DOI:
10.1039/D4PY00340C
(Paper)
Polym. Chem., 2024,
15, 2862-2872
Radical polymerisation and thiol–ene post-polymerisation functionalisation of terpenoid acrylates in conventional and renewably sourced organic solvents†
Received
28th March 2024
, Accepted 20th June 2024
First published on 25th June 2024
Abstract
Geranyl acrylate has been synthesized and polymerized by various radical polymerisation techniques. Due to the structural diversity, a number of post polymerisation functionalization options were explored. Monomer conversion, polymer structure and the molecular weight were assessed by NMR, FT-IR and size exclusion chromatography (SEC). Free radical polymerization and Cu-mediated reversible deactivation radical polymerization (RDRP) were used to synthesise poly(geranyl acrylate) of various molecular weight in both conventional petro-derived organic and bio-based renewably sourced solvents. Cu0 wire-mediated RDRP was found to be a suitable polymerisation method when compared to CuII-mediated photoinduced RDRP giving the optimal living radical polymerisation method for the synthesis of poly(geranyl acrylate) in Cyrene (Mn,
SEC = 3000, ĐSEC = 1.25). The residual allylic double bonds provided a useful option for post-polymerization modification. Poly(geranyl acrylate) was subjected to photoinduced thiol–ene reactions, using different thiols with a variety of functional groups. The success of the functionalization reaction was evaluated by NMR, FT-IR spectroscopy, and SEC. In addition, differential scanning calorimetry was used to investigate changes in the thermal properties of the polymer, before and after thiol–ene functionalization.
Introduction
Polymer design has greatly advanced over the past few decades, enabling the synthesis and development of adaptable materials that led to improvements in quality of life.1 Polyacrylates are polymers that are widely used and display many useful qualities, including desirable mechanical and thermal properties, and are used in a wide range of applications.2 Since 1950, 8.3 billion metric tonnes of polymers have been manufactured, the majority of which were made with monomers derived from petroleum-based feedstocks.1 This contributes to both the depletion of fossil fuel resources and to an increase in greenhouse gas emissions.2 Long-term limitations on crude oil supplies have prompted the desire for more sustainable, alternative resources that could serve as the foundation for a variety of novel materials and potentially open up new applications.3 In order to minimise their environmental footprint, significant investments have been made in an attempt to find competitive polyacrylates made either entirely, or partially, from bio-based renewable chemicals.
According to earlier research, a promising method for producing bio-based acrylic monomers and polymers relies on using widely available and sustainable materials such as lignin, terpenes, terpenoids, and lactates.2 Terpenes and terpenoids are a family of hydrocarbon-rich molecules that are generated in large quantities by numerous trees and plants, such as conifers and other pine trees. These compounds also exhibit a wide structural variability and a highly functional character.4 Thus, research into uses of terpenes and terpenoids has grown in popularity.5,6 Isoprene, α- and β-pinenes, as well as D-limonene are the terpenes most frequently found in wood and citrus wastes.3 The transformation of terpenes into acrylates and the incorporation of these compounds into polymer structures has already been the focus of substantial research. For instance, Sainz et al. synthesised a series of acrylates and methacrylates through a two-step process that relied on the conversion of the terpenes to alcohols, followed by their esterification. In this way, novel poly(meth)acrylates were used in advanced renewable polymer coatings.7 Terpenoids have been employed in a variety of applications such as in the food and fragrance industries, where the most widely used terpenoids are L-menthol, geraniol, and β-citronellol.3 Compared to terpenes, relatively little research has been conducted on the radical polymerization of (meth)acrylates obtained from terpenoids, particularly those that contain unsaturated double bonds in their the side-chain, such as citronellol and geraniol, which have the potential to complicate the polymerization reaction due to radical addition. In 2019, Billon and co-workers reported that tetrahydrogeraniol (THG) had the potential to become a bio-based acrylate that could be used to form triblock copolymers using RAFT polymerization.8 Indeed, THG displays an absence of double bonds which might otherwise lead to uncontrolled branching, chain termination or cross-linking. However, this eliminates the possibility of post-polymerization modification. Worzakowska et al. used UV-photoinitiated polymerization to synthesise poly(geranyl acrylate) and poly(citronellyl acrylate) from the naturally-occurring terpenoids citronellol and geraniol, respectively, as well as methacrylate equivalents.9–11 Worzakowska also reported the synthesis of more advanced polymer structures, in the form of starch-graft-poly(neryl acrylate) and starch-graft-poly(geranyl acrylate).12 These examples demonstrate the potential of terpenoid-based acrylic analogues for industrial purposes. Furthermore, the additional unsaturated double bond(s) found in acrylate and methacrylate derivatives of both citronellol and geraniol offer a pathway for post-polymerization modification.3
Although significant progress has been achieved in the identification and synthesis of various monomers containing side-chain unsaturated double bonds, challenges remain in order to achieve successful polymerization by radical polymerization. Molina-Gutiérrez et al., for example, investigated the use of eugenol as a possible bio-based component for polymer functionalization. Derivatives of eugenol such as ethoxy dihydroeugenyl methacrylate (EDMA), ethoxy eugenol methacrylate (EEMA), and ethoxy isoeugenol methacrylate (EIMA) were successfully polymerized via free radical polymerization. Experiments involving monomers with allylic double bonds (EEMA and EIMA) displayed lower monomer conversions (EEMA, 84% and EIMA, 89%) compared to the one without, (EDMA, 98%). This behaviour was thought to arise due to the allylic radical becoming firmly stabilised by resonance as a result of allylic proton abstraction, thereby reducing the rate of polymerization.13
Post-polymerisation modifications can provide straight-forward pathways through which new polymer structures can be obtained.3 Thiol–ene chemistry has been widely used for the post-polymerization modifications of polymers thanks to high conversion, short reaction times and the ready availability of an extensive library of thiols.14 This method of polymer modification has been used effectively to form a variety of functional materials that have been widely reported on in the literature, particularly, 1,2-polybutadiene and its derivatives. For instance, Justynska et al. successfully applied thiol–ene chemistry to 1,2-poly(butadiene-block-poly(ethylene oxide)) copolymers by making use of a number of thiols with various functional groups, and reacting them with the vinyl double bonds of the copolymers.15 In 2017, Li et al. employed the same reaction to functionalize diene-based elastomers using 3-methylenehepta-1,6-diene, a derivative of butadiene.16
We have found no studies reporting on the free or living radical polymerization of terpenoid-based acrylate homopolymers containing backbone unsaturated double bonds, and their subsequent thiol–ene post-polymerization modification. The goal of this work was to examine the feasibility of minimising branching and crosslinking when polymerising geranyl acrylate, and eventually replacing petro-derived solvents with Cyrene, a bio-based solvent, in order to increase overall sustainability. The effects of solvent, reaction temperature, monomer concentration, catalyst amounts, and the nature of the ligand were studied. Subsequently, a thiol–ene reaction was carried out on the synthesized polymer with the help of a range of thiols containing various functional groups. The thermal properties of the polymers, before and after post-polymerization modification, were also investigated.
Results and discussion
Geranyl acrylate synthesis
Geraniol was the terpenoid chosen for this study, Scheme 1. Terpenoids can be converted into acrylates via a one-step reaction of the hydroxyl group. This results in a variety of distinctive, bio-based replacements for petrochemical-based monomers, that can potentially meet the requirements of various polymerisation techniques. The esterification of geraniol with acryloyl chloride in the presence of triethylamine is an efficient and well-established method for the synthesis of terpenoid acrylates, Scheme 1. It is noted that this is not a particularly sustainable method and reaction of the acid would be more suitable, however this method was used for convenience. Purified geranyl acrylate was characterized by NMR, FT-IR, and LC-MS, Fig. S1–S6, ESI.† The disappearance of the proton signal (R–OH) at 2.25 ppm and the appearance of vinyl peaks at 5.80, 6.13, and 6.37 ppm in the 1H NMR spectrum of the product was evidence of the successful formation of geranyl acrylate, Fig. 1. Furthermore, the absence of a weak and broad alcohol band at 3300 cm−1, as well as the presence of strong ester peaks at 1725 cm−1 (νC
O) and 1178 cm−1 (νC–O) in the FT-IR spectrum of the product suggested successful esterification, Fig. S7, ESI,† with a molecular weight m/z = 208.3 g mol−1 LC-MS.3 While it is expected to be more challenging to polymerize geranyl acrylate due to the presence of two side-chain alkenes, they may also prove amenable for post-polymerization modification by thiol–ene reaction. Furthermore, geranyl acrylate was synthesised from a natural renewable terpenoid. We decided it would be worthwhile to try both free and living radical polymerisation pathways to investigate the performance of each.
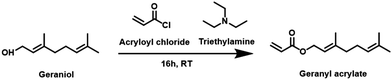 |
| Scheme 1 Synthesis of geranyl acrylate through the esterification of geraniol with acryloyl chloride in the presence of triethylamine. | |
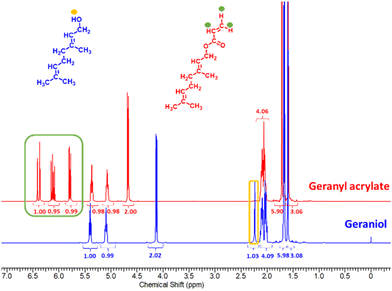 |
| Fig. 1
1H NMR (400 MHz, CDCl3) spectra of geraniol and geranyl acrylate. | |
Free radical polymerisation of geranyl acrylate in conventional organic and bio-based solvents
Free radical polymerisation (FRP) of geranyl acrylate was carried out in cyclohexane using the thermal initiator dimethyl 2,2′-azobis(2-methylpropionate) (V-601), Scheme 2. The reaction was optimised by adjusting two parameters: the weight ratio of monomer to solvent and the concentration of initiator, Table 1. At equal amounts of V-601 (1.0 mol%), gelation occurred at 30 wt% monomer to solvent, resulting in a polymer insoluble in all solvents. Compared to the polymer obtained using a 10 wt% monomer to solvent ratio (Mn,
SEC = 12
000 g mol−1), a 20 wt% ratio resulted in a higher molecular weight polymer (Mn,
SEC = 17
000 g mol−1) but with no evidence of gelation. By increasing the monomer to solvent ratio, the monomer concentration in the system increased, and as termination is diffusion-controlled, this process slowed down substantially. This, increases chain transfer to polymer, resulting in branching and subsequent gelation. It is probable that once most of the monomer units were consumed, the presence of the side-chain unsaturated double bond made it even easier for branching to occur, hastening gelation.17 Keeping a 20 wt% monomer to solvent ratio, the concentration of initiator was subsequently optimized. While increasing the concentration of V-601 initiator from 1.0 mol% to 2.0 mol% led to a substantial increase in the rate of monomer conversion, it also led to the formation of higher dispersity polymers, Table 1. To be noted, no conversion of the terpenoid double bonds was observed following polymerisation as evidenced by 1H NMR spectroscopy, Fig. S9, ESI.† As a result, 1.5 mol% was chosen as the optimal concentration of V-601 initiator for the FRP in cyclohexane, where acceptable monomer conversion, polymer molecular weight and dispersity were obtained. Monomer conversion was monitored by 1H NMR, by calculating the ratio between the integrated area of the vinyl end group peaks (δ = 6.4, 6.1, and 5.8 ppm) and that of the corresponding proton signal on the repeating unit of the polymer (δ = 4.5 ppm). Subsequently, keeping all other reaction conditions constant, cyclohexane was replaced by bio-based alternatives to improve the overall sustainability of the polymerization. Furthermore, the effects of different solvents on the free radical polymerization of geranyl acrylate were investigated.
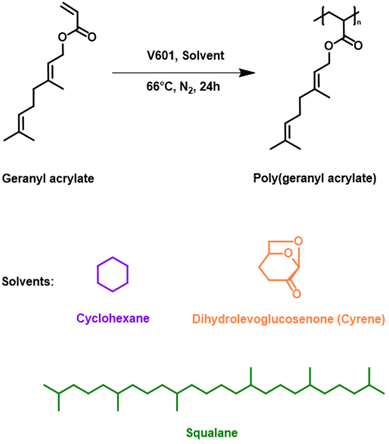 |
| Scheme 2 Reaction scheme of the FRP of geranyl acrylate in three solvents. | |
Table 1 Monomer and initiator concentrations, conversiona and molecular weightb data for poly(geranyl acrylate) synthesised by FRP in various solvents
Obtained via1H NMR in CDCl3.
M
n, and Mw obtained via SEC, RI detection in THF, pMMA standards.
|
|
In this study, squalane and dihydrolevoglucosenone (Cyrene) were used as plant-based, renewable substitutes for petrochemically-derived cyclohexane, Scheme 2.2 Squalane is produced via hydrogenation of the natural product squalene. Furthermore, the lack of unsaturated double bonds in squalane makes it an excellent hydrophobic solvent for use in FRP18 as a replacement for cyclohexane or toluene. Conversely, Cyrene is bio-based alternative for dipolar aprotic solvents (i.e., dimethylformamide (DMF) or N-methyl-2-pyrrolidone (NMP)). It is mainly sourced from cellulose in a two-step process and is miscible with various organic solvents.19 Under identical conditions, the FRP of geranyl acrylate in Cyrene led to the formation of a polymer with higher monomer conversion and molecular weight, but also broader dispersity (conv. = 58%, Mn,
SEC = 16
000 g mol−1, ĐSEC = 3.25) when compared to cyclohexane (conv. = 43%, Mn,
SEC = 14
000 g mol−1, ĐSEC = 1.87), the former being more viscous than the latter, Fig. 2 and Table 1. The viscosity of the solvent and its interactions with the monomer and polymer can significantly affect free radical polymerisation processes. It is thought that these properties were partially responsible for the differences observed in monomer conversion and the Mn of poly(geranyl acrylate) synthesised in these solvents. In FRP, chain termination usually occurs through bimolecular combination or disproportionation reactions. These reactions are diffusion-controlled, so that the rate constant of termination (kt) is inversely proportional to the viscosity of the system. Consequently, viscous media are expected to give rise to the formation of high molecular weight polymers.17 Conversely, the same reaction carried out in squalane, which is also more viscous than cyclohexane, resulted in a polymer with significantly lower monomer conversion and molecular weight, although with narrower dispersity (conv. = 21%, Mn,
SEC = 6000 g mol−1, ĐSEC = 1.27). This observation could also be the result of a number of different solvent effects. One of them is the impact of the solubility of monomer and polymer in the solvent, which can affect the rate constant of propagation kp.20 It is also possible that the growing polymer radicals are able to form a complex with solvent molecules, the stability of which could influence kp and result in a polymer of lower molecular weight.21 According to previous studies, the rate of FRP can be significantly affected by the degree of unsaturation of the monomer.
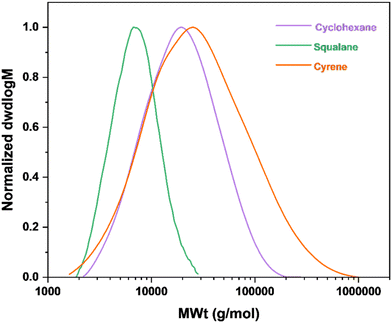 |
| Fig. 2 SEC curves for poly(geranyl acrylate) synthesized by FRP in various solvents, 20 wt% monomer to solvent, and 1.5 mol% V-601. | |
As the polymerization progresses, chain propagation competes with chain transfer events. Indeed, abstraction of the allylic hydrogens on the side-chain of geranyl acrylate leads to the formation of macroradicals that are ineffective at initiating new chains or propagating much further.22 Next, the effects of unsaturation on the synthesis of poly(geranyl acrylate) by copper-mediated living radical polymerisation methods were investigated in both organic and renewable solvents.
Cu0 wire-mediated RDRP of geranyl acrylate in organic and bio-based solvents
Cu0 wire-mediated RDRP is a living radical polymerisation method, which can provide control over molecular weight and dispersity. As was seen in the previous section, the two unsaturated double bonds in the side-chain of geranyl acrylate had a detrimental effect on the rate of polymerization and made polymerization challenging due to chain transfer. In order to maximise both control and monomer conversion, consideration was given to the choice of solvent selected to carry out the Cu0 wire-mediated RDRP of geranyl acrylate.23 Other parameters, such as the reaction temperature and the ratio of catalyst to ligand are known the influence the molecular weight and dispersity.
For the Cu0 wire-mediated RDRP of geranyl acrylate, a target of DPn = 50 was chosen, 5 cm of pre-activated copper wire (Cu0) was used, CuBr2 acted as the copper source, tris[2-(dimethylamino)ethyl]amine (Me6TREN) was used as the ligand and ethyl α-bromoisobutyrate (EBiB) as the initiator, Scheme 3. Taking into account previous work by Whitfield et al.,24 the reaction was carried out at ambient temperature under universal conditions: [EBiB]
:
[Me6TREN]
:
[CuBr2] = 1
:
0.18
:
0.05. A monomer to solvent volume ratio of 1 was used with seven common organic solvents, or solvent mixtures, covering a range of relative polarities25 (TFE, IPA, ACN, DMF, NMP, toluene
:
MeOH (4
:
1) and toluene
:
IPA (4
:
1)), Table 2. The synthesis of poly(geranyl acrylate) in TFE yielded a polymer with the narrowest dispersity (ĐSEC = 1.24) but also the lowest molecular weight (Mn,
SEC = 2300 g mol−1), Fig. 3 and Table 2. In pure solvents, polymer dispersity increased slightly with a decrease in relative polarity. As solvents with low relative polarity, such as toluene, could not readily dissolve the copper complex, mixtures of toluene and MeOH or IPA were used. Poly(geranyl acrylate) obtained in the toluene–MeOH mixture exhibited higher monomer conversion and molecular weight, but also broader dispersity (conv. = 65%, Mn,
SEC = 8100 g mol−1, ĐSEC = 3.34) than the polymer produced in the toluene–IPA mixture (conv. = 46%, Mn,
SEC = 4300 g mol−1, ĐSEC = 1.58). However, Cu0 wire-mediated RDRP is a radical process and, as such, chain transfer is unavoidable. This is especially true in this case, with two unsaturated double bonds present on the side chain of the monomer. An indication of this is the tendency of polymer dispersity to increase with increasing monomer conversion. In addition, it is possible that in certain cases, such as with acetonitrile, the solvent is able to better stabilise the CuBr species, which would increase the rate of polymerisation and result in a loss of control.26
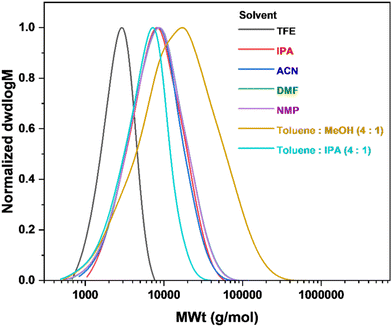 |
| Fig. 3 SEC curves for poly(geranyl acrylate) synthesized in different solvents and solvent mixtures. RI detection in THF with pMMA standards. Conditions: [GA] : [EBiB] : [Me6TREN] : [CuBr2] = 50 : 1 : 0.18 : 0.05, Vsolvent = 50% v/v to monomer, 24 h at RT. | |
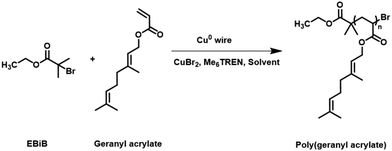 |
| Scheme 3 Reaction scheme for the Cu0 wire-mediated RDRP of geranyl acrylate. | |
Table 2 Conversiona and molecular weightb data for poly(geranyl acrylate) (DPn = 50) synthesized by Cu0 wire-mediated RDRPc in different solvents25
Solvent |
Relative polarity |
ConversionNMR (%) |
M
n, theoretical (g mol−1) |
M
n, SEC (g mol−1) |
M
w, SEC (g mol−1) |
Đ
|
Obtained via1H NMR in CDCl3.
M
n, and Mw obtained via SEC, RI detection in THF, pMMA standards.
[GA] : [EBiB] : [Me6TREN] : [CuBr2] = 50 : 1 : 0.18 : 0.05, Vsolvent = 50% v/v to monomer, 24 h, RT.
|
TFE |
0.90 |
21 |
2300 |
2300 |
2800 |
1.24 |
IPA |
0.54 |
52 |
5600 |
5900 |
10 000 |
1.71 |
ACN |
0.46 |
43 |
4600 |
5700 |
9700 |
1.72 |
DMF |
0.39 |
56 |
6000 |
5700 |
11 000 |
1.86 |
NMP |
0.35 |
62 |
6700 |
5700 |
10 000 |
1.86 |
Toluene : IPA (4 : 1) |
0.10/0.54 |
46 |
4900 |
4300 |
6700 |
1.58 |
Toluene : MeOH (4 : 1) |
0.10/0.76 |
65 |
7000 |
8100 |
27 000 |
3.34 |
The relative polarity of the solvent is a key reaction parameter for solubilising reagents but also as it influences the rate and extent of disproportionation of CuI. Furthermore, it was proposed that, in the presence of a suitable polar solvent and a nitrogen-containing ligand (e.g., Me6TREN), the CuIBr species formed in the initiation step can “instantly” disproportionate into the extremely active Cu0 species and CuBr2.27 As a result, it was important to investigate the relative polarity of Cyrene before using it as a substitute for conventional organic solvents. According to the literature, the relative polarity of Cyrene (0.333) is similar to that of DMF (0.386) and NMP (0.355).28 In subsequent experiments, a mixture of Cyrene and TFE was used, with the volume ratio of TFE decreasing (from 1
:
4 to 4
:
1 Cyrene
:
TFE) in order to improve the overall renewability of the reaction.29 It was found that increasing the proportion of Cyrene in the mixture improved both the molecular weight control and dispersity of the polymer, Fig. 4 and Table 3. Moreover, the only difference observed between pure Cyrene (Mn,
SEC = 4400 g mol−1, ĐSEC = 1.47) and the 4
:
1 mixture of Cyrene
:
TFE (Mn,
SEC = 4400 g mol−1, ĐSEC = 1.47) was a slight increase in Mw, which in turn increased dispersity. While substituting organic solvents with Cyrene resulted in overall slightly lower molecular weights, control over the polymerization remained. Cyrene was therefore deemed a satisfactory bio-based alternative to conventional organic solvents in the Cu0-wire meditated RDRP of geranyl acrylate.
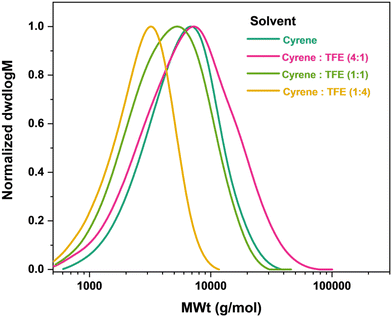 |
| Fig. 4 SEC curves for poly(geranyl acrylate) synthesized in Cyrene and mixtures with TFE. Conditions: [GA] : [EBiB] : [Me6TREN] : [CuBr2] = 50 : 1 : 0.18 : 0.05, Vsolvent = 50% v/v with respect to monomer, 24 h at RT. | |
Table 3 Conversiona and molecular weightb data for poly(geranyl acrylate) (DPn = 50) synthesized by Cu(0) wire-mediated RDRPc in Cyrene and mixtures with TFE
Solvent |
ConversionNMR (%) |
M
n, theoretical (g mol−1) |
M
n, SEC (g mol−1) |
M
w, SEC (g mol−1) |
Đ
|
Obtained via1H NMR in CDCl3.
M
n, and Mw obtained via SEC, RI detection in THF, pMMA standards.
Polymerisation conditions: [GA] : [EBiB] : [Me6TREN] : [CuBr2] = 50 : 1 : 0.18 : 0.05, Vsolvent = 50% v/v to monomer, 24 h at RT.
|
Cyrene : TFE (1 : 4) |
19 |
2000 |
2200 |
3100 |
1.39 |
Cyrene : TFE (1 : 1) |
30 |
3200 |
3300 |
5600 |
1.69 |
Cyrene : TFE (4 : 1) |
47 |
5000 |
4400 |
9000 |
2.03 |
Cyrene |
42 |
4500 |
4400 |
7000 |
1.47 |
In an attempt to improve control over the polymerisation in Cyrene the conditions were optimised based on three factors: the monomer to solvent ratio, the amounts of ligand and CuII, and the reaction temperature. Even though the monomer and copper complex were soluble in the solvent, Cyrene is intrinsically more viscous than the other organic solvents. Therefore, the monomer to solvent ratio was varied in order to investigate potential effects on the polymerization. Increasing it from 1
:
1 to 1
:
3 decreased monomer conversion from 42% to 28%, Fig. 5 and Table 4. No beneficial effects were observed from decreasing the amount of monomer relative to solvent. Thus, the ratio was kept at 1
:
1. Conversely, changing the [EBiB]
:
[Me6TREN]
:
[CuBr2] ratio from 1
:
0.18
:
0.05 to 1
:
0.54
:
0.15 increased both monomer conversion, which reached 53%, and molecular weight, Fig. 6 and Table 5. However, this came at the cost of slightly increased dispersity due to the disturbed active/dormant equilibrium, and reduced control, exhibited by the difference between theoretical and experiment Mn values.
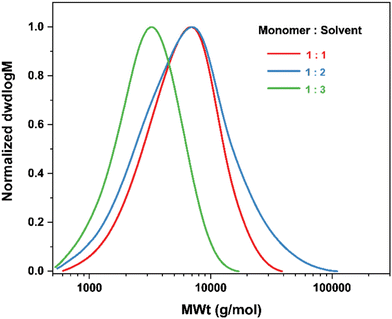 |
| Fig. 5 SEC curves for poly(geranyl acrylate) synthesized in Cyrene at different monomer to solvent ratios. RI detection in THF using pMMA standards. Conditions: [GA] : [EBiB] : [Me6TREN] : [CuBr2] = 50 : 1 : 0.18 : 0.05, 24 h at RT. | |
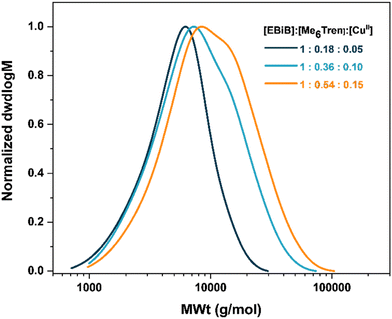 |
| Fig. 6 SEC curves for poly(geranyl acrylate) synthesized in Cyrene at different ligand : catalyst concentrations. RI detection in THF using pMMA standards. Conditions: VCyrene = 50% v/v to monomer, 24 h at RT. | |
Table 4 Conversiona and molecular weightb data for poly(geranyl acrylate) (DPn = 50) by Cu0 wire-mediated RDRPc in Cyrene, at different monomer concentrations
Monomer to solvent |
ConversionNMR (%) |
M
n, theoretical (g mol−1) |
M
n, SEC (g mol−1) |
M
w, SEC (g mol−1) |
Đ
|
Obtained via1H NMR in CDCl3.
M
n, and Mw obtained via SEC, RI detection in THF, pMMA standards.
Polymerisation conditions: [GA] : [EBiB] : [Me6TREN] : [CuBr2] = 50 : 1 : 0.18 : 0.05, 24 h at RT.
|
1 : 1 |
42 |
4500 |
4400 |
7000 |
1.47 |
1 : 2 |
39 |
4000 |
4300 |
8900 |
2.08 |
1 : 3 |
28 |
3000 |
2500 |
3600 |
1.43 |
Table 5 Conversiona and molecular weightb data for poly(geranyl acrylate) (DPn = 50) synthesised by Cu0 wire-mediated RDRPc in Cyrene, at different ligand
:
catalyst ratios
[EBiB] : [Me6TREN] : [CuII] |
ConversionNMR (%) |
M
n, theoretical (g mol−1) |
M
n, SEC (g mol−1) |
M
w, SEC (g mol−1) |
Đ
|
Obtained via1H NMR in CDCl3.
M
n, and Mw obtained via SEC, RI detection in THF, pMMA standards.
Polymerisation conditions: Vsolvent = 50% v/v to monomer, 24 h at RT.
|
[1] : [0.18] : [0.05] |
42 |
4500 |
4400 |
7000 |
1.47 |
[1] : [0.36] : [0.10] |
49 |
5300 |
5700 |
10 000 |
1.78 |
[1] : [0.54] : [0.15] |
53 |
5700 |
7000 |
13 000 |
1.87 |
Subsequently, the effects of reaction temperature were investigated. Overall, an increase in the reaction temperature led to a decrease in dispersity, although monomer conversion was lower than at ambient temperature. Indeed, at 70 °C, a monomer conversion of only 28% was achieved, with ĐSEC = 1.25, Fig. 7 and Table 6. Furthermore, good agreement between theoretical and experimental Mn values was obtained. However, a significant increase in dispersity at 80 °C was evidence of a loss of control and exhibited a limit above which the reaction should not be carried out. Furthermore, first order kinetics were confirmed in the Cu0 wire-mediated RDRP of geranyl acrylate at ambient temperature through the observation of the linear behaviour of percentage conversion as a function of time, Fig. S15, S16 and Table S1, ESI.†
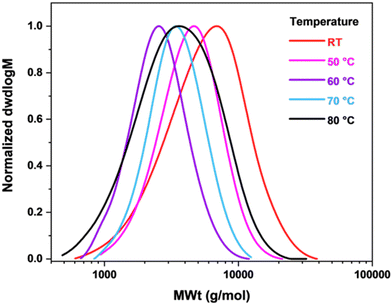 |
| Fig. 7 SEC curves for poly(geranyl acrylate) synthesized in Cyrene at different temperatures. Conditions: [GA] : [EBiB] : [Me6TREN] : [CuBr2] = 50 : 1 : 0.18 : 0.05, VCyrene = 50% v/v to monomer, 24 h. | |
Table 6 Conversiona and molecular weightb data for poly(geranyl acrylate) (DPn = 50) synthesised by Cu0 wire-mediated RDRPc in Cyrene, at different temperatures
Temperature (°C) |
ConversionNMR (%) |
M
n, theoretical (g mol−1) |
M
n, SEC (g mol−1) |
M
w, SEC (g mol−1) |
Đ
|
Obtained via1H NMR in CDCl3.
M
n, and Mw obtained via SEC, RI detection in THF, pMMA standards.
Polymerisation conditions: [GA] : [EBiB] : [Me6TREN] : [CuBr2] = 50 : 1 : 0.18 : 0.05, 24 h.
|
Room temperature |
42 |
4500 |
4400 |
7000 |
1.47 |
50 |
30 |
3200 |
3700 |
4900 |
1.31 |
60 |
26 |
2800 |
2200 |
2800 |
1.25 |
70 |
28 |
3000 |
3000 |
3800 |
1.25 |
80 |
28 |
3000 |
2700 |
4300 |
1.58 |
CuII-mediated photoinduced RDRP of geranyl acrylate in organic and bio-based solvents
The CuII-mediated photoinduced RDRP of geranyl acrylate was performed in both IPA and TFE, Scheme 4. This method required lower concentrations of CuBr2 and the tertiary amine ligand (Me6TREN)30 to obtain monomer conversions similar to those obtained by Cu0 wire-mediated RDRP. Indeed, the ratio of [EBiB]
:
[Me6TREN]
:
[CuBr2] was 1
:
0.12
:
0.02 in photoinduced RDRP compared to 1
:
0.18
:
0.05 in Cu0 wire-mediated RDRP. Furthermore, compared to Cu0 wire-mediated (Mn,
SEC = 5900 g mol−1, ĐSEC = 1.71), photoinduced RDRP in IPA resulted in a polymer of broadly equal percentage monmer conversion (52% and 50%, respectively) with similar molecular weight (Mn,
SEC = 4500 g mol−1) and higher dispersity (ĐSEC = 1.96), Fig. 3, 8 and Tables 2, 7. A similar trend was observed when the reaction was carried out in TFE, with similar monomer conversion in both cases and a higher dispersity in TFE. Cyrene was subsequently used to replace TFE and IPA. In this case, poly(geranyl acrylate) synthesized by CuII-mediated photoinduced RDRP exhibited both lower monomer conversion and molecular weight, as well as higher dispersity (30% conversion, Mn,
SEC = 3600 g mol−1, ĐSEC = 1.84), Table 7, compared to that obtained by Cu0 wire RDRP (42% conversion, Mn,
SEC = 4400 g mol−1, ĐSEC = 1.47), Table 6.
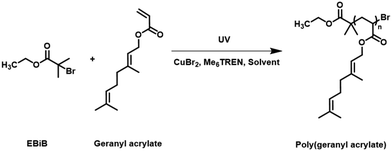 |
| Scheme 4 Reaction scheme for the CuII-mediated photoinduced RDRP of geranyl acrylate in IPA, TFE and Cyrene. | |
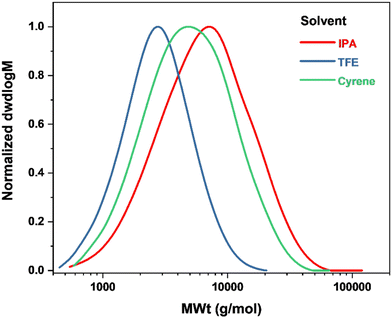 |
| Fig. 8 SEC curves of poly(geranyl acrylate) synthesized by CuII-mediated photoinduced RDRP in different solvents. Conditions: [GA] : [EBiB] : [Me6TREN] : [CuBr2] = 50 : 1 : 0.12 : 0.02, Vsolvent = 50% v/v to monomer, 24 h at RT. | |
Table 7 Conversiona and molecular weightb data for poly(geranyl acrylate) (DPn = 50) synthesised by CuII-mediated photoinduced RDRPc in three solvents
Solvent |
ConversionNMR (%) |
M
n, theoretical (g mol−1) |
M
n, SEC (g mol−1) |
M
w, SEC (g mol−1) |
Đ
|
Obtained via1H NMR in CDCl3.
M
n, and Mw obtained via SEC, RI detection, in THF, pMMA standards.
Polymerisation conditions: [GA] : [EBiB] : [Me6TREN] : [CuBr2] = 50 : 1 : 0.12 : 0.02, Vsolvent = 50% v/v to monomer, 24 h at RT.
|
IPA |
50 |
5400 |
4500 |
8900 |
1.96 |
TFE |
21 |
2200 |
2200 |
3300 |
1.48 |
Cyrene |
30 |
3200 |
3600 |
6600 |
1.84 |
Although CuII-mediated photoinduced RDRP achieved similar monomer conversions with lower concentrations of CuBr2 and Me6TREN, overall, Cu0 wire-mediated RDRP led to the formation of polymers with higher molecular weight and narrower dispersity. In addition, the Cu0 wire method was more versatile, as evidenced by its ability to maintain control over the polymerization in solvents covering a wider range of relative polarities, from TFE to Cyrene. Therefore, thanks to its versatility and the control it provided, Cu0 wire mediated-RDRP was deemed the optimal, living polymerisation method for the controlled synthesis of poly(geranyl acrylate) in all solvents that were tested.
Functionalization of poly(geranyl acrylate) by thiol–ene chemistry
Following polymerization, the residual side-chain allylic double bonds present on the repeating units of poly(geranyl acrylate) were targeted for functionalization. In earlier research, Uygun, Tasdelen, and Yagci conducted a comparative analysis of the type of initiation used in thiol–ene reactions, which included a variety of polymers and thiols.31 They showed that the majority of radical thiol–ene reactions were most effectively achieved via photoinitiation rather than thermal initiation, with 2,2-dimethoxy-2-phenyl acetophenone (DMPA) being most efficacious.32 Similar results were obtained by Cui and co-workers, in which a 3-methylenehepta-1,6-diene copolymer, and the relevant thiol, were involved in a photoinitiated thiol–ene reaction.16 Full conversion was achieved in <2 hours, whereas the degree of functionalization obtained in an overnight, thermally-initiated pathway was <80%.
A photoinitiated thiol–ene reaction was carried out, Scheme 5, with a 10-fold excess of methyl thioglycolate and DMPA as photoinitiator. The reaction proceeded overnight in toluene at λ = 365 nm.
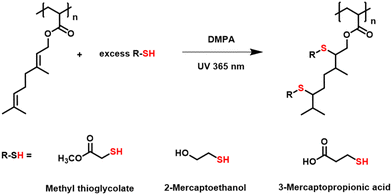 |
| Scheme 5 Reaction scheme for the functionalization of poly(geranyl acrylate) with various thiols, through a photoinitiated thiol–ene reaction. | |
The success of the reaction was observed, in part, by 1H NMR analysis. Indeed, full conversion of the side-chain allylic groups was shown by the loss of the corresponding peaks, originally at δ = 5.08 and 5.32 ppm, Fig. 9. This analysis also confirmed that no detectable cyclic units were formed. The peaks associated with the newly formed functional group (–CH2–S–CH(CH(CH3)CR)–) were observed in the range of 2.14 to 3.20 ppm. Furthermore, the degree of functionalization was derived from the integrals of signals HA (–COOCH2–) and HB (–COOCH3) of the functionalized polymer following purification, Fig. 11 and Table 8. In the IR spectrum, the loss of the weak alkene signal at 1670 cm−1 (νC
C) was further evidence of successful functionalization, Fig. 10. In addition, the molecular weight increased from Mn,
SEC = 19 kg mol−1 to Mn,
SEC = 36 kg mol−1 following the reaction, with monomodal distribution and no sign of shoulder peaks, thus signalling the absence of side reactions, such as interchain coupling, Fig. 12 and Table 8.
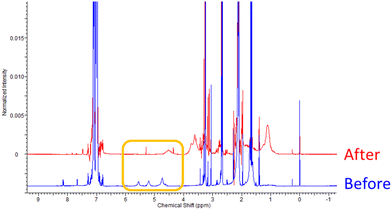 |
| Fig. 9
1H NMR (400 MHz, toluene-d8) spectra of poly(geranyl acrylate), before and after functionalization with methyl thioglycolate. | |
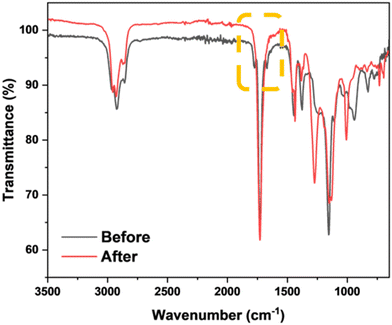 |
| Fig. 10 FT-IR spectra of poly(geranyl acrylate) before and after functionalization with methyl thioglycolate. Highlighted is the loss of the alkene signal at 1670 cm−1. | |
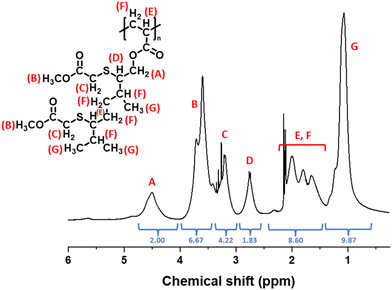 |
| Fig. 11
1H NMR (400 MHz, toluene-d8) spectrum of methyl thioglycolate-functionalised poly(geranyl acrylate). | |
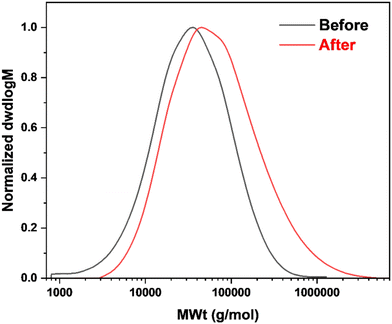 |
| Fig. 12 SEC curves of poly(geranyl acrylate), before and after functionalization with methyl thioglycolate. RI detection in THF using pMMA standards. | |
Table 8 Conversiona, thermal analysisb, and molecular weightc of poly(geranyl acrylate) before and after functionalization with different thiols
Thiol–ene |
Abbreviation |
DFa (%) |
T
g (°C) |
T
m (°C) |
M
n, SEC (g mol−1) |
M
w, SEC (g mol−1) |
Đ
|
Degree of functionalization (DF) (%) of the side-chain double bonds, obtained via1H NMR.
T
m and Tg obtained by DSC analysis under N2, from −100 to 220 °C at 10 K min−1 in aluminium pans.
M
n and Mw obtained via SEC, RI detection in THF (MT, ME) and DMF (MPA), pMMA standards.
|
Poly(geranyl acrylate) |
p(GA) |
N/A |
−58 |
N/A |
19 000 |
56 000 |
2.94 |
Methyl thioglycolate |
MT |
100 |
−24 |
N/A |
36 000 |
133 000 |
3.74 |
2-Mercaptoethanol |
ME |
100 |
−52 |
97 |
27 000 |
50 000 |
1.88 |
3-Mercaptopropionic acid |
MPA |
100 |
−67 |
N/A |
46 000 |
80 000 |
1.73 |
In addition to methyl thioglycolate, functionalization was assessed by reacting poly(geranyl acrylate) with thiols bearing different functional groups, 2-mercaptoethanol (hydroxyl), and 3-mercaptopropionic acid (carboxylic acid). In all cases, poly(geranyl acrylate) was completely functionalized, as shown by the loss of the allylic group peaks at δ = 5.08 and 5.32 ppm, as well as the appearance of the corresponding set of peaks from the thiol moiety, Table 8 and Fig. S17, S19, S21, S23, ESI.† Additionally, the FT-IR spectrum of each product did not exhibit a νCC signal at 1670 cm−1 (νC
C), Fig. S18 and S22, ESI.† Functionalization of poly(geranyl acrylate) with various thiols also led to changes in the polarity of the polymers, as evidenced by differences in solubility. Indeed, methyl thioglycolate-functionalized poly(geranyl acrylate) was soluble in toluene, whereas polymers functionalized with 2-mercaptoethanol and 3-mercaptopropionic acid were not. However, the latter two were both soluble in THF and DMSO. Functionalization of poly(geranyl acrylate) also led to changes in the thermal properties of the polymer. Initially, pure, non-functionalized poly(geranyl acrylate) displayed a glass transition (Tg) = −58 °C, Fig. 13 and Table 8. Following the thiol–ene reaction, which caused the side-chains of the polymers to be extended, the thermal behaviour of the polymers changed based on the structure of the thiol. Indeed, after addition of MT, the Tg of the functionalized polymer increased to −24 °C. Supporting this observation, it has been reported in the literature that polar functional groups increase cohesiveness, interchain attraction, and intermolecular forces.33 The other functionalized polymers exhibited a Tg close to that of the starting material, at −52 °C and −67 °C, by reaction with ME and MPA, respectively. Unlike the other two products and the homopolymer, 2-mercaptoethanol-functionalized poly(geranyl acrylate) exhibited a melting point transition (Tm) at 97 °C. This behaviour was attributed to the presence of ordered microdomains which were formed as a result of inter-macromolecular hydrogen bonds.16
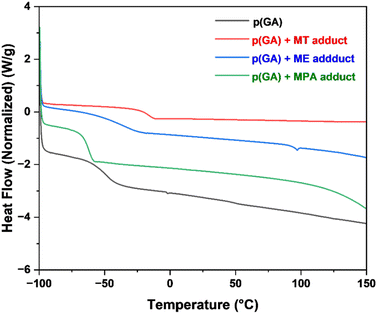 |
| Fig. 13 DSC graphs of poly(geranyl acrylate) and the products of its functionalization with methyl thioglycolate (MT), 2-mercaptoethanol (ME), and 3-mercaptopropionic acid (MPA), under N2, from −100 to 220 °C at 10 K min−1 in aluminium pans. | |
Conclusion
The monomer geranyl acrylate, largely derived from a renewable source, has been successfully synthesised by esterification of geraniol and characterized by NMR, FT-IR, and LC-MS. Poly(geranyl acrylate) was synthesized by FRP and the reaction conditions in both conventional organic and bio-based solvents were optimised. Up to 43% monomer conversion was achieved in cyclohexane, as well as 21% in squalane, and 58% in Cyrene, despite the presence of unsaturated double bonds on the side-chain of the polymer. Subsequently, the use of living polymerization techniques for the synthesis of libraries of poly(geranyl acrylate) polymers in various solvents was investigated. Both Cu0 wire-mediated and CuII-mediated photoinduced RDRP were successfully applied to the polymerization of geranyl acrylate. The effects of temperature, monomer concentration, catalyst amounts and the nature of the ligand on the Cu0 wire-mediated RDRP of geranyl acrylate were also investigated. Cu0 wire-mediated RDRP was found to be the optimal living radical polymerisation method in both organic and bio-based solvents, yielding polymers with higher molecular weight and lower dispersity compared to those obtained by CuII-mediated photoinduced RDRP. Finally, poly(geranyl acrylate) was functionalized through a photoinduced thiol–ene reaction with three different thiols, methyl thioglycolate, 2-mercaptoethanol and 3-mercaptopropionic acid. Reaction completion and successful functionalization were confirmed by 1H NMR, FT-IR and SEC. Finally, the thermal behaviour of the polymers, both before and after thiol–ene functionalization, was characterized by DSC.
Data availability
The data supporting this article has been included as part of the ESI.†
Conflicts of interest
There are no conflicts to declare.
Acknowledgements
We thank the Research Technology Platforms (RTP) of the University of Warwick and Dr Daniel Lester and James Town for providing training and equipment and EPSRC for equipment funded in part by EPSRC EP/V036211/1 and EP/V007688/1.
Notes and references
- R. M. O'Dea, J. A. Willie and T. H. Epps III, ACS Macro Lett., 2020, 9, 476–493 CrossRef PubMed
.
- C. Veith, F. Diot-Néant, S. A. Miller and F. Allais, Polym. Chem., 2020, 11, 7452–7470 RSC
.
- M. A. Droesbeke and F. E. Du Prez, ACS Sustainable Chem. Eng., 2019, 7, 11633–11639 CrossRef CAS
.
- A. L. Holmberg, N. A. Nguyen, M. G. Karavolias, K. H. Reno, R. P. Wool and T. H. Epps III, Macromolecules, 2016, 49, 1286–1295 CrossRef CAS
.
- P. A. Wilbon, F. Chu and C. Tang, Macromol. Rapid Commun., 2013, 34, 8–37 CrossRef CAS PubMed
.
- E. Oldfield and F. Y. Lin, Angew. Chem., Int. Ed., 2012, 51, 1124–1137 CrossRef CAS PubMed
.
- M. Sainz, J. Souto, D. Regentova, M. Johansson, S. Timhagen, D. J. Irvine, P. Buijsen, C. Koning, R. Stockman and S. Howdle, Polym. Chem., 2016, 7, 2882–2887 RSC
.
- S. Noppalit, A. Simula, N. Ballard, X. Callies, J. M. Asua and L. Billon, Biomacromolecules, 2019, 20, 2241–2251 CrossRef CAS PubMed
.
- M. Worzakowska and E. Torres-Garcia, Polym. Degrad. Stab., 2016, 133, 227–233 CrossRef CAS
.
- M. Worzakowska, Polymers, 2021, 13, 1659 CrossRef CAS PubMed
.
- M. Worzakowska, J. Therm. Anal. Calorim., 2017, 127, 2025–2035 CrossRef CAS
.
- M. Worzakowska, Eur. Polym. J., 2019, 110, 265–275 CrossRef CAS
.
- S. Molina-Gutiérrez, A. Manseri, V. Ladmiral, R. Bongiovanni, S. Caillol and P. Lacroix-Desmazes, Macromol. Chem. Phys., 2019, 220, 1900179 CrossRef
.
- L. Li, S. Li and D. Cui, J. Polym. Sci., Part A: Polym. Chem., 2017, 55, 1031–1039 CrossRef CAS
.
- J. Justynska and H. Schlaad, Macromol. Rapid Commun., 2004, 25, 1478–1481 CrossRef CAS
.
- L. Li, S. Li and D. Cui, Macromolecules, 2016, 49, 1242–1251 CrossRef CAS
.
- S.-i. Matsuoka, T. Kikuno, K. Takagi and M. Suzuki, Polym. J., 2010, 42, 368–374 CrossRef CAS
.
- F. Mellou, A. Varvaresou and S. Papageorgiou, Int. J. Cosmet. Sci., 2019, 41, 517–525 CrossRef CAS PubMed
.
- J. E. Camp, ChemSusChem, 2018, 11, 3048–3055 CrossRef CAS PubMed
.
-
M. Kamachi, Advances in Polymer Science, Springer, Berlin, 1981, pp. 55–87 Search PubMed
.
- G. M. Burnett, G. G. Cameron and S. N. Joiner, J. Chem. Soc., Faraday Trans. 1, 1973, 69, 322–327 RSC
.
- Z. Demchuk, O. Shevchuk, I. Tarnavchyk, V. Kirianchuk, A. Kohut, S. Voronov and A. Voronov, ACS Sustainable Chem. Eng., 2016, 4, 6974–6980 CrossRef CAS
.
- S. Harrisson, S. R. Mackenzie and D. M. Haddleton, Macromolecules, 2003, 36, 5072–5075 CrossRef CAS
.
- R. Whitfield, A. Anastasaki, V. Nikolaou, G. R. Jones, N. G. Engelis, E. H. Discekici, C. Fleischmann, J. Willenbacher, C. J. Hawker and D. M. Haddleton, J. Am. Chem. Soc., 2017, 139, 1003–1010 CrossRef CAS PubMed
.
- M. Dutkiewicz, J. Chem. Soc., Faraday Trans., 1990, 86, 2237–2241 RSC
.
- R. Whitfield, A. Anastasaki, G. R. Jones and D. M. Haddleton, Polym. Chem., 2018, 9, 4395–4403 RSC
.
- A. Anastasaki, V. Nikolaou, G. Nurumbetov, P. Wilson, K. Kempe, J. F. Quinn, T. P. Davis, M. R. Whittaker and D. M. Haddleton, Chem. Rev., 2016, 116, 835–877 CrossRef CAS PubMed
.
- J. Sherwood, A. Constantinou, L. Moity, C. R. McElroy, T. J. Farmer, T. Duncan, W. Raverty, A. J. Hunt and J. H. Clark, Chem. Commun., 2014, 50, 9650–9652 RSC
.
- A. Marathianos, E. Liarou, E. Hancox, J. L. Grace, D. W. Lester and D. M. Haddleton, Green Chem., 2020, 22, 5833–5837 RSC
.
- A. Anastasaki, V. Nikolaou, Q. Zhang, J. Burns, S. R. Samanta, C. Waldron, A. J. Haddleton, R. McHale, D. Fox and V. Percec, J. Am. Chem. Soc., 2014, 136, 1141–1149 CrossRef CAS PubMed
.
- M. Uygun, M. A. Tasdelen and Y. Yagci, Macromol. Chem. Phys., 2010, 211, 103–110 CrossRef CAS
.
- A. B. Lowe, Polym. Chem., 2014, 5, 4820–4870 RSC
.
- N. R. Jadhav, V. L. Gaikwad, K. J. Nair and H. M. Kadam, Asian J. Pharm., 2009, 3, 82–89 CrossRef
.
|
This journal is © The Royal Society of Chemistry 2024 |
Click here to see how this site uses Cookies. View our privacy policy here.