DOI:
10.1039/D4NH00070F
(Communication)
Nanoscale Horiz., 2024,
9, 1002-1012
Polydopamine-encapsulated zinc peroxide nanoparticles to target the metabolism-redox circuit against tumor adaptability for mild photothermal therapy†
Received
16th February 2024
, Accepted 26th March 2024
First published on 4th April 2024
Abstract
Regulating the metabolism-redox circuit of cancer cells has emerged as an attractive strategy to improve the therapeutic outcome, while often confronting the glaring issue of resistance due to the multiple adaptive responses of tumor cells. This study presents a simple yet efficient approach to regulate this circuit simultaneously against tumor adaptability by utilizing polydopamine-encapsulated zinc peroxide nanoparticles (ZnO2@PDA NPs). The nanoparticles could deliver large amounts of Zn2+ and H2O2 into tumor cells to unfold an intracellular self-amplifying loop for breaking the balance in zinc and redox homeostasis by H2O2-mediated endogenous Zn2+ release from metallothioneins due to its oxidation by H2O2 and Zn2+-induced in situ H2O2 production by disturbing mitochondrial respiration, ultimately disrupting tumor adaptability to exogenous stimuli. The elevated levels of Zn2+ and H2O2 also inhibited adenosine triphosphate (ATP) generation from glycolysis and mitochondrial respiration to disrupt energy adaptability. Furthermore, insufficient ATP supply could reduce glutathione and heat shock protein expression, thereby sensitizing oxidative stress and enabling PDA-mediated mild photothermal therapy (PTT). Consequently, this trinity nanoplatform, which integrated dual-starvation therapy, amplified oxidative stress, and mild PTT, demonstrated outstanding therapeutic effects and a facile strategy.
New concepts
The majority of current therapeutic strategies, including starvation therapy, oxidative stress therapy, hyperthermia, etc., confront the glaring issue of tumor resistance due to the multiple adaptive responses of tumor cells. Reverse tumor resistance to treatment has persistently been a formidable challenge with anti-tumor agents. Metabolic reprogramming and elevated levels of reactive oxygen species (ROS) are two distinct traits observed in cancer. The interdependent relationship between these two traits establishes a crucial metabolism-redox circuit, which provides tumors with survival advantages and promotes resistance to oncotherapy. In this study, we have successfully employed an extremely simple design – zinc peroxide nanoparticles encapsulated in a polydopamine shell (ZnO2@PDA NPs), ingeniously utilizing the self-amplifying loop of zinc ions and hydrogen peroxide to effectively target the tumor energy metabolism-redox circuit, thus effectively overcoming the adaptability of multiple antitumor therapies. Thus, this simple and efficient design can serve as a promising strategy for reverse tumor resistance, offering new insights for the design of future antitumor strategies.
|
Introduction
Cancer cells prefer aerobic glycolysis over mitochondrial respiration under metabolic reprogramming to accommodate high nutrient and energetic requirements for rapid proliferation.1–3 Of note, the energy metabolic plasticity and adaptability allow cancer cells to flexibly dynamically switch between aerobic glycolysis and mitochondrial respiration in response to changing microenvironments to maintain the supply of adenosine triphosphate (ATP) and nutrients, which contributes to resistance against various types of oncotherapies.4 Additionally, such hyperactive energy metabolism in tumors often leads to the accumulation of reactive oxygen species (ROS).5 Increased ROS, particularly hydrogen peroxide (H2O2), can act as second messengers and rapidly regulate cellular metabolic pathways by oxidizing cysteine residues present in various metabolic enzymes; then, the rewired tumor metabolism in turn managed the redox homeostasis by upregulating antioxidants such as glutathione (GSH) to avoid cell death induced by excessive oxidative stress.6–8 This interdependent relationship between metabolism and redox signaling establishes a crucial metabolism-redox circuit, endowing tumors with survival advantages and facilitating oncotherapy resistance.9 Therefore, the combination of energy-deprivation and ROS amplifiers is expected to break the adaptability of tumor cells to single starvation therapy and oxidative stress, holding great promise for treating cancer and overcoming oncotherapy resistance. However, it is a significant challenge to meet these requirements solely with a single drug. Nanomedicine has emerged as a promising strategy to address this obstacle.10,11
At present, nanomaterials with the forementioned functions mainly rely on glucose oxidase, which can consume glucose and oxygen (O2) to produce H2O2, as well as the incorporation of small molecule inhibitors of glycolysis or mitochondrial respiration with ROS amplifiers.12,13 However, tumor hypoxia, metabolic adaptability and complex synthetic processes impede the utilization of these approaches. Zinc ions, existing as a divalent cation (Zn2+), are the second most abundant essential trace element and act as a cofactor for various enzymes in the body.14 Several studies have confirmed that high levels of Zn2+ inhibit glycolysis and mitochondrial respiration, by inhibiting the activity of related metabolic enzymes, such as glyceraldehyde 3-phosphate dehydrogenase, phosphofructokinase, lactate dehydrogenase (LDH), and α-ketoglutarate dehydrogenase (α-KGDHC), as well as interfering with the mitochondrial electron transfer chain (ETC).15–18 Interestingly, Zn2+ can also augment the production of ROS, such as superoxide anion (˙O2−) and H2O2 through the single electron reduction of O2 from ETC and the single/double electron reduction of O2 induced by α-KGDHC and nicotinamide adenine dinucleotide phosphate oxidase.18–22 Therefore, increased Zn2+ is a reliable candidate for targeting the energy metabolism-redox circuit. It is noteworthy that increased Zn2+ will upregulate metallothioneins (MTs) enriched with cysteine, which can maintain intracellular Zn2+ balance and reduce oxidative stress.23 Consequently, the upregulation of the MT levels will weaken the inhibitory effect of Zn2+ on metabolism and attenuate oxidative stress injury. Fortunately, some researches have shown that high levels of ROS can oxidize MTs to destroy Zn2+-cysteine binding, leading to the release of Zn2+ and disruption of Zn2+ homeostasis.24–26 Consequently, disrupting the intracellular equilibrium between Zn2+ and ROS will ignite an uncontrollable self-amplifying cycle, reversing the adaptability of tumor cells to external stimuli. Therefore, utilizing the unique relationship between Zn2+ and ROS amplifiers can enhance their capabilities to regulate metabolism-redox circuits, improve the tumor killing effect and reverse therapeutic resistance.
Mild photothermal therapy (PTT), an emerging non-invasive and spatiotemporally controllable therapeutic modality, has gained substantial admiration in cancer treatments. As it can kill tumors at comparatively low temperatures (below 45 °C), while avoiding damage to surrounding tissues induced by thermal diffusion, it greatly improves the therapeutic tolerance. However, once subjected to hyperthermia, heat shock proteins (HSPs), well-known molecular chaperones, will be upregulated to repair the denatured and aggregated proteins induced by heat, thereby enhancing thermotolerance and reducing therapeutic effects. However, the biosynthesis and regulatory activity of HSPs require ATP supply, thus nanomaterials targeting the energy metabolism-redox circuit are promising to effectively reverse the resistance to mild PTT by reducing ATP content.
Herein, we successfully developed zinc peroxide nanoparticles (ZnO2 NPs) as highly effective metabolism-redox circuit regulators and ROS enhancers by a simple method. Subsequently, a polydopamine (PDA) shell was encapsulated on the surface (ZnO2@PDA NPs) to improve the stability and biocompatibility, endowing them with photothermal properties. The nanocomplex was designed to deliver large amounts of exogenous Zn2+ and H2O2 into cells through acid-responsive degradation. The presence of exogenous Zn2+ could promote the production of endogenous H2O2 through the multi-mechanism previously described. The combined effect of exogenous and newly generated endogenous H2O2 could promote endogenous Zn2+ release from MTs and disrupt the regulation effects of MTs on Zn2+ homeostasis. Subsequently, the released Zn2+ in turn promoted H2O2 production, thus forming a self-amplifying loop between Zn2+ and H2O2. The significantly increased Zn2+ and H2O2 levels could obstruct ATP production from glycolysis and mitochondrial respiration by inhibiting the activity of multiple metabolism enzymes and causing mitochondrial dysfunction, thus breaking the adaptability of single-pathway starvation therapy. Meanwhile, ATP depletion could impede ATP-dependent GSH and HSP biosynthesis, reversing the resistance to endogenous/exogenous H2O2-induced oxidative stress and PDA-mediated mild PTT. This nanocomplex that integrated a smart self-amplifying disruptor of the energy metabolism-redox circuit with mild PTT was confirmed to be a superior therapeutic agent for considerably enhancing antitumor effects. Therefore, this work provided a promising strategy for the targeted regulation of the metabolism-redox circuit in tumors to maximize the efficacy of mild PTT, which offers a valuable reference for the development of novel therapeutic approaches to overcome therapeutic resistance (Scheme 1).
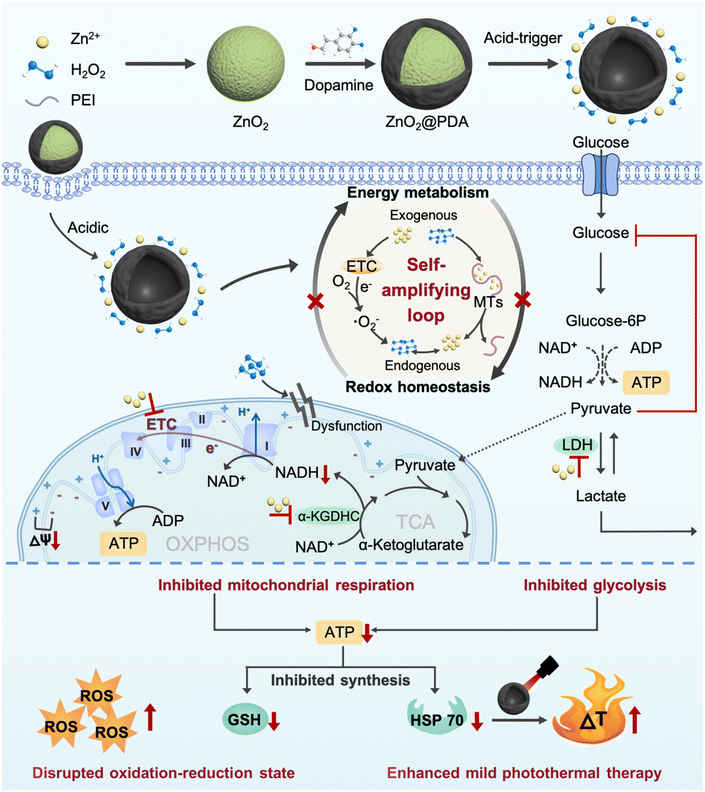 |
| Scheme 1 Schematic illustration of the preparation and therapeutic mechanism of ZnO2@PDA NPs. | |
Results and discussion
Synthesis and characterization of ZnO2@PDA NPs
ZnO2@PDA NPs were synthesized by reacting zinc acetate with H2O2 in an alkaline aqueous solution containing polyethyleneimine (PEI) and then coated with a PDA protective layer via in situ self-polymerization of dopamine in a weakly alkaline environment (Fig. 1a). As shown in the transmission electron microscope (TEM) image, the as-synthesized ZnO2 NPs exhibited spherical structures with an average diameter of 98 nm (Fig. 1b) and ZnO2@PDA NPs showed a typical core–shell structure, and the shell thickness was about 32 nm (Fig. 1b). The high angle annular dark field image-scanning transmission electron microscope (HAADF-STEM) image and energy dispersive X-ray spectroscopy (EDX) elemental mapping images confirmed the presence of Zn, O, C, and N elements. Notably, Zn and O elements were predominantly located at the core, while C and N elements were uniformly distributed throughout the NPs, indicating the successful surface coating of PDA (Fig. 1c). Meanwhile, after coating PDA, the average hydrodynamic diameter increased from 190.8 nm to 231.4 nm, and the mean zeta potential transformed from 40.5 mV to −22.2 mV, providing further confirmation of the successful synthesis of ZnO2@PDA NPs (Fig. S1, ESI†). According to the thermo-gravimetric analysis, the amount of PDA in ZnO2@PDA was around 13.8 wt% (Fig. S2, ESI†). X-ray diffraction analysis (XRD) verified that the patterns of the synthesized ZnO2 and ZnO2@PDA were consistent with those of the standard ZnO2 card (PDF #13-00311), indicating that PDA encapsulation had no obvious influence on the structure phase of ZnO2 (Fig. 1d). Additionally, the chemical bonds and elemental compositions were determined by X-ray photoelectron spectroscopy (XPS) (Fig. S3 (ESI†) and Fig. 1e and f). Compared with the ZnO2 sample, the attenuation of the XPS signals and the augmentation in N element content in the ZnO2@PDA sample could be ascribed to the PDA coating. The high-resolution spectrum of Zn 2p exhibited two characteristic peaks at 1045.0 eV and 1022.2 eV, which were assigned to the Zn 2p1/2 and Zn 2p3/2 orbits of Zn2+, respectively (Fig. 1e). The O1s peak at 533.1 eV could be attributed to O22−, indicating that PDA encapsulation did not obstruct peroxides (Fig. 1f).27 These were well consistent with the results of TEM, element mapping, and XRD. Besides, because of the presence of peroxo groups that could reduce pink MnO4− to colorless Mn2+, ZnO2@PDA NPs effectively made the acidic potassium permanganate (KMnO4) solutions fade, and the absorption peaks of MnO4− in the UV–vis spectrum disappeared (Fig. S4, ESI†).
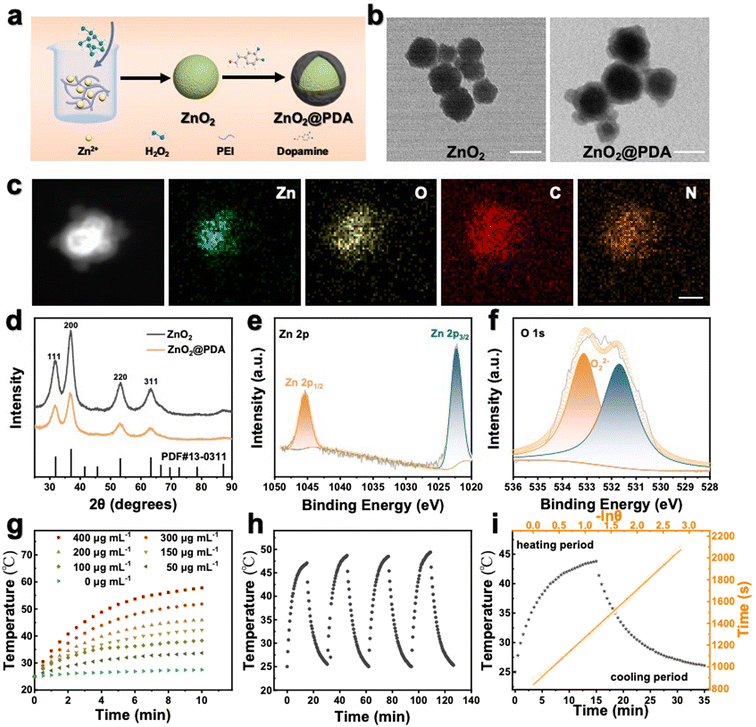 |
| Fig. 1 (a) Simple illustration of the synthesis process of ZnO2 NPs and ZnO2@PDA NPs. (b) Transmission electron microscopy (TEM) images of ZnO2 NPs and ZnO2@PDA NPs. Scale bars, 100 nm. (c) High angle annular dark field image-scanning transmission electron microscope (HAADF-STEM) image and energy dispersive X-ray spectroscopy (EDX) elemental mapping images showing ZnO2@PDA NPs with a typical core–shell structure. Scale bar, 100 nm. (d) The X-ray diffraction analysis (XRD) of ZnO2 and ZnO2@PDA NPs. (e) and (f) The X-ray photoelectron spectroscopy spectra of ZnO2@PDA NPs: Zn 2p regions (e) and O 1s regions (f). (g) Photothermal heating curves of ZnO2@PDA NPs at different concentrations upon 808 nm laser exposure (1.0 W cm−2). (h) Photothermal stability test of ZnO2@PDA NPs (200 μg mL−1) upon four repeated irradiation (1.0 W cm−2) on–off cycles. (i) Calculation of the photothermal conversion efficiency of ZnO2@PDA NPs according to the heating/cooling curves. | |
The pH-responsive decomposition of ZnO2@PDA NPs in vitro
The PDA coating served as a protective layer to hinder particle aggregation. Therefore, ZnO2@PDA NPs exhibited better stability under aqueous and simulated humoral conditions than the uncoated ones (Fig. S5, ESI†), suggesting their potential for applications under physiological conditions. Next, we explored the acid-responsive degradation behavior of ZnO2@PDA NPs. Their morphological changes under neutral (pH 7.4) and acidic (pH 5.5) conditions were meticulously characterized by TEM (Fig. S6a and b, ESI†). As expected, the ZnO2@PDA NPs maintained a stable morphology and size under neutral conditions. However, in the acidic environment, the original core–shell structure dissipated entirely, due to the sheet spacings and gaps in the PDA shell, which allow the phosphate buffered saline (PBS) buffer to directly contact the ZnO2 nano core, degrade the core, and leave the acid-resistant PDA shell behind, indicating a pH-dependent degradation process of ZnO2.28,29 Studies have shown that an acidic environment prompted the degradation of ZnO2 NPs into Zn2+ and H2O2, because of the available proton concentration around ZnO2 in acidic conditions, while the proton can only be obtained via a slow hydrolysis process of water under neutral conditions.27 To further investigate the degradation process, the liberation patterns of Zn2+ and H2O2 in different pH buffers were evaluated. Zn2+ concentration was detected by ICP-MS. The H2O2 concentration was measured by the cerium sulfate method, as Ce4+ can be reduced to colorless Ce3+ by H2O2 in acid solution.27 As shown in Fig. S6c and d (ESI†), ZnO2@PDA NPs released Zn2+ and H2O2 much more rapidly and extensively in acidic buffers than in neutral buffers. All these results implied that the ZnO2@PDA could hold a stable “off” state during blood circulation, while it converted to the “on” state in the acidic tumor microenvironment by releasing Zn2+ and H2O2, unveiling its potential for precision tumor therapy.
Photothermal performance of ZnO2@PDA NPs in vitro
Besides serving as a protective layer, PDA could also be used as an efficient photothermal reagent attributed to its melanin-like molecular structure.30 Therefore, we further investigated the photothermal characteristics of the ZnO2@PDA NPs. Following the incorporation of the PDA shell, the ZnO2@PDA NPs exhibited an increased absorbance in the near-infrared region, implying enhanced photothermal performance (Fig. S7a, ESI†). Next, the photothermal effect of the ZnO2@PDA NPs was investigated by monitoring the temperature of the ZnO2@PDA suspensions at different concentrations upon 808 nm laser irradiation. As shown in Fig. 1g, the ZnO2@PDA NPs showed a time- and concentration-dependent photothermal activity. More specifically, the temperature of the ZnO2@PDA suspension at the concentration of 400 μg mL−1 could escalate to 57.8 °C within 10 min, while there was no apparent change for the temperature of water. The thermographic images visually displayed the real-time temperature changes of the ZnO2@PDA suspensions upon laser irradiation (Fig. S7b, ESI†). In addition, a laser power density-dependent temperature raising profile of ZnO2@PDA NPs has also been demonstrated (Fig. S7c and d, ESI†), indicating that the photothermal temperature of ZnO2@PDA could be conveniently regulated by managing the power density of an external 808 nm laser source. Moreover, the degradation of the ZnO2 core did not affect the photothermal properties of the ZnO2@PDA NPs, indicating their stable photothermal conversion capacity in an acidic tumor microenvironment, which provides support for stable photothermal therapy in vivo (Fig. S8, ESI†). The photothermal performance of ZnO2@PDA was unaffected over four cycles of laser on/off irradiation, indicating remarkable photothermal stability (Fig. 1h). The photothermal effect (27.8%) of ZnO2@PDA NPs highlighted their great potential as effective agents for PTT (Fig. 1i). Considering the irreversible heating damage to surrounding healthy tissues and the undesirable inflammation induced by hyperthermia, achieving effective therapeutic effects at relatively mild temperatures (≤45 °C) is greatly desirable for the application of PTT. Therefore, we chose suitable conditions, including the ZnO2@PDA concentration (150 μg mL−1) and laser irradiation (1.0 W cm−2), for subsequent experiments.
Intracellular self-amplifying loop of Zn2+ and H2O2
To investigate whether ZnO2@PDA NPs could be uptaken by HeLa cells, we labeled the NPs with fluorescein isothiocyanate (FITC) and used confocal laser scanning microscopy (CLSM) to visually detect their uptake process. With the extension of the incubation time, the green fluorescence representing FITC-labeled ZnO2@PDA NPs gradually increased in the cells, which proved that the NPs can be effectively uptaken by HeLa cells (Fig. S9, ESI†). Encouraged by the controllable release behavior of ZnO2@PDA NPs in acidic conditions, their capability to induce intracellular accumulation of Zn2+ and H2O2 was further evaluated. Zinquin ethyl ester (Zn2+ probe) and 2′,7′-dichlorodihydrofluorescein diacetate (DCFH-DA, ROS probe) were used to evaluate the levels of Zn2+ and H2O2 in HeLa cells via CLSM, as zinquin ethyl ester can combine with Zn2+ to produce blue fluorescence, and DCFH-DA can be oxidized by H2O2 to 2′,7′-dichlorofluorescein and produce green fluorescence. Compared to the control group and PDA group, the fluorescence intensities of both zinquin ethyl ester (blue) and DCFH-DA (green) in the ZnO2@PDA group were significantly enhanced, indicating an obvious increase of intracellular Zn2+ and H2O2 content (Fig. 2a). More interestingly, the addition of N,N,N,N-tetrakis(2-pyridylmethyl)-ethylenediamine (TPEN), a membrane-permeable zinc ion specific-chelating agent reduced the intracellular H2O2 output, as evidenced by the weaker green fluorescence intensity of the ZnO2@PDA + TPEN group. Similarly, when adding catalase (CAT) to drive H2O2 decomposition, the intracellular free Zn2+ level was decreased (Fig. 2a). This phenomenon revealed a significant correlation between Zn2+ overload and H2O2 accumulation in cancer cells, which could be regarded as a “self-amplifying loop”. More specifically, the elevated Zn2+ can inhibit the forward electron transfer in ETC at the probable sites of complexes I and III, causing electronic leakage to O2 for forming ˙O2− and dissipating mitochondrial membrane potential (ΔΨm).17,22,31 Then the generated ˙O2− could be converted into H2O2 under the catalysis of endogenous superoxide anion dismutase. In addition, elevated Zn2+ can strongly induce the oxidase activity of dihydrolipoyl dehydrogenase in the α-KGDHC complex, which functions in transferring electrons and H+ from nicotinamide adenine dinucleotide (NADH) to O2, leading to the production of H2O2 and ˙O2−.18–20 The levels of intracellular ˙O2− were measured using a dihydroethidium (DHE) reagent. The results showed that compared to the control and PDA groups, ZnO2@PDA NPs significantly increased the intracellular ˙O2− levels (Fig. 2a). Furthermore, the addition of TPEN reduced the generation of intracellular ˙O2− induced by ZnO2@PDA NPs. These findings are consistent with previous studies and further support the idea that elevated Zn2+ levels led to the production of endogenous ˙O2− and H2O2. Conversely, elevated H2O2 can reduce the release of endogenous Zn2+, likely through an interaction between H2O2 and MTs. MTs are ubiquitous proteins that play a crucial role in regulating Zn2+ homeostasis and preventing oxidative stress.24,32,33 Upon exposure to abundant H2O2, the rich zinc-binding cysteine residues in MTs could be oxidized to form disulfide bonds, thereby expelling free Zn2+ to break Zn2+ homeostasis.24,26 In this study, we utilized an ultraviolet-visible spectrometer to observe the release behavior of Zn2+ from MTs in the presence of zinc indicator (ZI). The ZI can chelate free Zn2+ and form an absorption peak at 618 nm. Our results indicated that there was no significant increase in absorbance at 618 nm without H2O2, suggesting that Zn2+ release from MTs was minimal without H2O2 (Fig. S10a, ESI†). However, upon the addition of H2O2, the absorbance at 618 nm increased, indicating that H2O2 can induce MTs to release Zn2+ in a time-dependent manner (Fig. 2b and Fig. S10b–d, ESI†), which is consistent with previous findings.34 Furthermore, oxidative stress can impair the functionality of organelles such as the endoplasmic reticulum and mitochondria, leading to a reduction in their ability to regulate Zn2+ homeostasis and ultimately resulting in an increase in free Zn2+ content.35,36 Therefore, the fluorescence signals of Zn2+ and ROS detected in HeLa cells treated with ZnO2@PDA NPs are a result of not only exogenous Zn2+ and H2O2 release, but also endogenous Zn2+ release and ROS production.
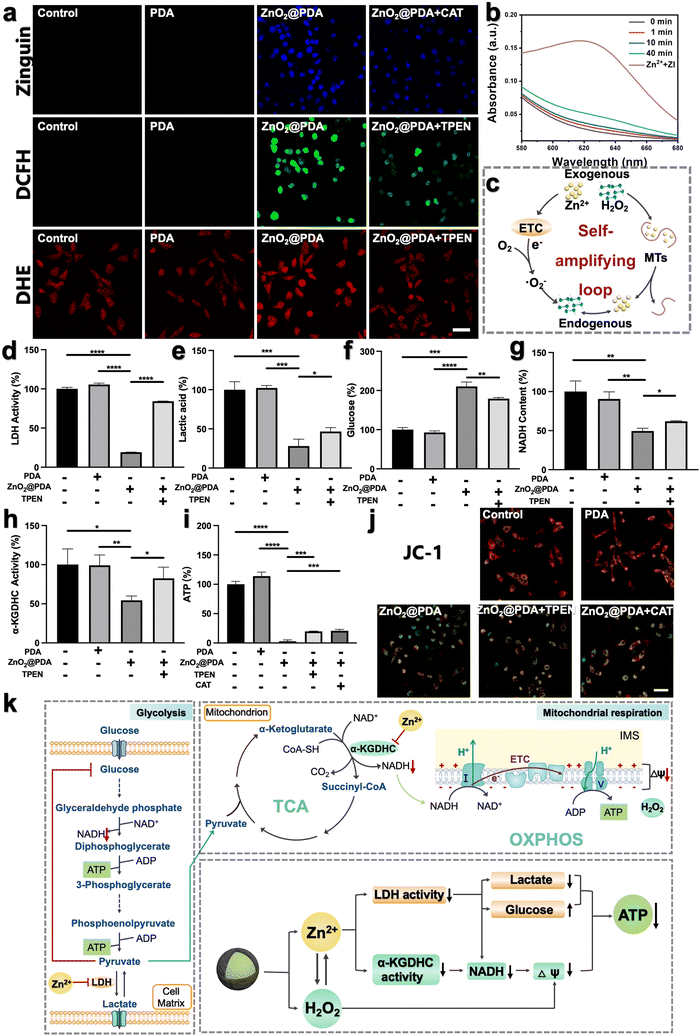 |
| Fig. 2 (a) The intracellular distribution of intracellular free Zn2+ by Zinquin ethyl ester probe (blue color), H2O2 by DCFH-DA probe (green color), and ˙O2− by DHE probe (red color) in HeLa cells subjected to diverse treatments through confocal laser scanning microscope (CLSM) imaging. Scale bar, 50 μm. (b) Absorption spectra showing the release of Zn2+ from MTs by ZI in the presence of H2O2. ZI, as a Zn2+ indicator, can chelate free Zn2+ and form an absorption peak at 618 nm. (c) Illustration of the mechanism of the intracellular self-amplifying loop of Zn2+ and H2O2. (d)–(i) Energy metabolism-related enzyme activity, glucose and some metabolite content: LDH activity (d), lactic acid content in the culture medium (e), glucose content in the culture medium (f), NADH (g), α-KGDHC activity (h), and ATP levels (i) in HeLa cells after different treatments. Data are represented as mean ± S.D. n = 3. *P < 0.05, ** P < 0.01, ***P < 0.001, ****P < 0.0001. (j) Mitochondrial membrane potential of HeLa cells after diverse treatments probed by JC-1. The change in color from red to green represents a decrease in mitochondrial membrane potential. Scale bar, 50 μm. (k) Illustration of the inhibitory mechanism of energy metabolism by ZnO2@PDA-induced Zn2+ and H2O2 overload. | |
In summary, ZnO2@PDA NPs could release exogenous Zn2+ and H2O2 in response to the acidic tumor microenvironment, which then unfolds an intracellular self-amplifying loop to boost endogenous Zn2+ and ROS (Fig. 2c). This dual amplification strategy is promising for disrupting zinc and redox homeostasis efficiently and durably, ultimately leading to Zn2+ and H2O2 overload in tumor cells.
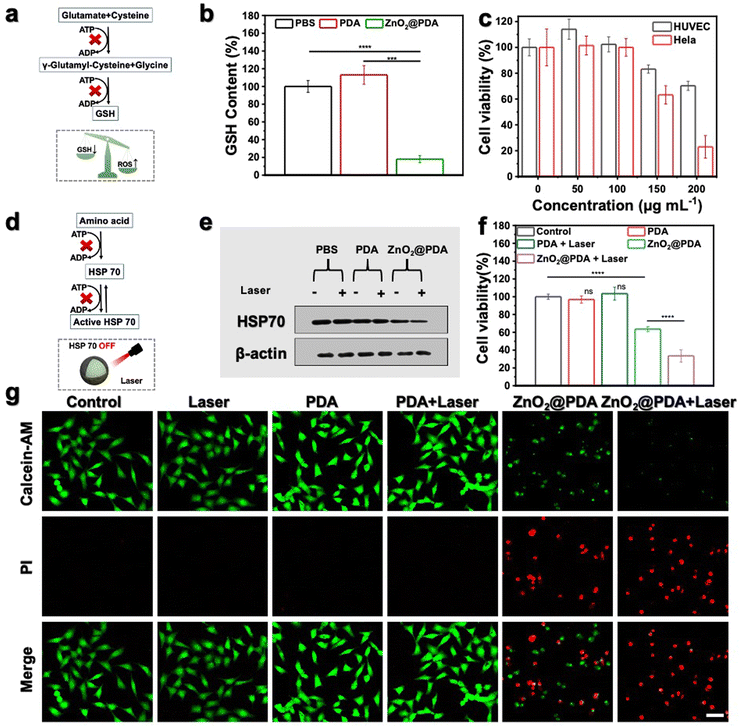 |
| Fig. 3 (a) Simple illustration of ATP depletion inhibiting the production of GSH. (b) GSH levels in HeLa cells subjected to diverse treatments for 24 h. ***P < 0.001, ****P < 0.0001. (c) Cell viability of HeLa (tumor cell) and HUVEC (normal cell) cells subjected to diverse concentrations of ZnO2@PDA NPs for 24 h. (d) Simple illustration of ATP depletion inhibiting the biosynthesis and functional activation of HSP 70. (e) Western blot analysis of HSP 70 expression in HeLa cells subjected to diverse treatments for 24 h. (f) Cell viability of HeLa cells subjected to diverse treatments for 24 h. (g) Calcein AM (Green color represents live cells) and PI (Red color represents dead cells) co-staining of HeLa cells subjected to diverse treatments for 24 h through CLSM imaging. Scale bar, 50 μm. | |
The dual-inhibitory effect of ZnO2@PDA on both glycolysis and mitochondrial respiration
The imbalance of both Zn2+ and ROS levels would affect multifarious physiological functions. Therefore, we further investigated the regulatory potency of ZnO2@PDA NPs in tumor cells, particularly pertaining to energy metabolism. It has been reported that intracellular Zn2+ overload could disrupt energy metabolism through interfering with multiple pathways, including glycolysis and mitochondrial respiration, which includes the tricarboxylic acid cycle (TCA) and oxidative phosphorylation (OXPHOS).15–19,37,38 In view of the fact that most tumor cells are highly dependent on aerobic glycolysis to meet the energy requirements; we firstly investigated the regulatory effect of ZnO2@PDA NPs on the glycolysis process. LDH, a key glycolytic enzyme, catalyzes the interconversion between pyruvate and lactate. Intriguingly, elevated Zn2+ can effectively suppress LDH activity to obstruct pyruvate-to-lactic acid conversion, resulting in pyruvate accumulation with negative feedback on glycolysis, and finally blocking ATP and NADH generation (Fig. 2k).39,40 Firstly, we quantified the intracellular LDH activity, and ATP and NADH content, as well as the lactate and glucose content in the culture medium in each group. As expected, the LDH activity in HeLa cells treated with ZnO2@PDA significantly decreased compared to the control and PDA groups. This decrease resulted in lower lactate levels, slower glycolysis, and reduced glucose utilization, and ultimately hindered the production of ATP and NADH (Fig. 2d–g and i). Meanwhile, utilizing TPEN to chelate partial Zn2+ successfully relieved the above changes caused by ZnO2@PDA NPs (Fig. 2d–g and i). All these results suggested that ZnO2@PDA -induced Zn2+ overload in tumor cells inhibited the glycolytic pathway.
Notably, inhibited glycolysis in tumor cells may lead to an up-regulation of mitochondrial respiration in order to maintain ATP supply through metabolic plasticity.41 It is worth considering whether the accumulation of pyruvate induced by Zn2+ enters the TCA and promotes mitochondrial respiration, thereby counteracting the inhibition of energy metabolism. Fortunately, it has been reported that elevated Zn2+ can simultaneously inhibit both glycolysis and mitochondrial respiration, showing great potential in combating the metabolic adaptability of tumors.17,42 Therefore, we conducted further investigations on the inhibition of ZnO2@PDA NPs on mitochondrial respiration. NADH, which is a critical substance for OXPHOS and originates from both glycolysis and TCA, acts as a donor of hydrogen (H+) and electrons. The provided H+ flows into the mitochondrial membrane gap for forming ΔΨm, and the internal flow of H+ at the complex V site drives ATP generation (Fig. 2k). As shown in Fig. 2g, the NADH content in ZnO2@PDA-treated HeLa cells decreased compared to the control and PDA groups. This decrease can be attributed not only to the glycolysis blockade induced by zinc overload, but also to the interference effect of Zn2+ accumulation on the TCA cycle by inhibiting α-KGDHC activity.18 Elevated Zn2+ can obstruct ketoglutarate decarboxylation and the transformation from NAD+ to NADH by inhibiting α-KGDHC activity, which will interrupt mitochondrial energy metabolism (Fig. 2k).18–20 Therefore, we quantified the α-KGDHC activity in HeLa cells with different treatments. As expected, the α-KGDHC activity decreased in HeLa cells treated with ZnO2@PDA compared to that of the control and PDA groups (Fig. 2h). Moreover, dysregulated Zn2+ could directly affect the ETC, hindering electron transfer and H+ pumping, resulting in a decrease in ΔΨm and blocking ATP generation.42 Consequently, the decrease in intracellular NADH and the obstruction of the ETC synergistically inhibit the OXPHOS pathway, impeding ATP production.
Furthermore, as confirmed earlier, the accumulation of exogenous/endogenous ROS induced by ZnO2@PDA NPs can further induce mitochondrial dysfunction by causing oxidative damage to mitochondrial enzymes, lipids, and nucleic acids, which impairs ATP production (Fig. 2i).43 To assess the health of the mitochondria, we used JC-1, a voltage-sensitive probe exclusively relevant to mitochondria. CLSM results showed that untreated or PDA-treated HeLa cells exhibited strong red fluorescence of JC-1 aggregates indicating healthy mitochondria with sufficient ΔΨm, while the ZnO2@PDA NPs and ZnO2@PDA NPs + Laser groups exhibited increased green fluorescence indicating mitochondria dysfunction with reduced ΔΨm (Fig. 2j). Additionally, the addition of TPEN or CAT could alleviate mitochondrial membrane depolarization caused by ZnO2@PDA, confirming that the mitochondrial dysfunction induced by the ZnO2@PDA NPs is a result of both zinc overload and oxidative stress (Fig. 2i and j).
In summary, ZnO2@PDA NPs exhibited a synergistic inhibitory effect on the production of ATP from glycolysis and mitochondrial respiration, which was achieved through a complex interplay of multiple pathways (Fig. 2k).
ATP depletion enhanced mild PTT and oxidative stress by suppressing GSH and HSP 70
It is widely recognized that cancer cells typically exhibit a high redox state with the ROS levels approaching the toxicity threshold, rendering them more susceptible to ROS-mediated therapy. However, cancer cells may counteract excessive ROS by upregulating the production of reducing agents (such as GSH). As shown in Fig. 3a, the synthesis of GSH involves two ATP-dependent enzymatic reactions, including formation of γ-glutamyl-cysteine from glutamate and cysteine, and formation of GSH from glycine binding to γ-glutamyl-cysteine.44,45 Therefore, lowering GSH levels by inhibiting ATP production could augment the effectiveness of oxidative stress (Fig. 3a). Given the outstanding ATP-depleting and ROS-accumulating capabilities, ZnO2@PDA NPs are expected to effectively break the redox balance in tumors. To confirm this, the GSH consumption-capability of ZnO2@PDA was evaluated at the cellular level. Consistent with expectations, HeLa cells exposed to ZnO2@PDA exhibited significantly reduced intracellular GSH content compared to those in both the control and PDA groups, indicating their significant inhibitory effect on the antioxidant defenses of tumor cells (Fig. 3b). As a result, the synchronous energy metabolism inhibition and oxidative stress amplification induced by ZnO2@PDA NPs effectively disrupted the metabolism-redox circuit of tumor cells, facilitating tumor cells to approach death. The outcomes from the cell counting Kit-8 (CCK-8) cytotoxicity assay showed that ZnO2@PDA could efficiently decrease tumor cell viability in a concentration-dependent manner, while normal cells could tolerate the adverse effects of ZnO2@PDA at the same concentration (Fig. 3c).
ZnO2@PDA-mediated disruption of the tumor metabolism-redox circuit also exhibits great potential in reversing tumor resistance to PTT. It is widely acknowledged that the overexpression of HSPs triggered by high-temperature stimulation hinders the effectiveness of PTT. The biosynthesis and functional activation of HSPs relies on ATP support (Fig. 3d).46 Hence, we conducted a western blotting analysis to investigate whether the ZnO2@PDA-induced energy metabolic blockade could inhibit the biosynthesis of HSPs. The results, as shown in Fig. 3e and Fig. S11 (ESI†), demonstrate that the expression of HSP 70, a typical HSP, in HeLa cells treated with ZnO2@PDA NPs was reduced. Moreover, when NIR laser irradiation was applied to the ZnO2@PDA-treated cells for temperature elevation, the intracellular HSP 70 remained at a lower level. This finding underscores the potential application of ZnO2@PDA in mild PTT. The CCK-8 assay results and live/dead cell staining experiments further confirmed that the combination of ZnO2@PDA treatment and 808 nm laser irradiation resulted in significantly higher cytotoxicity against HeLa cells compared to the ZnO2@PDA group alone (Fig. 3f–g and Fig. S12, ESI†). Conversely, HeLa cells treated with an equivalent dose of PDA did not exhibit any influence on cellular vitality, even upon laser irradiation (Fig. 3f–g and Fig. S12, ESI†). Clearly, the inhibitory effects of ZnO2@PDA on ATP generation amplified the effect of PDA-mediated mild PTT.
Overall, ZnO2@PDA NPs demonstrated high efficacy in reducing intracellular ATP levels by modulating the metabolism-redox circuit, leading to a decrease in GSH biosynthesis and HSP 70 expression. Consequently, this resulted in a significant antitumor effect through a synergistic approach involving effective starvation therapy, increased oxidative stress, and enhanced mild PTT. These findings provide a solid basis for future in vivo studies.
Evaluation of antitumor efficacy on subcutaneous tumor models
The in vivo antitumor efficacy of ZnO2@PDA NPs was investigated in U14 subcutaneous tumor models, following the treatment timeline (Fig. 4a). The heating capability at tumor sites during laser exposure in the ZnO2@PDA NPs + laser group was assessed, and the 12th hour after intravenous injection was selected as the optimal photothermal treatment time, which showed superior photothermal properties (Fig. 4b and Fig. S13, ESI†). The tumor site was then exposed to laser irradiation for 5 minutes to control the temperature close to 43 °C for subsequent experiments. After three intravenous injections, both the ZnO2@PDA NPs group and the ZnO2@PDA NPs + laser group demonstrated excellent tumor suppression effects compared to the other four groups (Fig. 4c–e). The ZnO2@PDA NP group showed a tumor growth inhibition rate of 55%, while the ZnO2@PDA NPs + laser group demonstrated an impressive tumor growth inhibition rate of 92%, which was attributed to the combination of multiple synergistic effects (Fig. S14, ESI†). Based on changes in body weight, hematoxylin eosin (H&E) (staining of major organs, and blood physiological analysis, it has been demonstrated that the ZnO2@PDA NPs have excellent biocompatibility (Fig. 4f and Fig. S15–17, ESI†). H&E staining images of tumor tissues showed large amounts of karyolysis, pyknosis, and karyorrhexis of tumor cells in the ZnO2@PDA NPs and ZnO2@PDA NPs + laser groups, representing cell necrosis and apoptosis (Fig. 4g). The terminal deoxynucleotidyl transferase-mediated deoxyuridine triphosphate nick end labeling (TUNEL) staining also confirmed a more elevated level of apoptosis cells in the ZnO2@PDA NPs and ZnO2@PDA NPs + laser groups (Fig. 4g). HSP 70 staining proved that regulating the energy metabolism in tumor tissue could efficiently inhibit HSP 70 production, thereby reducing the heat resistance of tumor cells to achieve PTT sensitization (Fig. 4g). The above experimental results demonstrated that the ZnO2@PDA NPs + laser groups had excellent antitumor efficacy without obvious toxic side effects.
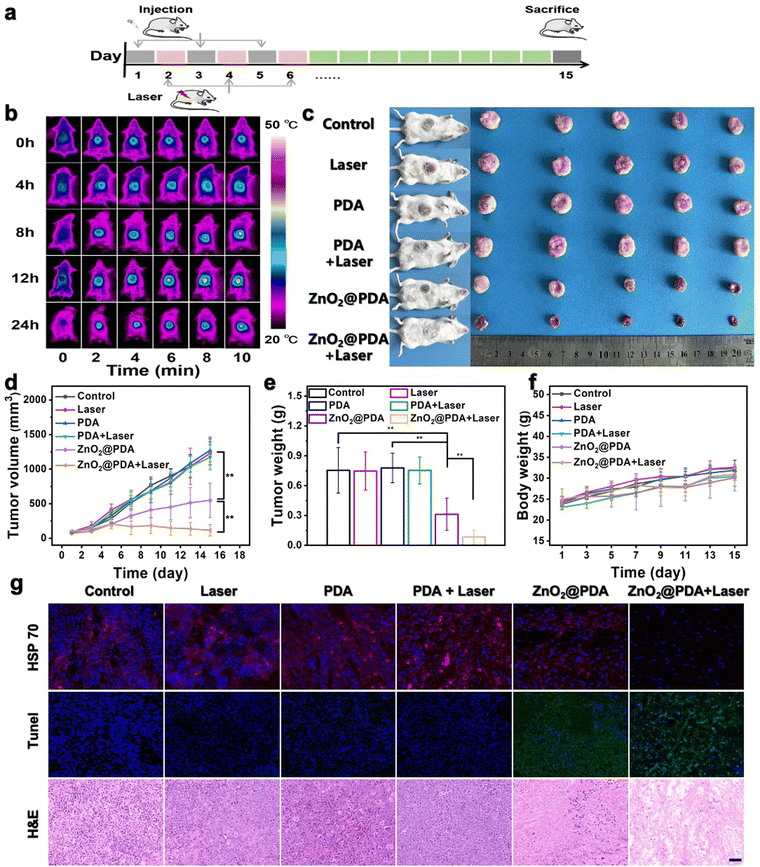 |
| Fig. 4 Therapeutic efficacy against U14 subcutaneous tumor-bearing mice by ZnO2@PDA NPs. (a) Simple illustration of the treatment process of tumor-bearing mice. (b) Thermographic images of tumor-bearing mice irradiated at tumor sites at various specific timepoints (0, 4, 8, 12, 24 h) after intravenous injection of ZnO2@PDA NPs. (c) Photographs of tumor-bearing mice and dissected tumors of different groups. (d)–(f) Tumor volumes, tumor weight, and body weight after different treatments. Data are represented as mean ± S.D. n = 5. ** P < 0.01. (g) HSP 70 (Red color represents HSP 70 and blue color represents nucleus), Tunel (Green color represents Tunel and blue color represents nucleus) and H&E staining of tumor tissues after different treatments. Scale bar, 50 μm. | |
Conclusions
In summary, we synthesized ZnO2@PDA NPs, which are metabolism-redox circuit regulators with photothermal properties. This study confirmed that exogenous Zn2+ and H2O2 released by ZnO2@PDA NPs in the acidic tumor microenvironment could unfold their intracellular self-amplifying loop, thereby augmenting the generation of endogenous H2O2 and Zn2+ and disrupting cell adaptation to stress. The increased levels of H2O2 and Zn2+ efficiently regulated the metabolism-redox circuit, and reduced glucose utilization by tumor cells, resulting in reduced ATP production from glycolysis and mitochondrial respiration, greatly reducing energy supply, which further impeded the biosynthesis of ATP-dependent GSH and HSP 70, reversing the resistance to H2O2-induced oxidative stress and PDA-mediated PTT. Ultimately, ZnO2@PDA NPs demonstrated optimal antitumor efficacy by synergistically employing multiple strategies including dual-starvation therapy, oxidative stress, and mild PTT. With the discovery of the intracellular self-amplifying loop of Zn2+ and ROS, as well as the in-depth understanding of the energy metabolism-redox circuit, and in turn utilizing them, the combination of Zn2+ and ROS can serve as an effective strategy for regulating the energy metabolism-redox circuit, opening up new possibilities for the development of novel antitumor approaches.
Conflicts of interest
There are no conflicts to declare.
Acknowledgements
This work was supported by the National Key R&D Program of China (Grant No. 2022YFE0113000), the National Science Fund for Distinguished Young Scholars (Grant No. 22025406), the National Natural Science Foundation of China (Grant No. 22204162) and the Hygiene Specific Subjects of Jilin Province (Grant No. 2022SCZ12, 2021SCZ37).
References
- N. N. Pavlova and C. B. Thompson, Cell Metab., 2016, 23, 27–47 CrossRef CAS PubMed.
- B. Faubert, A. Solmonson and R. J. DeBerardinis, Science, 2020, 368, eaaw5473 CrossRef CAS PubMed.
- O. Warburg, F. Wind and E. Negelein, J. Gen. Physiol., 1927, 8, 519–530 CrossRef CAS PubMed.
- S. M. Fendt, C. Frezza and A. Erez, Cancer Discovery, 2020, 10, 1797–1807 CrossRef CAS PubMed.
- C. Gorrini, I. S. Harris and T. W. Mak, Nat. Rev. Drug Discovery, 2013, 12, 931–947 CrossRef CAS PubMed.
- D. Trachootham, J. Alexandre and P. Huang, Nat. Rev. Drug Discovery, 2009, 8, 579–591 CrossRef CAS PubMed.
- K. M. Holmstrom and T. Finkel, Nat. Rev. Mol. Cell Biol., 2014, 15, 411–421 CrossRef CAS.
- J. Kim, J. Kim and J. S. Bae, Exp. Mol. Med., 2016, 48, e269 CrossRef CAS PubMed.
- K. Wang, J. Jiang, Y. Lei, S. Zhou, Y. Wei and C. Huang, Trends Biochem. Sci., 2019, 44, 401–414 CrossRef CAS PubMed.
- H. Yin, C. Fan, C. C. Wong, L. Mao, Y. Huang and G.-Q. Chen, Fundam. Res., 2023, 3, 479–480 CrossRef CAS.
- X. Song, H. Zhang, T. Yan, T. Hong and S. Zhang, Chin. J. Chem., 2020, 38, 1489–1496 CrossRef CAS.
- L. H. Fu, C. Qi, Y. R. Hu, J. Lin and P. Huang, Adv. Mater., 2019, 31, 1808325 CrossRef PubMed.
- Z. M. Fu, Y. F. Zhang, X. L. Chen, N. Wang, R. Y. Ma, X. Y. Luo, X. Pan, Y. Yang and W. Xue, Adv. Funct. Mater., 2023, 33, 2211869 CrossRef CAS.
- T. Kambe, T. Tsuji, A. Hashimoto and N. Itsumura, Physiol. Rev., 2015, 95, 749–784 CrossRef CAS PubMed.
- Z. Meng, X. Zhang, H. Tan and H. Lian, Chem. Eng. J., 2022, 435, 134781 CrossRef CAS.
- S. X. Wu, K. X. Zhang, Y. Liang, Y. B. Wei, J. Y. An, Y. F. Wang, J. L. Yang, H. L. Zhang, Z. Z. Zhang, J. J. Liu and J. J. Shi, Adv. Sci., 2022, 9, 2103534 CrossRef CAS PubMed.
- K. E. Dineley, T. V. Votyakova and I. J. Reynolds, J. Neurochem., 2003, 85, 563–570 CrossRef CAS PubMed.
- A. M. Brown, B. S. Kristal, M. S. Effron, A. I. Shestopalov, P. A. Ullucci, K. F. Sheu, J. P. Blass and A. J. Cooper, J. Biol. Chem., 2000, 275, 13441–13447 CrossRef CAS PubMed.
- I. G. Gazaryan, B. F. Krasnikov, G. A. Ashby, R. N. Thorneley, B. S. Kristal and A. M. Brown, J. Biol. Chem., 2002, 277, 10064–10072 CrossRef CAS.
- A. K. Chauhan, N. Mittra, G. Singh and C. Singh, J. Mol. Neurosci., 2022, 72, 1413–1427 CrossRef CAS.
- K. M. Noh and J. Y. Koh, J. Neurosci., 2000, 20, RC111 CrossRef CAS PubMed.
- K. E. Dineley, L. L. Richards, T. V. Votyakova and I. J. Reynolds, Mitochondrion, 2005, 5, 55–65 CrossRef CAS PubMed.
- A. K. Baltaci, K. Yuce and R. Mogulkoc, Biol. Trace Elem. Res., 2018, 183, 22–31 CrossRef CAS PubMed.
- N. C. Korkola and M. J. Stillman, J. Am. Chem. Soc., 2023, 145, 6383–6397 CrossRef CAS PubMed.
- D. E. Sutherland and M. J. Stillman, Metallomics, 2011, 3, 444–463 CrossRef CAS PubMed.
- C. Hubner and H. Haase, Redox Biol., 2021, 41, 101916 CrossRef PubMed.
- M. Zhang, R. Song, Y. Liu, Z. Yi, X. Meng, J. Zhang, Z. Tang, Z. Yao, Y. Liu, X. Liu and W. Bu, Chem, 2019, 5, 2171–2182 CAS.
- A. A. R. Watt, J. P. Bothma and P. Meredith, Soft Matter, 2009, 5, 3754–3760 RSC.
- N. T. Tran, D. P. Flanagan, J. A. Orlicki, J. L. Lenhart, K. L. Proctor and D. B. Knorr, Jr., Langmuir, 2018, 34, 1274–1286 CrossRef CAS PubMed.
- L. Tian, X. Li, H. Ji, Q. Yu, M. Yang, L. Guo, L. Huang and W. Gao, J. Nanobiotechnol., 2022, 20, 485 CrossRef CAS PubMed.
- S. L. Sensi, H. Z. Yin, S. G. Carriedo, S. S. Rao and J. H. Weiss, Proc. Natl. Acad. Sci. U. S. A., 1999, 96, 2414–2419 CrossRef CAS PubMed.
- C. Jacob, W. Maret and B. L. Vallee, Proc. Natl. Acad. Sci. U. S. A., 1998, 95, 3489–3494 CrossRef CAS PubMed.
- W. Maret, Proc. Natl. Acad. Sci. U. S. A., 1994, 91, 237–241 CrossRef CAS PubMed.
- S. Andrzejewski, E. Klimcakova, R. M. Johnson, S. Tabaries, M. G. Annis, S. McGuirk, J. J. Northey, V. Chenard, U. Sriram, D. J. Papadopoli, P. M. Siegel and J. St-Pierre, Cell Metab., 2017, 26(778–787), e775 Search PubMed.
- S. S. Sabharwal and P. T. Schumacker, Nat. Rev. Cancer, 2014, 14, 709–721 CrossRef CAS PubMed.
- K. G. Slepchenko, Q. Lu and Y. V. Li, Am. J. Physiol.: Cell Physiol., 2017, 313, C448–C459 CrossRef CAS PubMed.
- K. Murakami and M. Yoshino, Biometals, 2017, 30, 335–340 CrossRef CAS PubMed.
- H. Y. Liu, J. R. Gale, I. J. Reynolds, J. H. Weiss and E. Aizenman, Biomedicines, 2021, 9, 489 CrossRef CAS PubMed.
- X. Chen, L. Liu, S. Kang, J. R. Gnanaprakasam and R. Wang, Sci. Adv., 2023, 9, eadd9554 CrossRef CAS PubMed.
- C. Hayes, C. L. Donohoe, M. Davern and N. E. Donlon, Cancer Lett., 2021, 500, 75–86 CrossRef CAS PubMed.
- F. Dong, Q. Jiang, L. Li, T. Liu, S. Zuo, L. Gao, M. Fang, Y. Gao, B. Sun, C. Luo, Z. He and J. Sun, Nano Res., 2022, 15, 3422–3433 CrossRef CAS.
- E. Nam, J. Han, J. M. Suh, Y. Yi and M. H. Lim, Curr. Opin. Chem. Biol., 2018, 43, 8–14 CrossRef CAS PubMed.
- B. Perillo, M. Di Donato, A. Pezone, E. Di Zazzo, P. Giovannelli, G. Galasso, G. Castoria and A. Migliaccio, Exp. Mol. Med., 2020, 52, 192–203 CrossRef CAS PubMed.
- E. Hatem, N. El Banna and M. E. Huang, Antioxid. Redox Signaling, 2017, 27, 1217–1234 CrossRef CAS PubMed.
- S. C. Lu, Biochim. Biophys. Acta, 2013, 1830, 3143–3153 CrossRef CAS PubMed.
- M. I. Y. Elmallah, M. Cordonnier, V. Vautrot, G. Chanteloup, C. Garrido and J. Gobbo, Cancer Lett., 2020, 469, 134–141 CrossRef CAS PubMed.
|
This journal is © The Royal Society of Chemistry 2024 |
Click here to see how this site uses Cookies. View our privacy policy here.