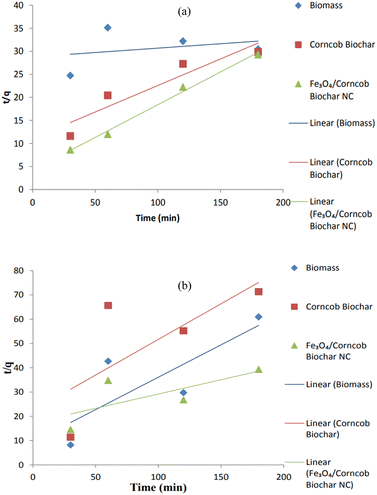 |
| Fig. 16 Second order plot (a) Cr(IV) and (b) Ni(II). | |
3.5. Differences between the removal capacity of Ni(II) and Cr(IV)
3.5.1. Corncob biomass.
For chromium(VI), the biomass achieved a higher removal capacity (qe) of 7.18 mg g−1 compared to nickel(II) with a qe of 3.53 mg g−1 in the pseudo first-order kinetics. The same trend was observed in the pseudo second-order kinetics, where the qe for chromium(VI) is 52.63 mg g−1, significantly higher than nickel(II) with a qe of 3.74 mg g−1. The rate constant (K1ad) for the pseudo first-order kinetics was higher for nickel(II) (0.00073 min−1) than for chromium(VI) (0.0019 min−1), indicating a faster initial adsorption rate for nickel(II) on biomass. However, the pseudo second-order kinetics rate constant (K2ad) is higher for chromium(VI) (1.3 × 10−5 mg g−1 min−1) than nickel(II) (0.00756 mg g−1 min−1), suggesting a more efficient adsorption process for chromium(VI) at later stages.
3.5.2. Corncob biochar.
Similar to biomass, corncob biochar exhibits a higher removal capacity for chromium(VI) (qe of 10.64 mg g−1) compared to nickel(II) (qe of 1.63 mg g−1) in the pseudo first-order kinetics. In the pseudo second-order kinetics, the qe for chromium(VI) is 8.68 mg g−1, significantly surpassing the qe for nickel(II) at 3.41 mg g−1. The rate constants also show differences, with the pseudo first-order kinetics rate constant (K1ad) being higher for nickel(II) (0.0025 min−1) than for chromium(VI) (0.0041 min−1). However, the pseudo second-order kinetics rate constant (K2ad) is higher for chromium(VI) (0.0012 mg g−1 min−1) than nickel(II) (0.00385 mg g−1 min−1).
3.5.3. Fe3O4/corncob biochar NC.
For this adsorbent, the removal capacity (qe) for chromium(VI) is lower (1.96 mg g−1) compared to nickel(II) (4.27 mg g−1) in the pseudo first-order kinetics. However, in the pseudo second-order kinetics, the qe for chromium(VI) is higher (7.04 mg g−1) than nickel(II) (8.48 mg g−1). The rate constants indicate that the pseudo first-order kinetics rate constant (K1ad) is higher for nickel(II) (8.684 min−1) compared to chromium(VI) (0.0010 min−1). The pseudo second-order kinetics rate constant (K2ad) is higher for chromium(VI) (0.0048 mg g−1 min−1) than nickel(II) (0.0008 mg g−1 min−1).
3.6. Specific surface area
The BET surface area of the magnetic nanocomposite was determined to be 44.33 m2 g−1 in the relative pressure range from 0.0 to 1.0, indicating a favorable scenario for heavy metal removal. The relatively high surface area suggested abundant active sites available for adsorption, implying a substantial adsorption capacity and efficient removal of heavy metal ions from aqueous solutions (Fig. 17).
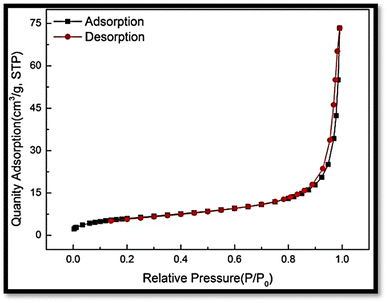 |
| Fig. 17 Specific surface area (BET analysis). | |
3.7. Desorption test
Approximately 0.15 g each of biomass, biochar, and magnetite/corncob nanocomposite were individually introduced into 60 mL of a solution containing 60 mg L−1 heavy metals within a 300 mL flask. The solution's pH was adjusted to 3.21, 4.35, and 2.15 for biochar, biomass, and NC, respectively, corresponding to the pH at which maximum adsorption occurred for each. The mixture was stirred for 1 h, followed by centrifugation at 350 rpm for 40 min, and the remaining heavy metal concentration was measured at 500 nm. The metal-loaded adsorbents were rinsed with water, dried at 75 °C to complete dryness, and then immersed in 120 mL of water for the desorption process. After vigorous shaking for 40 min, centrifugation was performed, and the desorbed heavy metal concentration was determined by the following equation: |  | (5) |
The percentage desorption sequence is biochar (89.24%) > NC (82.36%) > biomass (67.45%). Desorption exceeding 80% suggests that biochar and NC can be readily regenerated for additional adsorption cycles. The relatively higher desorption percentages for biochar and NC imply a weak bond between heavy metals and these materials, indicative of physiosorption. Conversely, the lower desorption percentage for biomass suggests a strong chemical adsorption process with heavy metals tightly bonded to the biomass (Fig. 18).
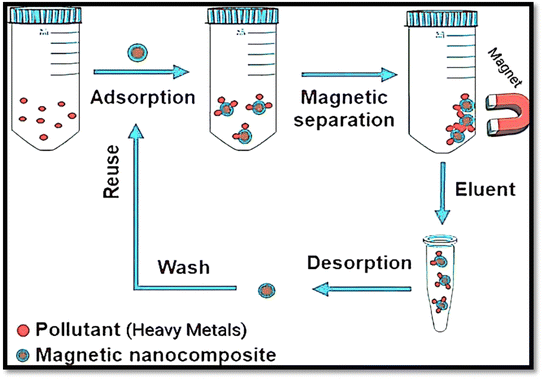 |
| Fig. 18 Desorption and recycling of adsorbent. | |
3.8. Innovation
This research introduces a groundbreaking innovation in the field of heavy metal remediation by employing a green chemistry approach for the synthesis of a magnetite nanocomposite. The key innovation lies in the utilization of Ziziphus Mauritania Lam. leaves extract to facilitate the reduction of FeCl3·6H2O salt, resulting in the eco-friendly synthesis of magnetite nanoparticles (NPs). These NPs are then ingeniously incorporated into finely powdered and pyrolyzed corncob biochar, forming a novel nanocomposite material.
The use of magnetite nanocomposites represents a significant advancement over traditional adsorbents. The integration of magnetite NPs within the corncob biochar matrix enhances the adsorption efficiency for toxic pollutants such as chromium(VI) and nickel(II). The nanocomposite exhibits several distinctive features, including a larger surface area, which enhances adsorption capacity, and a sustainable synthesis process that utilizes natural extracts, aligning with the principles of green chemistry.
Furthermore, the study provides a comprehensive understanding of the adsorption kinetics and equilibrium of chromium(VI) and nickel(II) on the developed nanocomposite. The application of kinetic models and isotherm studies contributes to the innovative design of an adsorbent tailored for optimal heavy metal removal efficiency. The synthesized nanocomposite's remarkable performance, coupled with its environmental friendliness, reusability, and cost-effectiveness at an industrial scale, positions it as a pioneering solution for addressing heavy metal contamination in wastewater. This innovation opens new avenues for sustainable water treatment technologies, emphasizing the potential of magnetite nanocomposites as a transformative approach in the realm of environmental remediation.
4. Conclusion
This research exploited green chemistry approach to engineer magnetite nanoparticles for effectively trapping chromium(VI) and nickel(II) from unhygienic water. These nanoparticles seamlessly integrated with finely powdered and pyrolyzed corncob biochar in a 5
:
1 ratio, forming a potent nanocomposite. Our comprehensive analysis, spanning UV-vis spectroscopy, FT-IR, SEM, TEM, and EDX, validated the successful synthesis. Conclusively, the analysis affirmed the nanoscale dimensions, indicating a nanoparticle size of 17 ± 3.49 nm. The VSM analysis revealed a saturation magnetization (Ms) value of 45 emu g−1, confirming vigorous magnetic behavior in the nanoparticles. Equilibrium and kinetic studies provided valuable insights into the adsorption process. For Cr(VI), the adsorption data closely adhered to the pseudo 1st order model for corncob biomass, while biochar and nanocomposite exhibited strong adherence to the pseudo 2nd order model. In case of Ni(II) the suitability of the Freundlich isotherm model was observed to be effective for all bio-adsorbents. The magnetic nanocomposite exhibited a BET surface area of 44.33 m2 g−1 and demonstrated a comparatively higher adsorptive removal capacity of 99% for Ni and 96% for Cr. In conclusion, all tested adsorbents displayed promising results. However, the nanocomposites emerged as heavy metal removal champions, offering expansive surface area, reusability, cost-effectiveness, and remarkable efficacy against both pollutants. This breakthrough positions magnetic nanocomposites as the future frontier for scalable, eco-conscious industrial applications..
Conflicts of interest
There are no conflicts to declare.
References
- M. Basu, A. K. Guha and L. Ray, J. Cleaner Prod., 2017, 151, 603–615 CAS.
- G. Vilardi, L. Di Palma and N. Verdone, Chin. J. Chem. Eng., 2018, 26, 455–464 CAS.
- L. Zheng, D. Peng and P. Meng, Colloids Surf., A, 2019, 561, 109–119 CAS.
- N. Blagojev, D. Kukić, V. Vasić, M. Šćiban, J. Prodanović and O. Bera, J. Hazard. Mater., 2019, 363, 366–375 CAS.
- G. Vilardi, J. M. Ochando-Pulido, N. Verdone, M. Stoller and L. Di Palma, J. Cleaner Prod., 2018, 190, 200–210 CAS.
- V. Gupta, J. Sandesh and N. Chandra, Int. J. Sci. Res. Sci. Eng. Technol., 2018, 5, 169–174 Search PubMed.
- N. E. Ibisi and C. A. Asoluka, Chem. Int., 2018, 4, 52–59 CAS.
- J. Xu, Z. Cao, Y. Zhang, Z. Yuan, Z. Lou, X. Xu and X. Wang, Chemosphere, 2018, 195, 351–364 CAS.
- A. E. Burakov, E. V. Galunin, I. V. Burakova, A. E. Kucherova, S. Agarwal, A. G. Tkachev and V. K. Gupta, Ecotoxicol. Environ. Saf., 2018, 148, 702–712 CAS.
- A. Ali, K. Saeed and F. Mabood, Alexandria Eng. J., 2016, 55, 2933–2942 Search PubMed.
- M. Hua, S. Zhang, B. Pan, W. Zhang, L. Lv and Q. Zhang, J. Hazard. Mater., 2012, 211, 317–331 Search PubMed.
- S. Yu, X. Wang, H. Pang, R. Zhang, W. Song, D. Fu, T. Hayat and X. Wang, Chem. Eng. J., 2018, 333, 343–360 CAS.
- N. Ünlü and M. Ersoz, J. Hazard. Mater. B, 2006, 136, 272–280 Search PubMed.
- Ç. Kırbıyık, A. E. Pütün and E. Pütün, Water Sci. Technol., 2016, 73, 423–436 Search PubMed.
- C. R. T. Tarley and M. A. Z. Arruda, Chemosphere, 2004, 54, 987–995 Search PubMed.
- G. Ç. Dönmez, Z. Aksu, A. Öztürk and T. Kutsal, Process Biochem., 1999, 34, 885–892 Search PubMed.
- I. L. Ouma, E. B. Naidoo and A. E. Ofomaja, J. Environ. Chem. Eng., 2018, 6, 5409–5419 CAS.
- Y. Zhang, L. Zhu, Y. Wang, Z. Lou, W. Shan, Y. Xiong and Y. Fan, J. Taiwan Inst. Chem. Eng., 2018, 91, 291–298 CAS.
- J. K. Patra and K.-H. Baek, J. Photochem. Photobiol., B, 2017, 173, 291–300 CAS.
- S. Venkateswarlu, Y. S. Rao, T. Balaji, B. Prathima and N. Jyothi, Arabian J. Chem., 2013, 100, 241–244 CAS.
- M. Nasrollahzadeh, S. M. Sajadi, A. Rostami-Vartooni and M. Khalaj, J. Mol. Catal. A: Chem., 2015, 396, 31–39 CAS.
- A. A. Kajani and A.-K. Bordbar, J. Hazard. Mater., 2019, 366, 268–274 Search PubMed.
- C. Prasad, G. Yuvaraja and P. Venkateswarlu, J. Magn. Magn. Mater., 2017, 424, 376–381 CAS.
- R. K. Verma, M. Pandey, M. D. Indoria, R. Singh and S. Suthar, Trop. J. Pharm. Life Sci., 2018, 5, 08–18 Search PubMed.
- S. Wang, B. Gao, Y. Li, A. Mosa, A. R. Zimmerman, L. Q. Ma, W. G. Harris and K. W. Migliaccio, Bioresour. Technol., 2015, 181, 13–17 CAS.
- F. Yang, S. Zhang, Y. Sun, Q. Du, J. Song and D. C. Tsang, Bioresour. Technol., 2019, 274, 379–385 CAS.
- D. Kołodyńska, J. Bąk, M. Kozioł and L. Pylychuk, Nanoscale Res. Lett., 2017, 12, 433 Search PubMed.
- R. M. Al-Bahrani, S. M. A. Majeed, M. N. Owaid, A. B. Mohammed and D. A. Rheem, Acta Pharm. Sci., 2018, 56, 85–92 CAS.
- M. Zhang, B. Gao, S. Varnoosfaderani, A. Hebard, Y. Yao and M. Inyang, Bioresour. Technol., 2013, 130, 457–462 CAS.
- V. Vimal, M. Patel and D. Mohan, RSC Adv., 2019, 9, 26338–26350 CAS.
- M. Kobya, Adsorpt. Sci. Technol., 2004, 22, 51–64 CAS.
- Y. Bulut and H. Aydın, Desalination, 2006, 194, 259–267 CAS.
- H. Cederlund, E. Börjesson, D. Lundberg and J. Stenström, Water, Air, Soil Pollut., 2016, 227, 1–12 CAS.
- F. Y. Zhao, Y. L. Li and L. H. Li, Appl. Mech. Mater., 2014, 618, 24–27 CAS.
- V. A. R. Villegas, J. I. D. L. Ramírez, E. H. Guevara, S. P. Sicairos, L. A. H. Ayala and B. L. Sanchez, J. Saudi Chem. Soc., 2020, 24, 223–235 Search PubMed.
- H. M. Asoufi, T. M. Al-Antary and A. M. Awwad, J. Comput. Biol., 2018, 6, 9–16 Search PubMed.
- K. Petcharoen and A. Sirivat, Mater. Sci. Eng. B, 2012, 177, 421–427 CAS.
- S. Qu, F. Huang, S. Yu, G. Chen and J. Kong, J. Hazard. Mater., 2008, 160, 643–647 CAS.
- V. Alfredo Reyes Villegas, J. Isaías De León Ramírez, E. Hernandez Guevara, S. Perez Sicairos, L. Angelica Hurtado Ayala and B. Landeros Sanchez, J. Saudi Chem. Soc., 2020, 24, 223–235 CAS.
- V. Ranjithkumar, S. Sangeetha and S. Vairam, J. Hazard. Mater., 2014, 273, 127–135 CAS.
- A. Kumar, Y. Negi, V. Choudhary and N. Bhardwaj, J. Hazard. Mater., 2014, 2, 1–8 Search PubMed.
- L. Nalbandian, E. Patrikiadou, V. Zaspalis, A. Patrikidou, E. Hatzidaki and C. Papandreou, Curr. Nanosci., 2015, 12, 1 Search PubMed.
- O. M. Abd Almawgood, S. A. El Tohamy, E. H. Ismail and F. A. Samhan, Egypt. J. Chem., 2021, 64, 1293–1313 Search PubMed.
- A. Buthiyappan, J. Gopalan and A. A. A. Raman, J. Environ. Manage., 2019, 249, 109323 CAS.
Footnote |
† Electronic supplementary information (ESI) available: Sustainability statement, water impact statement, calibration curves and table of bio-adsorbents utilized for sequestrating heavy metals. See DOI: https://doi.org/10.1039/d3na00923h |
|
This journal is © The Royal Society of Chemistry 2024 |
Click here to see how this site uses Cookies. View our privacy policy here.