DOI:
10.1039/D3NR04503J
(Paper)
Nanoscale, 2024,
16, 180-187
Electron-beam writing of a relaxor ferroelectric polymer for multiplexing information storage and encryption†
Received
7th September 2023
, Accepted 14th November 2023
First published on 14th November 2023
Abstract
To meet the strong demand for high-level encryption security, several efforts have been focused on developing new encryption techniques with high density and data security. Herein we employed a template-free electron beam lithography (EBL) technique to write various nanopatterns on poly(vinylidene fluoride-trifluoroethylene-chlorofluoroethylene) (P(VDF-TrFE-CTFE)) films and applied it to electron-beam/electric multiplexing memory. Furthermore, electron beams can arbitrarily tailor down the domain structure evolutions and dipole directions, as proved by a combination of AFM-IR and PFM. Finally, our devices could function concurrently as an electron-beam write-only-memory (EB-WOM) and FeRAM, where the information could be encoded with the metastable phase evolutions from the ferroelectric phase to the paraelectric phase and variable bi-level ferroelectric signals. Our systematic study provides an inspiring idea for the design of information encryption devices with high-security requirements in flexible electronic fields.
Introduction
With the arrival of the information explosion era, people are facing a huge risk of data leakage and falsification in the process of information transmission.1–3 Thus, memory devices with high density and high-level security have attracted tremendous attention in fields ranging from commercial and military activities to daily life. Traditional single-dimensional memory devices (e.g. resistive random access memory (R-RAM),4,5 magnetoresistive random-access memory (M-RAM),6,7 and ferroelectric random access memory (Fe-RAM)8–10) show two stable polarization states upon the application of alternating electric or magnetic fields,11 which readily suffer from data leakage. Herein, multiplexing FeRAM based on domain engineering is a promising avenue to attain high data capacity in a single storage cell and high information security with a specific read/write mode.12
Poly(vinylidene fluoride-trifluoroethylene-chlorofluoroethylene) (P(VDF-TrFE-CTFE)) is a chemically inert material, and its domain-controllable strategies are complicated and tedious for chemical modifications.13–16 Several efforts have been devoted to patterning ferroelectric polymer nanostructures using high-resolution and well-defined anodic aluminum oxide (AAO) molds17,18 or a hundred-nanometer-level silicon mold.19,20 Nanoconfined crystallization in the mold is conducive for the formation of one-type orientation domains, thus showing excellent data storage behavior for single-dimensional FeRAM.21,22 All the physical modification methods, such as nanoconfined crystallization,23 graphene-induced method24 and blending, are difficult to arbitrarily tailor the size and orientation of the ferroelectric domains in the micro/nano-processing technology.
In this study, electron beam lithography25 (EBL) was used as a specific domain-writing and template-free lithography technology that can arbitrarily pattern dose-controllable ferroelectric dots and grating arrays with a 200 nm resolution, and domain evolutions from the ferroelectric phase to the paraelectric phase that could be easily tailored by controlling the exposure doses. For the first-level information encryption, the information was encoded with the meta-stable phase evolutions from the ferroelectric phase to the paraelectric phase by the precise control of exposure doses, which served as a new technology of electron-beam write-only-memory (WOM) for digital watermarking.26,27 For the second encryption, the bi-polarization states were still built in the exposed P(VDF-TrFE-CTFE)[65
:
31
:
4] during the data writing/reading process; however, the meta-stable phases showed different phase contrasts, which can be used for second information storage and encryption. Therefore, the electron-beam/electric multiplexing memory based on domain-controllable strategies serves as a new type of high-security-level information encryption, except for optical storage material (OSM).28 Meanwhile, the effect of the exposure dose on domain evolutions from the relaxor ferroelectric phase into the paraelectric phase and dipole orientations were confirmed by atomic force microscopy-infrared spectroscopy29 (AFM-IR) and piezoelectric force microscopy (PFM).30 Briefly, our systematic study paves a new avenue to develop high-density ferroelectric patterns for high-security-level information storage and encryption, which has emerged as the next-generation memory devices.
Results and discussion
In the last few decades, ferroelectric polymers have shown two stable polarization states, which were developed as a single-dimensional FeRAM. Herein, we demonstrate that EBL can be configurable write ferroelectric nanopatterns, which can not only be developed as a new type of electron-beam write-only-memory (WOM) based on domain evolutions and dipole orientations for digital watermarking but also can be applied for variable bi-state FeRAM. This new type of electron-beam/electric multiplexing FeRAM can improve the information encryption security and capacitor, as shown in Fig. 1a. Typically, poly(vinylidene fluoride-trifluoroethylene-chlorotrifluoroethylene) P(VDF-TrFE-CTFE), which is a relaxor ferroelectric body with complex domain structure and dipole orientations, is selected as the best candidate material for developing multiplexing FeRAM. In Fig. S1,† a typical ferroelectric polymer shows the largest coercive electric field of 62 MV m−1 and a low dielectric constant of 11.5, indicating that the operation voltage of the P(VDF-TrFE)-based memory devices is high energy consumption. While P(VDF-TrFE-CTFE) [64
:
29
:
7] shows the lowest coercive electric field and the lowest remanent polarization of 0.67 μC cm−2. Such low remanent polarization can result in poor storage stability. Therefore, compared with the typical ferroelectric polymer P(VDF-TrFE) [70
:
30] and P(VDF-TrFE-CTFE) [64
:
29
:
7], P(VDF-TrFE-CTFE) [65
:
31
:
4] exhibits a higher dielectric constant of 49.5 at a frequency of 1000 Hz (Fig. 1b) and a lower coercive electric field of 30 MV m−1 (Fig. 1c), which is developed as a low-power consumption and fast-switching behavior of the PVDF-based ferroelectric memory.
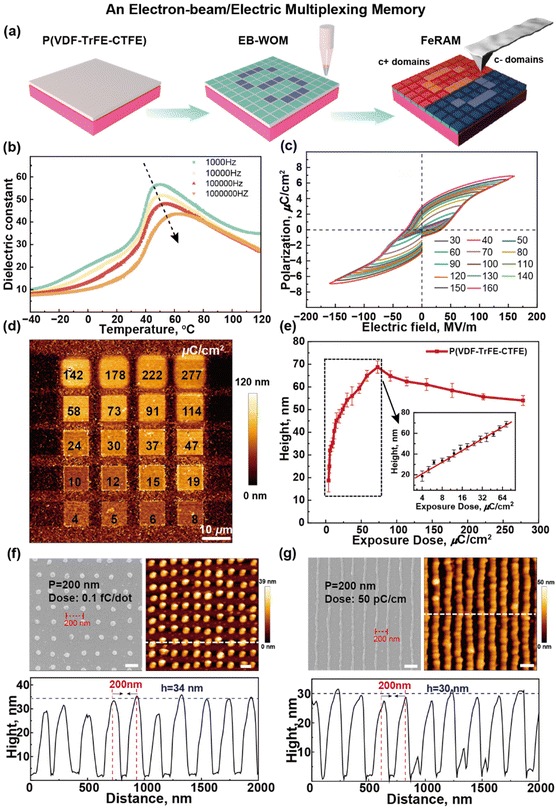 |
| Fig. 1 (a) Schematic diagram of an electron-beam/electric multiplexing memory. (b) Temperature-dependence of dielectric constant at frequencies of 1 kHz, 10 kHz, 100 kHz, and 1 MHz for pristine terpolymer P(VDF-TrFE-CTFE) films. (c) Bipolar polarization–electric (P–E) loops of P(VDF-TrFE-CTFE) at different electric fields. (d) AFM topography of the ferroelectric array irradiated at various exposure doses. The height value of the pattern vs. the exposure doses along the white-dashed line. (e) Height vs. exposure doses of irradiated P(VDF-TrFE-CTFE) patterns. At low exposure doses between 4 μC cm−2 to 73 μC cm−2, the exponential relationship between the exposure dose and the height of irradiated P(VDF-TrFE-CFE) in the inset. High-resolution capability demonstrated in the SEM images, AFM topography of (f) the dot array, and (g) the grating array with a period of 200 nm. The scale bar is 200 nm. The heights of the dot and grating arrays are 34 nm and 30 nm, respectively, along the white-dashed line. | |
To understand the processing behavior of P(VDF-TrFE-CTFE) when used as EBL resists, an array with 4 × 5 ferroelectric cells was written with a series of exposure doses from 4 to 278 μC cm−2, and then etched out by the butanone and DMF, as shown in Fig. S2.† During EB irradiation, the macromolecular radicals of P(VDF-TrFE-CTFE) were formed, and then the macromolecular radicals were finally turned into the cross-linking networks in the chain-termination reaction, as shown in Fig. S3.† Therefore, the solubility of P(VDF-TrFE-CTFE) can change after electron beam irradiation. The non-irradiated area was dissolved in DMF or butanone, and the irradiated zone was reserved onto the surface of the silicon. It is found that the butanone for each cell is the better developer than that of the DMF, as shown in Fig. S2,† possibly because the irradiated P(VDF-TrFE-CTFE) shows strong dissolvability in the butanone solution. Then, the thickness of the nanocells was measured using the atomic force microscope (AFM), as shown in Fig. 1d. The thickness increases at first and then decreases with the increasing exposure doses. Notably, the inset of Fig. 1f illustrates that there is an exponential relationship between the obtained thickness and the exposure range of 4–73 μC cm−2, which benefits the controllability of the following device fabrication. In order to determine the resolution capability of the P(VDF-TrFE-CTFE) resist, dense dot and grating arrays were written by EBL. As shown in Fig. S4,† the thickness of the films for EBL increased from 20 to 125 nm with the increase in the concentration of P(VDF-TrFE-CTFE) from 5 mg ml−1 to 25 mg ml−1. When the thickness is high (70 and 125 nm), regular dot arrays with different periods of 200 nm, 250 nm, and 300 nm could not be obtained, as shown in Fig. S5.† To avoid the collapse and binding, a thinner resist film could be beneficial for obtaining higher resolution. As shown in Fig. 1f and g, when the film thickness is scaled down to 20 nm, both the dot and grating array show a height of 34 nm and a period of 200 nm. It is developed as an integration density of up to 66.7 Gbit inch−2 for memory devices.
Crystal structure evolution
In the AFM-IR experiments, AFM-IR absorption spectra were obtained by keeping the AFM tip stationary at the testing position, as shown in Fig. 2a. Then, the crystal structure evolution of P(VDF-TrFE-CTFE) irradiated at different exposure doses from 5 to 58 μC cm−2 was swept using the laser in the wavelength range of 900–1900 cm−1, as shown in Fig. 2b. As was seen from the label, the bands at 1383 and 1425 cm−1 belong to non-nanopolar domains with TGTG’ conformation, whereas the bands at 1209, 1277, and 1431 cm−1 are ascribed to nanopolar domains with all-trans conformations. With the increase of the exposure dose from 5 to 58 μC cm−2, a large enhancement in the absorption value of the non-nanopolar domains at the peak of 1209 cm−1 was found in the irradiated P(VDF-TrFE-CTFE). The absorbance at 1277 cm−1 was remarkably decreased in turn, indicating that the electron beam irradiation can cause the partial nanopolar domains into non-polar domains. Furthermore, the corresponding IR absorption images at 1209 cm−1 and 1277 cm−1 were visualized and mapped in Fig. 2c–e, which are attributed to the distribution of nanopolar domains and non-polar domains in the irradiated area, respectively. At the low exposure dose of 10 μm cm−2, low value of IR amplitude at 1209 cm−1 (Fig. 2f) is 1.25 mV and high value of IR amplitude at 1277 cm−1 is 2.5 mV (Fig. 2g). At high exposure dose of 30 μC cm−2, the amplitude value at 1209 cm−1 increased to 2.32 mV, and the amplitude value at 1277 cm−1 increased to 1.36 mV, indicating that the nanopolar domains were transferred into non-polar domains under electron beam irradiation. In addition, P(VDF-TrFE-CTFE) exhibits a semi-crystalline crystal. Under electron beam irradiation, the larger size of ferroelectric crystals was tailored, and converted into a smaller size of the crystals and more amorphous phase in the irradiated P(VDF-TrFE-CTFE), as shown in Fig. S6.† Briefly, the domain structure evolutions from the ferroelectric phase to the paraelectric phase can be used for the information encryption discussed in the following section.
 |
| Fig. 2 Crystal structure evolution. (a) AFM-IR characterization of the ferroelectric dot arrays. (b) AFM-IR spectra of exposed P(VDF-TrFE-CTFE) at the exposure dose of 5, 12, 19, 30, 38, and 58 μm cm−2. (c) Topography image, AFM-IR absorption image on (d) 1277 cm−1, and (e) 1209 cm−1 laser. The scale bar is about 8 μm. The amplitude vs. distance line of each nanocell from the IR image on (f) 1209 cm−1, and (g) 1277 cm−1. | |
Electron-beam write-only-memory (EB-WOM).
To achieve electron-beam write-only-memory, electron beam exposure as a controllable tool induces the structural defects into the main chains to form a metastable phase, which can be used in information storage and encryption. A 4 × 4 array of the ferroelectric pattern was written with different exposure doses. The effect of the electron beam on the domain structure, size, and orientation in the exposed P(VDF-TrFE-CTFE) was conducted using piezoresponse force microscopy (PFM), as shown in Fig. 3a, b and Fig. S4a.† The result is displayed in the PFM phase diagram shown in Fig. 3c, the color zones from blue to green represent different dipole orientations. When four writing doses, such as 5, 15, 30, and 58 μC cm−2, were imposed the obtained dots exhibited four dipole orientations with four phase values varied from 30° to 5°, which were marked as “1”, “2”, “3”, and “4”. In addition, four-level-information dots have typical ferroelectric properties, such as the coercive field and piezoelectric response. At the exposure dose of 5 μC cm−2, the coercive field of the exposed P(VDF-TrFE-CTFE) dot is about 10 MV m−1, and the corresponding amplitude is 35 nA, which indicates P(VDF-TrFE-CTFE) still showed good relaxor ferroelectric properties similar to the properties of pristine P(VDF-TrFE-CTFE). At the exposure dose of 58 μC cm−2, the coercive field is only 2 MV m−1 and the maximum polarization is 9 nA, as shown in Fig. S7b.† For example, a “lucky clover” pattern was written by two exposure doses of 4, and 58 μC cm−2, as shown in Fig. 3e. It is found that two leaves in the upper zone at a high exposure dose of 58 μC cm−2 showed thicker film, lower amplitude, and phase value than those by the leaves at 4 μC cm−2 at the down zone, indicating that two meta-stable ferroelectric domains were formed. Along the white-dashed line (Fig. 3e), two stable phase values of 45°, and 25° in the pattern, as shown in Fig. S8b,† can serve as two storage states to encrypt the information in the pattern as digital watermarking. Compared to the pattern at an exposure dose of 58 μC cm−2 (Fig. S5a†), the pattern of the phase difference between 4 μC cm−2 and 24 μC cm−2 decreased to 5°, as shown in Fig. S8c† along the white-dashed line. As a result, the information could be encoded and stored in the pattern with different meta-stable phases with different domain sizes and orientations by the precise control of the exposure doses, which served as a new technology of electron-beam write-only-memory (WOM) for digital watermarking to improve the information security.
 |
| Fig. 3 Electron-beam write-only-memory (EB-WOM). (a) AFM height, and (b) PFM phase image of ferroelectric patterns with the square area of 8 μm × 8 μm at different exposure doses from 4 to 114 μC cm−2. (c) The statistics of the PFM phase value of four selected zones of 5, 15, 30, and 58 μC cm−2, as shown in Fig. 3(b). (d) PFM phase-electric field butterfly loops of P(VDF-TrFE-CTFE) films under the exposure doses of 5, 15, 30, and 58 μC cm−2. (e) Topography, PFM amplitude, and PFM phase imaging of irradiated P(VDF-TrFE-CTFE) pattern encrypted by two doses of 4 and 58 μC cm−2. | |
An electron-beam/electric multiplexing P(VDF-TrFE-CTFE) memory.
To achieve high-security-level information encryption, a multiplexing P(VDF-TrFE-CTFE) memory that functions concurrently as an electron-beam write-only-memory (EB-WOM) and variable-phase FeRAM was develeped. For the first information encryption (Fig. 4a), the capital letter “S” was also precisely written in patterns with a relatively high exposure dose of 30 μC cm−2, and other dots in the background were written with a low exposure dose of 4 μC cm−2, which was developed as digital watermarking for high-security-level information encryption. For the ferroelectric memory, a DC voltage pulse with an amplitude of ±10 V was modulated by a high-frequency AC voltage, which was applied to switch the ferroelectric domain for data writing, and reading process, as shown in the down position of Fig. S9.† After poling by a DC voltage of ±10 V, two states in the exposed P(VDF-TrFE-CTFE) dot at the dose of 4 μC cm−2 could be read out. The “0” state at 0° is a yellow-color line, and the “1” state at −160° is a green-color line, which is a typical binary bi-level nonvolatile memory, as shown in Fig. 4b. The value of the phase contrast is about 160°. The exposed P(VDF-TrFE-CTFE) dot at the dose of 30 μC cm−2 and the two stable state was still achieved. However, at a high dose of 30 μC cm−2 (Fig. 4c), the P(VDF-TrFE-CTFE) dots at the letter “S” form the relaxor ferroelectric domains, and the bi-polarization states were still built. The green line at −150° can be relaxed near the pink line at −20°, which served as an encoded state “1′” to improve the information security and capacitor. Therefore, at different exposure doses, the meta-stable phases have different phase contrasts, which can be used for second information encryption for high-security-level information encryption.
 |
| Fig. 4 An electron-beam/electric multiplexing P(VDF-TrFE-CTFE) memory. (a) Irradiated P(VDF-TrFE-CTFE) dot array was encrypted by two doses of 4 and 30 μC cm−2, and the “S” letter was exposed to 30 μC cm−2. Scheme of specifically designed voltage sequences for multiple data write-erase cycle of single-nanoscale data storage, and the recording phase angle dependence on time in the exposed P(VDF-TrFE-CTFE), (b) at 4 μC cm−2, and (c) 30 μC cm−2. | |
Conclusions
We demonstrated that high-density dot and grating arrays with 200 nm resolution could be successfully written using a template-free EBL technology, which may provide a new direction in using it for the electron-beam/electric multiplexing FeRAM based on domain-controllable engineering. Upon electron-beam exposure, noticeable enhancement in the absorption value of non-nanopolar domains at a characteristic amplitude of 1209 cm−1 and suppression in those of nanopolar domains at a characteristic amplitude of 1277 cm−1 indicates that the electron beam can determine domain size and orientation, as is proved by AFM-IR. For the information storage and encryption, a capital letter “S” was precisely written in the micro-patterns by controlling two different exposure doses, which was developed as a new type of electron-beam write-only-memory (WOM) based on domain evolutions and dipole orientations for digital watermarking. Secondly, the bi-polarization states were still built up in the exposed P(VDF-TrFE-CTFE)-based memory during the data writing/reading process, but the meta-stable phases show different phase contrast, which can be used for second information encryption. Our strategy enables on-demand programming on domain structure by controlling exposure doses, which can offer prospects for nano-fabrication of high-density ferroelectric patterns for high-security-level multiplexing memory.
Experimental section
Materials
P(VDF-TrFE-CTFE) (65/31/4 mol) powder was purchased from Piezotech Arkema, Butanone was supplied by Aladdin Company, China. N,N-Dimethylformamide(DMF) was purchased by Aladdin Reagent Company, China.
Preparation of the bulk P(VDF-TrFE-CTFE) membrane
For dielectric and ferroelectric testing, a P(VDF-TrFE-CTFE) thin film with a thickness of about 30 μm was prepared as follows: first, 0.2 g P(VDF-TrFE-CTFE) powder was dissolved in the 4 ml N,N-dimethylformamide (DMF). Second, the P(VDF-TrFE-CTFE) solution was poured into the round plate with a radius of 26 mm and then dried for 24 h at 60 °C. To further remove the residual solvents the obtained membrane was dried under a high vacuum at 60 °C for 24 h. Furthermore, the thin membrane was hot-pressed at the temperature of 140 °C under the pressure of 2.5–3 MPa. Finally, two gold electrodes with an area of 7.065 mm2 were sputtered onto the surfaces of the membrane in a vacuum using a carbon coater (SBC-12).
To prepare P(VDF-TrFE-CTFE) patterns by electron beam lithography. First, the P(VDF-TrFE-CTFE) powers with a mass of 25 mg were dissolved in 5 ml butanone to obtain P(VDF-TrFE-CTFE) solution with various concentrations of 5 mg ml−1. The P(VDF-TrFE-CTFE) solutions were deposited onto the silicon wafer by spin-coating at 3000 rpm for 45 s. The film thickness was controlled by the concentration of P(VDF-TrFE-CTFE) solutions. Then, the P(VDF-TrFE-CTFE) films were annealed at 110 °C for 5 hours.
Electron beam lithography.
The area-dose test, “s” patterns, lucky clover patterns, nanodot arrays, and line arrays in the annealed P(VDF-TrFE-CTFE) membrane were outlined using the electron beam lithography (Elphy Quantum system, Raith Gmbh, Germany) with the acceleration voltage of 20 kV. The area dose was varied between 4 and 277 μC cm−2. The optimized area doses for “lucky clover” patterns were selected as 4, 30, and 58 μC cm−2. For high-density patterns, a dot dose of 0.1 fC per dot was selected for nanodot arrays, and the line dose for line arrays. After exposure, N,N-dimethylformamide, and 2-butanone served as the negative-tone developer to dissolve the un-exposed areas for 30 s. The developed samples were rinsed with isopropanol and dried with N2 blowing before the following characterizations.
Dielectric characterization.
The dielectric properties of the materials were measured using a precision LCR instrument (TH2827C, pass-light) in the frequency range of 0.1 kHz to 1000 kHz at temperatures of −40 °C and 120 °C. Prior to testing, open circuit compensation and short circuit compensation were performed.
Ferroelectric characterization.
The bipolar P–E hysteresis loop measurements were obtained using an AI-based ferroelectric system (Poly K) equipped with voltage amplifiers capable of delivering up to 10
000 V at a testing frequency of 10 Hz. Additionally, the breakdown electric fields were determined utilizing a high-voltage power supply (DW-P503-1ACDFO).
Scanning force microscopy characterization.
Surface morphology analysis was conducted using scanning force microscopy (Ntegra Prima, NT-MDT, Russia) in tapping and phase contrast modes, operating at a resonant frequency of 70 kHz. Subsequently, nanoscale ferroelectric properties of the nanocomposite were examined using the contact mode, by applying an AC voltage of 3 V between the tip (HA-FM, Pt coating) and the substrate at a resonant frequency of 280 kHz, with a force constant of 6 N m−1 for the PFM tip. The samples for testing were prepared by exposing P(VDF-TrFE-CTFE) films to butanone for 30 s, followed by annealing at 60 °C for 2 h.
Morphology characterization.
The morphology of the prepared dot and grating arrays were examined using a scanning electron microscope (SEM, Sigma 300, Carl Zeiss).
AFM-IR statistical analysis.
The AFM-IR data was acquired and analyzed using the Analysis Studio v3.15. AFM-IR spectra were used to analyze the bands at 1277 cm−1and 1209 cm−1.
Author contributions
Y. X. C., J. Z., and X. F. Z. conceived the idea and directed the research. Y. S. L. and Y. X. C. conducted all the experiments. Y. S. L. performed the data analysis. Y. X. C. and Y. S. L. wrote the paper. Y. X. C., J. Z., and X. F. Z. revised the manuscript. We are grateful for the helpful discussions and technical assistance of PFM testing.
Conflicts of interest
The authors declare no conflict of interest.
Acknowledgements
This work was supported by the Zhejiang Provincial Natural Science Foundation (No. LY21E030007), the National Science Fund for Distinguished Young Scholars (52225312), National Natural Science Foundation of China (No. 52173020 and No. 51972292), the Fundamental Research Funds for the Provincial Universities of Zhejiang (GK219909299001-003), the Zhejiang Provincial Key Research and Development Program (2023C01053), Open Research Project of Zhejiang Lab (No. 2022MG0AB02).
References
- M. Yang, L. Zhu, Q. Zhong, R. El-Ganainy and P. Y. Chen, Spectral Sensitivity near Exceptional Points as a Resource for Hardware Encryption, Nat. Commun., 2023, 14, 1145 CrossRef CAS PubMed
.
- T. Ma, T. Li, L. Zhou, X. Ma, J. Yin and X. Jiang, Dynamic Wrinkling Pattern Exhibiting Tunable Fluorescence for Anticounterfeiting Applications, Nat. Commun., 2020, 11, 1811 CrossRef CAS
.
- J. Bai, Z. Shi and X. Jiang, “Acrostic” Encryption: Stress–Manipulation on Information Display, Adv. Funct. Mater., 2023, 2301797 CrossRef CAS
.
- F. Zhou, Z. Zhou, J. Chen, T. H. Choy, J. Wang, N. Zhang, Z. Lin, S. Yu, J. Kang and H. S. P. Wong, Etal Optoelectronic Resistive Random Access Memory for Neuromorphic Vision Sensors, Nat. Nanotechnol., 2019, 14, 776–782 CrossRef CAS PubMed
.
- Y. Xia, J. Wang, R. Chen, H. Wang, H. Xu, C. Jiang, W. Li and X. Xiao, 2d Heterostructure of Bi2o2se/Bi2seox Nanosheet for Resistive Random Access Memory, Adv. Electron. Mater., 2022, 8, 2200126 CrossRef CAS
.
- N. Sato, F. Xue, R. M. White, C. Bi and S. X. Wang, Two-Terminal Spin–Orbit Torque Magnetoresistive Random Access Memory, Nat. Electron., 2018, 1, 508–511 CrossRef CAS
.
- S. Jung, H. Lee, S. Myung, H. Kim, S. K. Yoon, S.-W. Kwon, Y. Ju, M. Kim, W. Yi and S. Han, Etal A Crossbar Array of Magnetoresistive Memory Devices for in-Memory Computing, Nature, 2022, 601, 211–216 CrossRef CAS
.
- Y. Chen, L. Zhang, J. Liu, X. Lin, W. Xu, Y. Yue and Q.-D. Shen, Ferroelectric Domain Dynamics and Stability in Graphene Oxide-P(Vdf-Trfe) Multilayer Films for Ultra-High-Density Memory application, Carbon, 2019, 144, 15–23 CrossRef CAS
.
- M. Guo, J. Jiang, J. Qian, C. Liu, J. Ma, C. W. Nan and Y. Shen, Flexible Robust and High–Density Feram from Array of Organic Ferroelectric Nano–Lamellae by Self–Assembly, Adv. Sci., 2019, 6, 1801931 CrossRef
.
- H. Sun, J. Wang, Y. Wang, C. Guo, J. Gu, W. Mao, J. Yang, Y. Liu, T. Zhang and T. Gao, Etal Nonvolatile Ferroelectric Domain Wall Memory Integrated on Silicon, Nat. Commun., 2022, 13, 4332 CrossRef CAS PubMed
.
- X. Chen, X. Han and Q.-D. Shen, Pvdf-Based Ferroelectric Polymers in Modern Flexible Electronics, Adv. Electron. Mater., 2017, 3, 1600460 CrossRef
.
- S. He, M. Guo, Y. Wang, Y. Liang and Y. Shen, An Optical/Ferroelectric Multiplexing Multidimensional Nonvolatile Memory from Ferroelectric Polymer, Adv. Mater., 2022, 34, 2202181 CrossRef CAS PubMed
.
- Y. J. Park, S. J. Kang, B. Lotz, M. Brinkmann, A. Thierry, K. J. Kim and C. Park, Ordered Ferroelectric Pvdf−Trfe Thin Films by High Throughput Epitaxy for Nonvolatile Polymer Memory, Macromolecules, 2008, 41, 8648–8654 CrossRef CAS
.
- X. Chen, H. Qin, X. Qian, W. Zhu, B. Li, B. Zhang, W. Lu, R. Li, S. Zhang, L. Zhu, F. Domingues Dos Santos, J. Bernholc and Q. M. Zhang, Relaxor Ferroelectric Polymer Exhibits Ultrahigh Electromechanical Coupling at Low Electric Field, Science, 2022, 375, 1418–1422 CrossRef CAS PubMed
.
- X. Chen, H. Qin, W. Zhu, B. Zhang, W. Lu, J. Bernholc and Q. M. Zhang, Giant Electrostriction Enabled by Defect-Induced Critical Phenomena in Relaxor Ferroelectric Polymers, Macromolecules, 2023, 56, 690–696 CrossRef CAS
.
- B. Chai, K. Shi, Y. Wang, Y. Liu, F. Liu, P. Jiang, G. Sheng, S. Wang, P. Xu and X. Xu, Etal Modulus-Modulated All-Organic Core-Shell Nanofiber with Remarkable Piezoelectricity for Energy Harvesting and Condition Monitoring, Nano Lett., 2023, 23, 1810–1819 CrossRef CAS PubMed
.
- X.-Z. Chen, Q. Li, X. Chen, X. Guo, H.-X. Ge, Y. Liu and Q.-D. Shen, Nano-Imprinted Ferroelectric Polymer Nanodot Arrays for High Density Data Storage, Adv. Funct. Mater., 2013, 23, 3124–3129 CrossRef CAS
.
- Y. Hu, X. Wang, M. Zhang, S. Wang, S. Li and G. Chen, A Hierarchical Anodic Aluminum Oxide Template, Nano Lett., 2021, 21, 250–257 CrossRef CAS
.
- Y. Chen, M. Xu, X. Hu, Y. Yue, X. Zhang and Q. Shen, High-Resolution Structural Mapping and Single-Domain Switching Kinetics in 2d-Confined Ferroelectric Nanodots for Low-Power Feram, Nanoscale, 2020, 12, 11997–12006 RSC
.
- B. Kim, J. Hwang, J. Yi, D. R. Kim, A. Urbas, Z. Ku and C. H. Lee, Replicable Quasi-Three-Dimensional Plasmonic Nanoantennas for Infrared Bandpass Filtering, ACS Appl. Mater. Interfaces, 2021, 13, 24024–24031 CrossRef CAS
.
- Z. Hu, M. Tian, B. Nysten and A. M. Jonas, Regular Arrays of Highly Ordered Ferroelectric Polymer Nanostructures for Non-Volatile Low-Voltage Memories, Nat. Mater., 2009, 8, 62–67 CrossRef CAS PubMed
.
- P. Sharma, T. J. Reece, S. Ducharme and A. Gruverman, High-Resolution Studies of Domain Switching Behavior in Nanostructured Ferroelectric Polymers, Nano Lett., 2011, 11, 1970–1975 CrossRef CAS PubMed
.
- M. Alexe, C. Harnagea, D. Hesse and U. Gösele, Patterning and Switching of Nanosize Ferroelectric Memory Cells, Appl. Phys. Lett., 1999, 75, 1793–1795 CrossRef CAS
.
- P. Viswanath, K. K. H. De Silva and H.-H. Huang, Masamichi Yoshimura, Large Piezoresponse in Ultrathin Organic Ferroelectric Nano Lamellae through Self-Assembly Processing, Appl. Surf. Sci., 2020, 532, 147188 CrossRef CAS
.
- Y. Guang, Y. Peng, Z. Yan, Y. Liu, J. Zhang, X. Zeng, S. Zhang, S. Zhang, D. M. Burn and N. Jaouen, Etal Electron Beam Lithography of Magnetic Skyrmions, Adv. Mater., 2020, 32, 2003003 CrossRef CAS
.
- H. J. Jeon, J. W. Leem, Y. Ji, S. M. Park, J. Park, K. Y. Kim, S. W. Kim and Y. L. Kim, Cyber–Physical Watermarking with Inkjet Edible Bioprinting, Adv. Funct. Mater., 2022, 32, 2112479 CrossRef CAS
.
- A. Speidel, I. Bisterov and A. T. Clare, Direct Writing Unclonable Watermarks with an Electrochemical Jet, Adv. Funct. Mater., 2022, 32, 2208116 CrossRef CAS
.
- Z. Long, J. Zhou, J. Qiu, Q. Wang, Y. Li, J. Wang, D. Zhou, Y. Yang, H. Wu and Y. Wen, Thermal Engineering of Electron-Trapping Materials for “Smart-Write-in” Optical Data Storage, Chem. Eng. J., 2021, 420, 129788 CrossRef CAS
.
- J. Zhang, D. Khanal and M. M. B. Holl, Applications of Afm-Ir for Drug Delivery Vector Characterization: Infrared, Thermal, and Mechanical Characterization at the Nanoscale, Adv. Drug Delivery Rev., 2023, 192, 114646 CrossRef CAS PubMed
.
- Q. Zeng, H. Wang, Z. Xiong, Q. Huang, W. Lu, K. Sun, Z. Fan and K. Zeng, Nanoscale Ferroelectric Characterization with Heterodyne Megasonic Piezoresponse Force Microscopy, Adv. Sci., 2021, 8, 2003993 CrossRef CAS
.
|
This journal is © The Royal Society of Chemistry 2024 |
Click here to see how this site uses Cookies. View our privacy policy here.