DOI:
10.1039/D4OB00966E
(Communication)
Org. Biomol. Chem., 2024,
22, 5891-5896
A HMPA–H2O mediated oxygenative carbocyclization of 2-alkynylphenyl-substituted p-quinone methides to indenones†
Received
7th June 2024
, Accepted 29th June 2024
First published on 1st July 2024
Abstract
Herein, we report a transition-metal and base-free protocol to access a wide range of functionalized indenone derivatives through a HMPA–H2O-mediated oxygenative annulation of 2-alkynylphenyl-substituted p-quinone methides. This method worked effectively for most of the p-QMs investigated and the corresponding indenone derivatives were obtained in moderate to good yields. This methodology was further extended to the formal synthesis of one of the resveratrol based natural products, (±)-isopaucifloral F.
Introduction
Indenone is considered a privileged core that is an integral part of many natural products and also serves as an important structural motif in pharmaceutical chemistry and materials science.1 Besides, indenone derivatives also serve as synthons/intermediates in the preparation of many important organic compounds including drug candidates.2Fig. 1 shows some indenone derivatives that show potential biological activities such as antitumor,3 COX-2 inhibition,4 and estrogen binding5 activities.
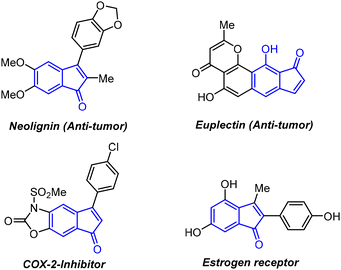 |
| Fig. 1 Indenone-based bio-active molecules. | |
The traditional method for indenone synthesis relies on intramolecular Friedel–Crafts type cyclization that often suffers from the need for harsh reaction conditions and a limited substrate scope.6 Besides, some radical and other synthetic approaches have also been developed to produce indenone derivatives.7 In recent years, transition metal catalysis has gained much attention in organic transformations and, to this end, many transition metal based protocols have also been documented in the literature for the synthesis of indenones,8 which include catalytic annulations of alkynes with ortho-functionalized aromatic aldehydes, esters or nitriles (a & b, Scheme 1).8b,c However, the use of expensive transition metal catalysts such as Pd and Rh limits their synthetic utility. Recently, Yao and co-workers reported a transition metal free tandem annulation of 2-alkynylbenzaldehydes with phenols for the synthesis of 2,3-diarylindenones (c, Scheme 1).9 However, their protocol is effective only for electron-poor aryl-substituted 2-alkynyl benzaldehydes. Moreover, both the regioisomers were formed in their method; of course, one would be the major product depending on the reaction conditions (c, Scheme 1). Considering the importance of indenone scaffolds in various fields, the development of more efficient and practical metal-free protocols to access these indenone derivatives are highly desirable.
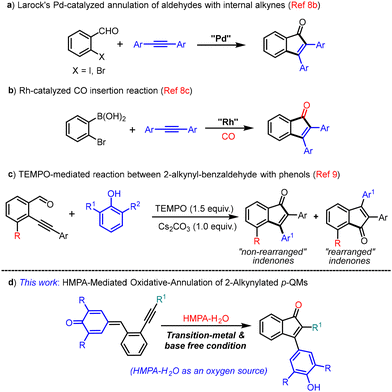 |
| Scheme 1 Selected reported protocols and our hypothesis. | |
In recent years, the synthetic utilities of p-quinone methides (p-QMs) have been widely explored.10 Our group was also involved in the synthesis of various triarylmethanes,11 carbocycles12 and heterocycles13 using p-QMs as synthons. Herein, we report a transition-metal and base-free HMPA-mediated oxygenative carbocyclization of 2-alkynylated p-QMs14 to access substituted indenones (d, Scheme 1). In this context, it should be noted that although HMPA has been utilized as an additive in many organic transformations15 including in SmI2 chemistry,16 only a handful of reports are available in the literature on HMPA-mediated organic transformations, which include regioselective additions of organolithium reagents to enones and enals, conjugate additions of (phenylthio/phenylseleno)allylithium reagents to cyclopentenones, retro-aldol reactions, arylations of indenes, etc.17
Results and discussion
To find out the best reaction conditions, the 2-alkynylphenyl-substituted p-QM 1a was chosen as the model substrate, and it was subjected to the cyclization reaction under different conditions (Table 1). Initially, we decided to use Au-based catalysts, as they are well known for activating the alkynes, and DMSO as the oxygenating source. However, the cyclization of 1a with AuCl in DMSO did not yield the expected product 2a even after 24 hours at room temperature (entry 1). Interestingly, when the reaction temperature was increased to 100 °C, the starting material 1a was completely consumed (as observed by TLC) after 24 h, and product 2a was isolated in 60% yield (entry 2). The structure of 2a was unambiguously confirmed by NMR and X-ray analysis (CCDC 2307508†). Upon changing the catalyst from gold(I) chloride to AuCl3, a slight decrease in the yield of 2a was observed (entry 3). Later, a couple of other gold-based catalysts such as (PPh3)AuCl and PPh3AuNTf2 were examined (entries 4 & 5), and in these cases, only trace amounts of 2a was observed. In addition, one reaction was conducted using a combination of gold and silver catalysts (entry 6); however, unfortunately, in that case too, only a trace amount of product formation was seen. When the reaction was performed using AuCl in commercially available HMPA (98.5% HMPA + 1.5% water) as a solvent, 2a was obtained in 53% yield in 8 hours (entry 7). Excited by this result, further optimization experiments were conducted in HMPA. To our surprise, the reaction of 1a in HMPA at 100 °C without any Au-catalyst also yielded the same product 2a in 60% yield (entry 8). We found this result very interesting as the reaction worked without any external oxygenating source and it hinted that HMPA is probably promoting the reaction and also acting as an oxygenating agent. Since HMPA has not been utilized as a promoter for any cyclization reactions, including the cyclization of 1a to 2a, we became interested in investigating this transformation in detail. We found that reaction temperature plays an important role in the cyclization of 1a. For example, increasing the temperature to 140 °C significantly improved the yield of 2a to 78% (entry 9). Another experiment was conducted in DMSO at 130 °C without the Au-catalyst and, in that case, 2a was not detected at all (entry 10). Interestingly, no product formation was observed when the reaction was conducted either in dry HMPA (entry 11) or in H2O (entry 12) under reflux conditions. However, when a 3
:
1 mixture of dry HMPA and water was used as a solvent, 2a was obtained in 30% yield (entry 13). Another experiment was also conducted using the commercially available N-methylpyrrolidone (NMP); however, the desired product 2a was formed only in 10% yield (entry 14). To exclude the effect of atmospheric oxygen on the outcome of the reaction, a reaction was conducted under inert conditions (entry 15) and in that case also, the product was obtained in 75% yield, clearly indicating that atmospheric oxygen is not playing any role in this transformation.
Table 1 Optimization studya
Next, the optimal reaction conditions (entry 9, Table 1) were employed to investigate the scope and limitations of this transformation (Table 2). The para-quinone methides (p-QMs) 1b–h, where the alkyne was substituted with electron-rich arenes, produced the desired products 2b–h in 40–86% yields. The halo-aryl alkyne-substituted p-QMs, 1i–m, also reacted smoothly and afforded the desired products 2i–m in 63–74% yields. In addition, the p-QMs 1n–q, substituted with electron-withdrawing groups, also underwent cyclization under the optimal conditions and provided the corresponding products 2n–q in the range of 50–87% yields. The 3-thienyl alkyne-substituted precursor 1r afforded the desired product 2r in 60% yield. The reaction also worked well with p-QMs 1s–u, having cycloalkyl substituents (such as cyclopropyl, cyclopentyl, and cyclohexyl) on the alkyne, and in these cases, the desired products 2s–u were isolated in 39–52% yields.
Reactions were carried out at the 50 mg scale with 1b–u in 1.0 mL of HMPA. Yields reported are isolated yields.
|
|
Later, the substrate scope investigation was extended to various substituted p-QMs 1v–1aa and the results are summarized in Table 3. The p-QMs 1v–x, having electron-rich substituents (–Me, –OMe, and 3,5-dimethoxy) on the aryl ring, afforded the corresponding products 3a–c in yields ranging from 53–61%. The halo-arene-substituted p-QMs 1y and 1z also reacted under the standard reaction conditions and produced the desired indenones 3d and 3e in 35 and 60% yields, respectively. The indenone 3f was isolated in 54% yield when the corresponding synthon 1aa was subjected to oxygenative cyclization under standard conditions. The isopropyl containing 2-alkynylphenyl-substituted p-QM 1ab also worked well under the optimized conditions and afforded product 3g in 80% yield.
Reactions were carried out at the 50 mg scale with 1v–1aa in 1.0 mL of HMPA. Yields reported are isolated yields.
|
|
To show the feasibility of this methodology, a relatively large-scale reaction of 1a was carried out under the standard reaction conditions, and in this case, 2a was obtained in 62% yield (Scheme 2).
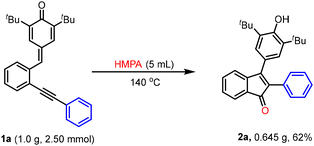 |
| Scheme 2 Gram scale reaction of 1a. | |
To demonstrate the practical applicability of this transformation, we targeted the formal synthesis of one of the resveratrol-derived natural products, isopaucifloral F (8),18 starting from p-QM 4.12a Upon treatment with the terminal alkyne 5, p-QM 4 afforded the 2-alkynylphenyl-substituted p-QM 6 under Sonogashira conditions (Scheme 3). p-QM 6 was then subjected to oxygenative cyclization under the optimized reaction conditions to produce the indenone derivative 7 in 53% yield. Compound 7 can easily be converted to isopaucifloral F 8 in 3 steps through a known procedure involving reduction followed by demethylation/de-tert-butylation.12a
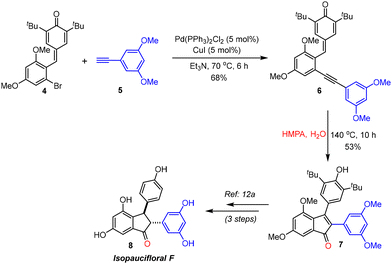 |
| Scheme 3 Formal synthesis of (±)-isopaucifloral F 8. | |
Next, we shifted our attention to find out the exact role of HMPA/water in this transformation. As discussed in the optimization table, the oxygenative cyclization of 1a did not work either in anhydrous HMPA or in pure water (entries 11 & 12, Table 1). However, when the reaction was conducted in a mixture of dry HMPA and water in a 3
:
1 ratio, 2a was obtained in 30% yield (entry 13, Table 1). These experiments clearly indicate that both HMPA and water are crucial to drive this transformation. The best result was obtained when the commercially available HMPA with 1.5% water content was used (entry 9, Table 1). To find out the optimal quantity of water required for this transformation, a few control experiments were performed in anhydrous HMPA by adding calculated amounts of water [0.5%, 1.0%, 1.5%, 2.0%, etc.] at 140 °C (a, Scheme 4). The reaction using 1.5% water gave product 2a in 74% yield, which is very similar when compared to the optimal reaction conditions using commercially available HMPA (entry 9, Table 1). However, interestingly, product 2a was obtained in much less yields when the water content in the reaction is less than or more than 1.5% (v/v) [Scheme 4]. These experiments clearly indicate that the optimal water quantity required for this transformation is approximately 1.5% (v/v). It is clear from these control experiments that the yields of 2a were decreasing gradually when the water content in the reaction was increased and, notably, no product formation was observed when the reaction was conducted in a 1
:
1 mixture of dry HMPA and water (a, Scheme 4). To further prove the involvement of water in the reaction, another control experiment was performed using a mixture of dry HMPA and 18O labelled water (H2O18) and, in this case, 2a′ was obtained in 30% yield with 60% of 18O incorporation (M − H, m/z = 411.2215) along with an unlabelled product (M − H, m/z = 409.2125) [b, Scheme 4]. We believe that the oxygen atom of the unlabelled product could have come from the HMPA, which explains the incorporation of the remaining 40% of 16O in the product.
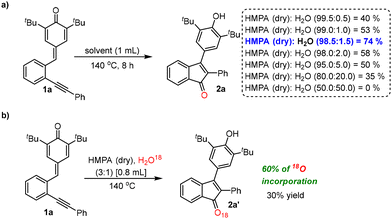 |
| Scheme 4 Control experiments. | |
Based on the above-mentioned control experiments, a plausible mechanism for this transformation was proposed (Scheme 5). We presume that, initially, the oxygen-atom of HMPA reacts with 1a in a 1,6-fashion to generate intermediate I. Now, there are two possibilities: (i) the attack of water on the alkyne moiety of I followed by cyclization with the expulsion of HMPA would lead to another intermediate II, which subsequently undergoes tautomerization (viaIII) followed by aerobic oxidation to generate product 2a (Path A) or (ii) the attack of another molecule of HMPA on the alkyne of I followed by intramolecular cyclization would lead to intermediate IV, which then reacts with water to generate another intermediate V. The expulsion of HMPA from intermediate V would lead to III, which upon aerobic oxidation produces product 2a (Path B). The HRMS analysis of the crude reaction mixture indicated the presence of either I or IV or both during the reaction as a peak was found at m/z (M + H)+ = 574.3555, which corresponds to I and/or IV. So, based on this observation and the control experiment (Scheme 5), we assume that both the pathways (Paths A & B) are simultaneously operating during the reaction.
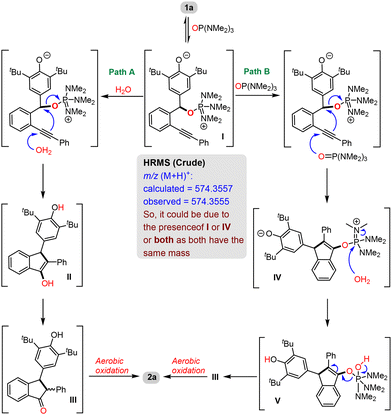 |
| Scheme 5 Plausible mechanism. | |
Conclusions
In conclusion, we have developed a HMPA–H2O mediated simple method for the synthesis of functionalized indenone derivatives through the oxygenative carbocyclization of 2-alkynylphenyl-substituted p-QMs. Many indenone derivatives could be accessed in moderate to good yields using this protocol. In addition, this transformation was also extended to the formal synthesis of isopaucifloral F. Considering the importance of indenones in medicinal chemistry, we believe that this protocol will definitely find some applications in the synthesis of other indenone-based natural products in the near future.
Data availability
The data supporting this article have been included as part of the ESI.†
Conflicts of interest
There are no conflicts to declare.
Acknowledgements
The authors are grateful to the Science and Engineering Research Board (SERB) of the Department of Science and Technology (DST), Govt. of India (CRG/2022/005391) and IISER Mohali for the financial support. F. A. thanks UGC, New Delhi for a research fellowship. S. F., Y. P. and P. K. R. thank IISER Mohali for a research fellowship. We are grateful for the NMR and HRMS facilities at IISER Mohali.
References
- For selected examples, please see:
(a) R. Nigam, K. R. Babu, T. Ghosh, B. Kumari, D. Akula, S. N. Rath, P. Das, R. Anindya and F. A. Khan, Bioorg. Med. Chem., 2018, 26, 4100–4112 CrossRef CAS PubMed
;
(b) J. H. Ahn, M. S. Shin, S. H. Jung, S. K. Kang, K. R. Kim, S. D. Rhee, W. H. Jung, S. D. Yang, S. J. Kim, J. R. Woo, J. H. Lee, H. G. Cheon and S. S. Kim, J. Med. Chem., 2006, 49, 4781–4784 CrossRef CAS PubMed
;
(c) K. Morinaka, T. Ubukata and Y. Yokoyama, Org. Lett., 2009, 11, 3890–3893 CrossRef CAS PubMed
.
- For selected examples, see:
(a) N. Parui, T. Mandal and J. Dash, Eur. J. Org. Chem., 2023, e202201285 CrossRef CAS
;
(b) H. Lahousse, H. J. Martens, S. Toppet and G. J. Hoornaert, J. Org. Chem., 1982, 47, 2001–2005 CrossRef CAS
;
(c) J. L. Jeffrey and R. Sarpong, Org. Lett., 2009, 11, 5450–5453 CrossRef CAS PubMed
.
-
(a) D. C. Harrowven, N. A. Newman and C. A. Knight, Tetrahedron Lett., 1998, 39, 6757–6760 CrossRef
;
(b) L. M. X. Lopes, M. Yoshida and O. R. Gottlieb, Phytochemistry, 1984, 23, 2021–2024 CrossRef CAS
;
(c) M. A. Ernst-Russell, C. L. L. Chai, J. H. Wardlaw and J. A. Elix, J. Nat. Prod., 2000, 63, 129–131 CrossRef CAS PubMed
.
- C. H. Park, X. Siomboing, S. Yous, B. Gressier, M. Luyckx and P. Chavatte, Eur. J. Med. Chem., 2002, 37, 461–468 CrossRef CAS PubMed
.
- R. E. McDevitt, M. S. Malamas, E. S. Manas, R. J. Unwalla, Z. B. Xu, C. P. Miller and H. A. Harris, Bioorg. Med. Chem. Lett., 2005, 15, 3137–3142 CrossRef CAS PubMed
.
-
(a) M. B. Floyd and G. R. Allen Jr, J. Org. Chem., 1970, 35, 2647–2653 CrossRef CAS
;
(b) P. Galatsis, J. J. Manwell and J. M. Blackwell, Can. J. Chem., 1994, 72, 1656–1659 CrossRef CAS
;
(c) G. Roberge and P. Brassard, Synth. Commun., 1979, 9, 129–139 CrossRef CAS
.
- For selected recent examples, see:
(a) For reviews on the radical cascade-based reactions to access indenones, see: Y. Zhang, K. Sun, Q. Lv, X. Chen, L. Qu and B. Yu, Chin. Chem. Lett., 2019, 30, 1361–1368 CrossRef CAS
;
(b) X. Yan, S. Zou, P. Zhao and C. Xi, Chem. Commun., 2014, 50, 2775–2777 RSC
;
(c) P. Zhao, Y. Liu and C. Xi, Org. Lett., 2015, 17, 4388–4391 CrossRef CAS PubMed
;
(d) S. K. Pagire, P. Kreitmeier and O. Reiser, Angew. Chem., Int. Ed., 2017, 56, 10928–10932 CrossRef CAS PubMed
;
(e) J. Wen, W. Shi, F. Zhang, D. Liu, S. Tang, H. Wang, X. M. Lin and A. Lei, Org. Lett., 2017, 19, 3131–3134 CrossRef CAS PubMed
;
(f) F. Jafarpour, M. Azizzade, Y. Golpazir-Sorkheh, H. Navid and S. Rajai-Daryasarei, J. Org. Chem., 2020, 85, 8287–8294 CrossRef CAS PubMed
;
(g) L. Li and G.-W. Wang, J. Org. Chem., 2021, 86, 14102–14112 CrossRef CAS PubMed
;
(h) W.-C. Yang, Y. Sun, L.-Y. Shen, X. Xie and B. Yu, Mol. Catal., 2023, 535, 112819 CrossRef CAS
;
(i) X.-T. Zhu, T.-S. Zhang, Q. Zhao, P.-J. Cai, W.-J. Hao, S.-J. Tu and B. Jiang, Chem. – Asian J., 2018, 13, 1157–1164 CrossRef CAS PubMed
;
(j) X.-T. Zhu, Q.-L. Lu, X. Wang, T.-S. Zhang, W.-J. Hao, S.-J. Tu and B. Jiang, J. Org. Chem., 2018, 83, 9890–9901 CrossRef CAS PubMed
.
- For selected examples, see:
(a) For reviews on transition-metal catalyzed synthesis of indenones, see: L. E. Hutchinson and D. J. Wilger, Adv. Synth. Catal., 2022, 364, 3441–3465 CrossRef CAS
;
(b) R. C. Larock, M. J. Doty and S. Cacchi, J. Org. Chem., 1993, 58, 4579–4583 CrossRef CAS
;
(c) Y. Harada, J. Nakanishi, H. Fujihara, M. Tobisu, Y. Fukumoto and N. Chatani, J. Am. Chem. Soc., 2007, 129, 5766–5771 CrossRef CAS PubMed
;
(d) H. Tsukamoto and Y. Kondo, Org. Lett., 2007, 9, 4227–4230 CrossRef CAS PubMed
;
(e) T. Miura and M. Murakami, Org. Lett., 2005, 7, 3339–3341 CrossRef CAS PubMed
;
(f) T. Morimoto, K. Yamasaki, A. Hirano, K. Tsutsumi, N. Kagawa, K. Kakiuchi, Y. Harada, Y. Fukumoto, N. Chatani and T. Nishioka, Org. Lett., 2009, 11, 1777–1780 CrossRef CAS PubMed
;
(g) B.-J. Li, H.-Y. Wang, Q.-L. Zhu and Z.-J. Shi, Angew. Chem., Int. Ed., 2012, 51, 3948–3952 CrossRef CAS PubMed
;
(h) Z. Qi, M. Wang and X. Li, Org. Lett., 2013, 15, 5440–5443 CrossRef CAS PubMed
;
(i) L. Kong, X. Yang, X. Zhou, S. Yu and X. Li, Org. Chem. Front., 2016, 3, 813–816 RSC
;
(j) Y. Kuninobu, T. Matsuki and K. Takai, Org. Lett., 2010, 12, 2948–2950 CrossRef CAS PubMed
;
(k) X. T. Zhu, Q. Zhao, F. Liu, A. F. Wang, P. J. Cai, W. J. Hao, S. J. Tu and B. Jiang, Chem. Commun., 2017, 53, 6828–6831 RSC
;
(l) F. Liu, Z. Dong, J. Wang and G. Dong, Angew. Chem., Int. Ed., 2019, 58, 2144–2148 CrossRef CAS PubMed
;
(m) M. Miao, M. Jin, P. Chen, L. Wang, S. Zhang and H. Ren, Org. Lett., 2019, 21, 5957–5961 CrossRef CAS PubMed
.
- T. Yao, S. Zhao, T. Liu, Y. Wu, Y. Ma, T. Li and X. Qin, Chem. Commun., 2022, 58, 4592–4595 RSC
.
- For recent reviews on the chemistry of p-QMs, see:
(a) C. G. S. Lima, F. P. Pauli, D. C. S. Costa, A. S. de Souza, L. S. M. Forezi, V. F. Ferriera and F. de Carvalho da Silva, Eur. J. Org. Chem., 2020, 2650–2692 CrossRef CAS
;
(b) J.-Y. Wang, W.-J. Hao, S.-J. Tu and B. Jiang, Org. Chem. Front., 2020, 7, 1743–1778 RSC
;
(c) G. Singh, R. Pandey, Y. A. Pankhade, S. Fatma and R. V. Anand, Chem. Rec., 2021, 21, 4150–4173 CrossRef CAS PubMed
;
(d) X. Liu, Y. Ren, L. Zhu, T. Li, W. Xu, Y. Liu, K.-W. Tang and B. Xiong, Tetrahedron, 2023, 148, 133655 CrossRef CAS
;
(e) Y. F. Gan, Y. Y. Li, X. Q. Chen, Y. Y. Guo and R. Wang, Synthesis, 2023, 1172–1186 CAS
.
-
(a) V. Reddy and R. V. Anand, Org. Lett., 2015, 17, 3390–3393 CrossRef CAS PubMed
;
(b) P. K. Ranga, F. Ahmad, P. Nager, P. S. Rana and R. V. Anand, J. Org. Chem., 2021, 86, 4994–5010 CrossRef CAS PubMed
.
-
(a) A. S. Jadhav, Y. A. Pankhade, R. Hazra and R. V. Anand, J. Org. Chem., 2018, 83, 10107–10119 CrossRef CAS PubMed
;
(b) Y. A. Pankhade, R. Pandey, S. Fatma, F. Ahmad and R. V. Anand, J. Org. Chem., 2022, 87, 3363–3377 CrossRef CAS PubMed
.
-
(a) F. Ahmad, P. K. Ranga, Y. A. Pankhade, S. Fatma, A. Gouda and R. V. Anand, Chem. Commun., 2022, 58, 13238–13241 RSC
;
(b) F. Ahmad, P. K. Ranga, S. Fatma, A. Kumar and R. V. Anand, Adv. Synth. Catal., 2023, 365, 3271–3276 CrossRef CAS
.
- For selected recent examples on the synthetic applications of 2-alkynylated p-quinone methides, see:
(a) H.-D. Zuo, C.-C. Cui, C. Guo, W.-J. Hao, S.-J. Tu and B. Jiang, Chem. – Asian J., 2020, 15, 4070–4076 CrossRef CAS PubMed
;
(b) H.-D. Zuo, W.-J. Hao, C.-F. Zhu, C. Guo, S.-J. Tu and B. Jiang, Org. Lett., 2022, 22, 4471–4477 CrossRef PubMed
;
(c) A. S. Jadhav, Y. A. Pankhade and R. V. Anand, J. Org. Chem., 2018, 83, 8615–8626 CrossRef CAS PubMed
.
- For selected examples, see:
(a) W. Wu, W. Dai, X. Ji and S. Cao, Org. Lett., 2016, 18, 2918–2921 CrossRef CAS PubMed
;
(b) D.-P. Jin, P. Gao, D.-Q. Chen, S. Chen, J. Wang, X.-Y. Wang, X.-Y. Liu and Y.-M. Liang, Org. Lett., 2016, 18, 3486–3489 CrossRef CAS PubMed
.
-
(a) D. V. Sadasivam, P. K. S. Antharjanam, E. Prasad and R. A. Flowers II, J. Am. Chem. Soc., 2008, 130, 7228–7229 CrossRef CAS PubMed
;
(b) K. A. Choquette, D. V. Sadasivam and R. A. Flowers II, J. Am. Chem. Soc., 2010, 132, 17396–17398 CrossRef CAS PubMed
;
(c) H. Ohno, R. Wakayama, S.-I. Maeda, H. Iwasaki, M. Okumura, C. Iwata, H. Mikamiyama and T. Tanaka, J. Org. Chem., 2003, 68, 5909–5916 CrossRef CAS PubMed
.
-
(a) W. H. Sikorski and H. J. Reich, J. Am. Chem. Soc., 2001, 123, 6527–6535 CrossRef CAS PubMed
;
(b) M. R. Binns and R. K. Haynes, J. Org. Chem., 1981, 46, 3790–3795 CrossRef CAS
;
(c) M. R. Binns, R. K. Haynes, T. L. Houston and W. R. Jackson, Tetrahedron Lett., 1980, 21, 573–576 CrossRef CAS
;
(d) P. R. Carlier, C. W.-S. Lo, M. M.-C. Lo, N. C. Wan and I. D. Williams, Org. Lett., 2000, 2, 2443–2445 CrossRef CAS PubMed
;
(e) X. Ji, J. Li, M. Wu and S. Cao, ACS Omega, 2018, 3, 10099–10106 CrossRef CAS PubMed
.
- For selected examples, see:
(a) M. H. Keylor, B. S. Matsuura and C. R. J. Stephenson, Chem. Rev., 2015, 115, 8976–9027 CrossRef CAS PubMed
;
(b) C. Zhong, G. Zhu, J. Chang and X. Sun, Tetrahedron Lett., 2011, 52, 2815–2817 CrossRef CAS
;
(c) S. Faiz, M. Yousaf, A. F. Zahoor, S. A. R. Naqvi, A. Irfan and Z. Zaman, Synth. Commun., 2017, 47, 1121–1135 CrossRef CAS
;
(d) M.-L. Tang, P. Peng, Z.-Y. Liu, J. Jiang, J.-M. Yu and X. Sun, Chem. – Eur. J., 2016, 22, 14535–14539 CrossRef PubMed
.
|
This journal is © The Royal Society of Chemistry 2024 |
Click here to see how this site uses Cookies. View our privacy policy here.