DOI:
10.1039/D3QI01333B
(Research Article)
Inorg. Chem. Front., 2024,
11, 123-132
Efficient Nb2O5@g-C3N4 heterostructures for enhanced photocatalytic CO2 reduction with highly selective conversion to CH4†
Received
13th July 2023
, Accepted 17th September 2023
First published on 24th November 2023
Abstract
Achieving the goal of carbon neutralization using photocatalytic CO2 reduction has garnered widespread attention. However, rapid photogenerated charge recombination seriously impedes the further improvement of photocatalytic properties. In response, we propose a strategy to solve this limitation by way of constructing a heterojunction. A Nb2O5@g-C3N4 type II heterojunction photocatalyst was developed by a straightforward hydrothermal method. The construction of a heterojunction greatly accelerates the separation of photoinduced charges and increases the photocatalytic activity. As a result, Nb2O5@g-C3N4 (1
:
5) possesses outstanding properties for CO2 reduction under visible light with the production of CH4 and CO of about 19.06 and 1.93 μmol g−1, respectively. The CH4 selectivity is up to 98.79%. Furthermore, the mechanism of photocatalytic CO2 reduction is revealed in detail based on in situ DRIFTS and photochemical characterization, thus providing guidance for the design of high-performance CO2 photoreduction systems.
1. Introduction
The significantly selective catalytic reduction of CO2 to CH4 through the absorption of solar photon energy by photocatalytic semiconductors is a prospective way to achieve carbon neutrality.1–6 Nevertheless, from a kinetic perspective, CH4 formation is more challenging than the two-electron conversion of CO2 to CO since it takes eight electrons. Now, great selectivity can be obtained using strategies like choosing an ideal semiconductor to construct a heterojunction.7–9 Previous research has shown that the construction of heterojunctions is one of the most direct and operative ways to generate greatly selective CH4, and it also plays a crucial role in CO2 activation.10–13
Among a variety of semiconductors, graphitic phase carbon nitride (g-C3N4) with a special structure has attracted the attention of current researchers because of its appropriate band structure and low band gap value (Eg ∼ 2.7 eV).14,15 It is made up of two elements that are common on the Earth and have some special traits, such as good optical and electrical properties and ease of large-scale synthesis.16,17 The well-known TiO2 semiconductor is only functional in the UV spectrum, whereas a suitable band gap for g-C3N4 is 2.7 eV, which allows it to respond to visible light. Unfortunately, because photoinduced e−–h+ pairs quickly recombine, the photocatalytic capacity of pristine g-C3N4 is typically constrained by the inadequate availability of light.14,18,19 In recent years, the shortcoming was solved by creating semiconductor heterojunctions with a suitable band structure, which can greatly facilitate the separation and migration of photoinduced charges.14,20–22 To date, heterojunction photocatalysts based on g-C3N4 in abundance have been effectively designed to improve the photocatalytic activity, like g-C3N4/ZnFe2O4,23 g-C3N4/Ag3VO4,24 g-C3N4/CdS,25 Bi2WO6/g-C3N4
26 and so on. However, some of the above materials have obvious drawbacks such as poor stability, high production difficulty and high cost, which ultimately limit their practical application.
Niobium oxide (Nb2O5), an n-type semiconductor, is a promising semiconductor material for coupling to g-C3N4 among other semiconductors and possesses a high specific surface area and excellent photoactivity towards CO2 reduction and has an optical band gap of approximately 3.2 eV.27–29 The photocatalytic performance of numerous composites, including Nb2O5/RGO,30 Nb2O5/ZnO,27 Nb2O5/iron oxide,31 Nb2O5/CuO32 and TiO2/Nb2O5,33 has significantly improved as a result of the incorporation of Nb2O5 powder. Long periods of synthesis and uncontrollable heterostructure formation are significant shortcomings of the systems previously discussed. These factors can result in Nb2O5 particles having poor dispersion on the g-C3N4 surface and having large particle sizes, which lower the activity of the photocatalysts. Indeed, the construction of highly selective and active heterojunction catalysts based on g-C3N4 is still a significant challenge.
Herein, we synthesized a novel type of Nb2O5@g-C3N4 (NB@CN) heterojunction photocatalyst by a straightforward one-step hydrothermal process. The photocatalytic conversion of CO2 under LED light (a wavelength of 400 nm to 800 nm) simulated solar light irradiation is utilized to examine the photoreduction capacity of the as-produced photocatalysts. The synthesized NB@CN type II heterojunction exhibits remarkable photocatalytic CO2 reduction performance with 98.79% CH4 selectivity, greatly outperforming pristine Nb2O5 and g-C3N4 with regard to photocatalytic activity. Furthermore, the mechanism of photocatalytic CO2 reduction is investigated in detail through photochemical characterization and in situ DRIFTS, which provides a reference and new inspiration for the development and design of efficient photocatalytic systems.
2. Experimental section
Chemicals, materials, the production of Nb2O5 and g-C3N4, characterization, the experiment of photocatalytic CO2 conversion and in situ DRIFTS are presented in the ESI.†
2.1. Construction of the NB@CN heterojunction
In a typical hydrothermal preparation process, g-C3N4 (1 g) is initially added to 60 mL ultrapure water at normal temperature, stirring evenly. Then C4H4NNbO9·XH2O (200 mg) is added to the above solution. The blend is introduced into a 100 mL Teflon-lined stainless-steel autoclave after 1 hour of ultrasonication and 30 minutes of stirring. 20 hours are spent heating the autoclave to 180 °C. The white precipitates produced are centrifuged, washed five times using ultrapure water and ethanol and subsequently dried in a freezer dryer to obtain NB@CN (1
:
5). To prepare NB@CN composites with various mass ratios, the quantity of C4H4NNbO9·XH2O is regulated.
3. Results and discussion
3.1. Phase structure and morphology
XRD was conducted to look into the crystalline structure and phase purity of the produced photocatalysts. As shown in Fig. 1a, the characteristic diffraction peak of the g-C3N4 (002) crystal facet is visible at 27.2°.34 The primary characteristic diffraction peaks for hexagonal phase Nb2O5 correspond to the (001), (101), (002), (110) and (102) facets at 22.5°, 28.3°, 36.6°, 46.1°, 50.8° and 55.2° (PDF# 30-0873).35 Nonetheless, the diffraction strength of each peak of Nb2O5 falls as the g-C3N4 content increases, which is on account of the addition of g-C3N4 resulting in the reduction of Nb2O5 crystallinity. Furthermore, in comparison to g-C3N4 and Nb2O5, the XRD patterns of NB@CN contain all of the typical peaks of Nb2O5 and g-C3N4, and these findings can preliminarily demonstrate the successful construction of a heterojunction. The Fourier transform infrared spectrum (FT-IR) was employed to figure out the molecular structure of the catalysts. The results in Fig. 1b are in agreement with the FT-IR spectrum of the metal oxide, which exhibits no characteristic peaks beyond 1000 cm−1, but a spectral band that is typically below 800 cm−1.36 There is an obvious peak at 803 cm−1 for g-C3N4 and composite photocatalysts that can be attributed to the stretching vibration pattern of the triazine rings.37 Besides, the characteristic stretching vibration mode of aromatic C
N and C–N in g-C3N4 is in the 1127–1723 cm−1 region.38,39 The wide peak at 3000–3500 cm−1 is produced by the N–H of the exterior scattered amine group and the interaction of a trace of urea of partial decomposition with absorbed water.40 All these results can confirm the ideal coupling of NB@CN heterojunctions.
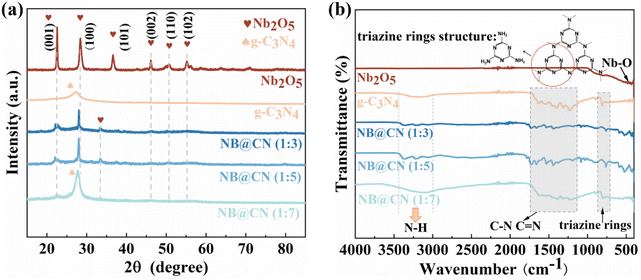 |
| Fig. 1 (a) XRD and (b) FT-IR spectrum of Nb2O5, g-C3N4 and NB@CN composites with different contents of g-C3N4. | |
SEM and TEM were employed to describe the morphology and structure of photocatalysts. Fig. 2a–c shows the NB@CN (1
:
5) heterojunction with a clumped shape, and the Nb2O5 flocculent is evenly dispersed on g-C3N4. The TEM images of the NB@CN (1
:
5) catalyst are shown in Fig. 2d–f, and g-C3N4 exhibits the typical amorphous characteristics, lacks lattice fringes, and exists in an amorphous form. Additionally, the catalyst has a 0.39 nm lattice spacing, which corresponds to the (001) plane of Nb2O5, which is consistent with the XRD findings, indicating that Nb2O5 is successfully attached to g-C3N4. Moreover, the EDX images (Fig. 2g) also prove the coexistence of Nb, C, O and N elements in the NB@CN (1
:
5) photocatalyst, which indicates that the NB@CN heterojunction is successfully constructed.
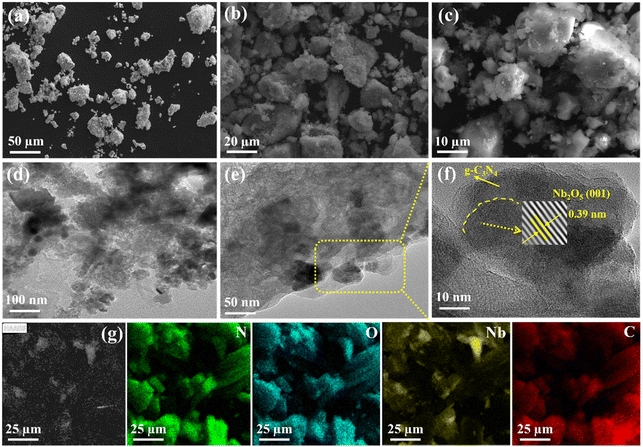 |
| Fig. 2 (a)–(c) SEM and (d)–(f) TEM of the NB@CN (1 : 5) composite photocatalyst. (g) EDS mapping of NB@CN (1 : 5), N (green), O (blue), Nb (yellow), and C (red). | |
3.2. Elemental states analysis
XPS was conducted to examine the elemental states and chemical composition of the photocatalysts. Fig. 3a shows the overall spectra of Nb2O5, g-C3N4, and NB@CN (1
:
5), revealing the existence of O, Nb, C, and N elements in NB@CN (1
:
5). The spectra also show a great match with the elemental composition of g-C3N4 and Nb2O5. The C 1s XPS spectra of NB@CN (1
:
5) and g-C3N4 are illustrated in Fig. 3b. The three fitted peaks at binding energies of 287.6 eV, 285.8 eV, and 284.2 eV can be attributed to the N–C
N and C-NHx (x = 1, 2) coordination peaks of the triazine ring in g-C3N4 and the foreign hydrocarbons (sp2, C–C), respectively.41,42 In Fig. 3c, the N 1s spectra exhibit three distinctive peaks located at 400.5 eV, 399.4 eV, and 397.9 eV, which correspond to the amino group (C–N–H), tertiary nitrogen N-(C)3 groups, and sp2 bonded nitrogen (C
N–C), respectively.43,44 The O 1s spectra in Fig. 3d show two fitted peaks at 532.2 eV and 534.1 eV, which can be assigned to the lattice oxygen of Nb2O5 and the surface adsorbed oxygen in the –OH group, respectively.45 The Nb 3d XPS spectra in Fig. 3e reveal two prominent peaks with spin orbits split to 2.7 eV, which are positioned at 207.9 eV and 210.6 eV and can be allocated to Nb 3d5/2 and Nb 3d3/2 of Nb5+.46 The great symmetry and narrow half-peak width of the two peaks indicate that Nb5+ has a single valence state.47 Compared with the XPS spectra of each element in pristine g-C3N4 and Nb2O5, the peaks of the NB@CN (1
:
5) composite are slightly shifted. These characteristics can be allocated to the powerful interaction between Nb2O5 and g-C3N4.
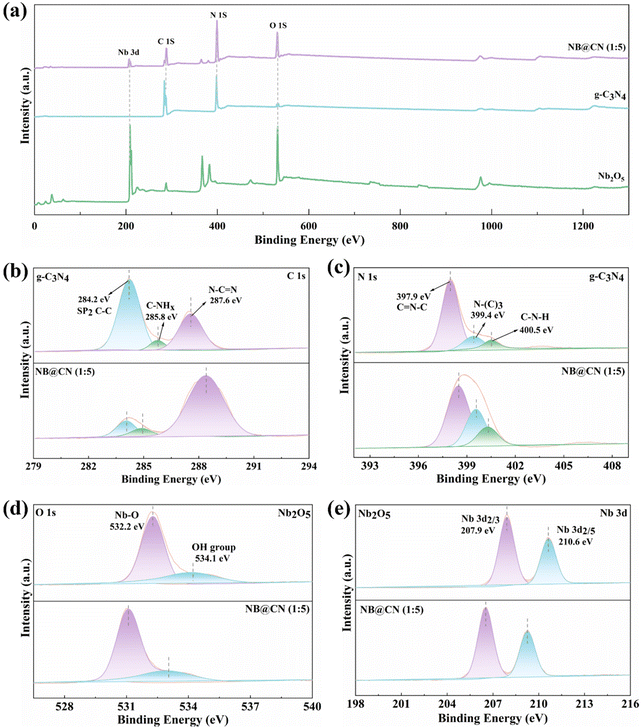 |
| Fig. 3 (a) XPS spectra of Nb2O5, g-C3N4, and NB@CN (1 : 5). High-resolution XPS spectra of (b) C 1s, (c) N 1s, (d) O 1s, and (e) Nb 3d. | |
3.3. Catalytic activity
Under visible light irradiation, photocatalytic CO2 reduction was performed for validating the impact of the constructed NB@CN (1
:
5) type II heterojunction on photo-generated carrier separation. As illustrated in Fig. 4a, the pristine Nb2O5 and g-C3N4 exhibit minimal photocatalytic CH4 productivity, which is allocated to the rapid recombination of photo-induced charges. Fascinatingly, the CH4 yield of the NB@CN (1
:
5) composite is significantly increased to 19.06 μmol g−1 compared to those of pure Nb2O5 and g-C3N4. The reason for the phenomenon could be the building of type II heterojunctions that accelerate the separation and migration of charge carriers, promote the protonation of the photocatalyst, and boost the absorption of visible light. Among them, when the mass ratio of Nb2O5 to g-C3N4 exceeds 1
:
5, the NB@CN composite photocatalysts show decreased photocatalytic capacity. The shielding effect and unneeded recombination brought on by excess g-C3N4 could be the source of the occurrence. The yield changes of CO and CH4 of the NB@CN (1
:
5) composite photocatalyst within 6 h are depicted in Fig. 4b. During the photocatalytic reaction of CO2 reduction within 10 h, the yield of products is relatively stable, and within 6 hours, there is no overt increase or decrease, exhibiting a sustained growth trend. Interestingly, after the heterojunction construction of Nb2O5 and g-C3N4, the selectivity of CH4 increases from 45.8% and 48% of pure Nb2O5 and g-C3N4 to 98.79%, as shown in Fig. 4c, which shows that the design of the NB@CN (1
:
5) heterojunction greatly improves the photocatalytic activity.
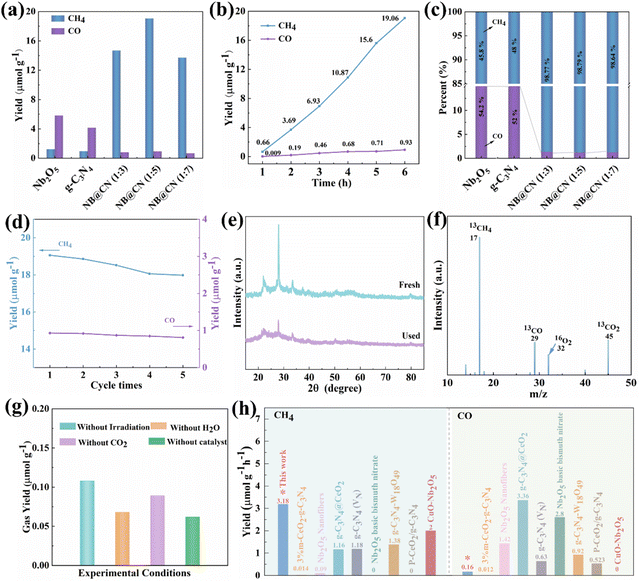 |
| Fig. 4 (a) The yield of CO and CH4 for the Nb2O5, g-C3N4 and NB@CN (1 : 5) samples with different contents of g-C3N4. (b) Alteration of the CO and CH4 production by photocatalysis in 6 h for the NB@CN (1 : 5) catalyst. (c) The selectivity of CO and CH4 for different samples. (d) The yield of CO and CH4 in cycling tests of photocatalytic CO2 reduction for NB@CN (1 : 5). (e) XRD spectra of NB@CN (1 : 5) before and after 5 cycles of CO2 photoreduction. (f) CH4 and CO mass spectra produced in 13CO2 ambience. (g) The gas yield in a controlled trial. (h) Comparison of CO and CH4 generating rate of NB@CN (1 : 5) and some Nb2O5, g-C3N4 based photocatalysts. | |
To demonstrate the steadiness of catalyst performance, we carried out a cycle test. As presented in Fig. 4d, the CO and CH4 production only attenuated slightly after five cycles, indicating that the catalyst has great stability. Moreover, XRD was performed on the samples before and after the cycle, and the diffraction peaks of the XRD patterns closely match (Fig. 4e). This confirmed that the chemical states of the NB@CN (1
:
5) photocatalyst are not altered during the photocatalytic reaction process, indicating that the catalyst is extremely stable, which is also the key reason why it shows stable performance.48 In order to clarify the sources of carbon in CH4 and CO and eradicate the interference of polluting carbon during the process of preparation, a mass spectrometry experiment with isotope labeling was carried out under a 13CO2 atmosphere, proving that every carbon-based product is transformed from CO2,49 as illustrated in Fig. 4f. The control experiments were conducted to demonstrate the necessary conditions of the photocatalytic process. As presented in Table S1† and Fig. 4g, the gas content in the four controlled experiments could be ignored revealing that CH4 products were created by the photoreduction of CO2 in NB@CN (1
:
5), and any adjustment to the reaction conditions will significantly impact the activity. In addition, we made a synthetic comparison about the catalytic reduction capability of CO2 by some Nb2O5 and g-C3N4-based photocatalytic materials, and the results demonstrate that NB@CN (1
:
5) exhibits the best photocatalytic activity (Fig. 4h and Table S4†), further indicating that the combination of Nb2O5 and g-C3N4 is advantageous for boosting the catalytic reaction activity.
3.4. Photoelectric properties
The photoluminescence (PL), time-resolved PL decay and electrochemical impedance spectrum (EIS) Nyquist plots are obtained to further research the transport characteristics of carriers for Nb2O5, g-C3N4 and NB@CN. As displayed in Fig. 5a, at the 370 nm excitation wavelength, the peak appears at nearly 400 nm. The strength of the peak corresponds to the recombination frequency of photoinduced charges. As expected, NB@CN (1
:
5) presents the weakest peak intensity, and the photoluminescence intensity is obviously quenched, indicating that the association of g-C3N4 and Nb2O5 can successfully impede the photogenerated charge recombination. The lifetime of the photo-induced carriers was characterized by the time-resolved PL decay spectra that were captured at the corresponding steady-state emission peaks, as shown in Fig. 5b. In light of the findings of fitting, NB@CN (1
:
5) has the longest carrier average lifetime (τave = 5.13 ns), which suggests that there is a more efficient photogenerated e−–h+ separation after the heterojunction construction of Nb2O5 and g-C3N4. Moreover, the EIS was utilized to further research the kinetics of charge migration for the catalysts. As illustrated in Fig. 5c, NB@CN (1
:
5) shows the smallest arc radius than that of other catalysts. In general, the resistance for charge migration is presented by the arc radius, and the decreased radius manifests a smaller resistance, that is quicker photo-induced electron–hole pair separation and transfer. Additionally, Fig. 5d illustrates that the NB@CN (1
:
5) heterojunction exhibits the strongest photocurrent response signal, compared with pure Nb2O5 and g-C3N4. Therefore, NB@CN (1
:
5) exhibits better photo-induced charge separation performance and presents better photocatalytic capacity.
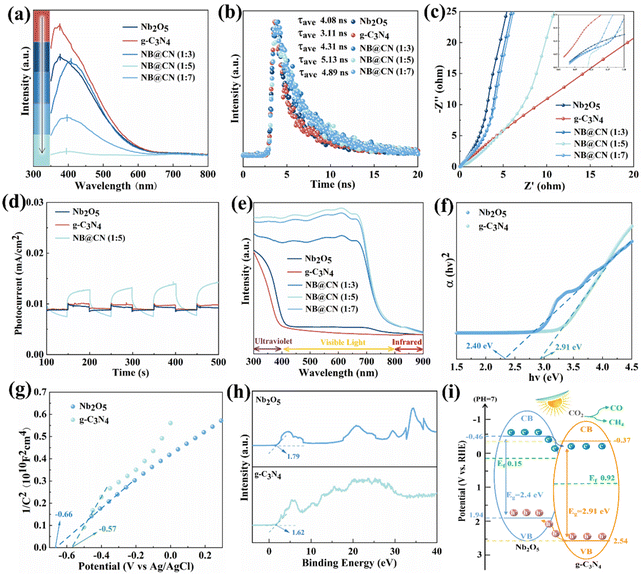 |
| Fig. 5 (a) PL spectra, (b) time-resolved PL decay spectra, (c) EIS spectra and (e) UV-vis DRS of Nb2O5, g-C3N4 and NB@CN samples with different contents of g-C3N4. (d) Photocurrent of the Nb2O5, g-C3N4 and NB@CN (1 : 5) samples. (f) Band gap energy spectra, (g) Mott–Schottky curves, (h) valence-band XPS spectra and (i) band structures of Nb2O5 and g-C3N4. | |
The optical absorption capabilities of the photocatalysts at various wavelengths are obtained through UV-Vis diffuse reflection spectroscopy. Fig. 5e depicts the UV-Vis absorption spectra in the 300–900 nm wavelength range, which demonstrates that the range of visible light response is expanded by the combination of Nb2O5 and g-C3N4. Within the wavelength range of 430–740 nm, NB@CN (1
:
5) exhibits the greatest ability to absorb visible light. As the content of g-C3N4 increased, samples started to gather, leading to a decrease in the utilization of visible light. According to the Tauc plot method,50,51 the relationship curves between (αhv)2 and hv obtained by UV-vis spectroscopy are shown in Fig. 5f. Nb2O5 and g-C3N4 have optical band gaps of 2.40 eV and 2.91 eV, respectively. The flat-band potentials (Efb) of Nb2O5 and g-C3N4 are −0.66 V and −0.57 V vs. Ag/AgCl (−0.46 and −0.37 V vs. NHE), respectively (Fig. 5g). The Mott–Schottky curve has a positive slope, showing that Nb2O5 and g-C3N4 are N-type semiconductors.36 As a result, the CBs of Nb2O5 and g-C3N4 are found to be −0.46 and −0.37 V, respectively. It is possible to deduce that the VBs are 1.94 and 2.54 V from the band gap value Eg. The distance between the VB and Ef may be calculated by VB-XPS (Fig. 5h), revealing that the Fermi level Ef values of Nb2O5 and g-C3N4 are 0.15 and 0.92 V, which are closer to the CB and comply with the band structure of N-type semiconductors.52
The band structures of Nb2O5 and g-C3N4 are depicted in Fig. 5i according to the results discussed above. It can be seen that the CB and the VB of the semiconductor Nb2O5 are higher than the CB and the VB of the semiconductor g-C3N4. The locations of the bands indicate that a type II heterojunction between Nb2O5 and g-C3N4 has formed. Thus, when semiconductors are photoexcited, photo-induced electrons migrate from the CB of the Nb2O5 semiconductor to that of the g-C3N4 semiconductor, while photoinduced holes migrate in the opposing direction, from the VB of g-C3N4 to that of Nb2O5. Finally, the photo-generated e− and h+ gather on the CB and VB of the semiconductors g-C3N4 and Nb2O5, respectively. Therefore, the separation of photoinduced e− and h+ is realized, ensuring that more photoinduced electrons can be employed for photocatalytic conversion of CO2, showing better photocatalytic performance.53
3.5. Photocatalytic CO2 reaction mechanism
The intermediates of the CO2RR process are tracked by in situ DRIFTS under photocatalytic simulation to further research the mechanism of CO2 conversion via photocatalysis. As illustrated in Fig. 6a–i, the critical intermediates in the CO2 conversion process by Nb2O5, g-C3N4 and NB@CN (1
:
5) are successfully discovered after applying photocatalytic reaction conditions.54,55 The potential mechanisms of photocatalytic conversion of CO2 to produce CO and CH4 utilizing water as an electron source are as follows: CO2 → COOH* → CO* (partially, CO↑) → CHO* → CH2O* → CH3O* → CH4.56 As shown in Fig. 6a and c, the g-C3N4 sample exhibits signal peaks at 1761 cm−1 and 1341 cm−1, which correlate to the intermediate products COOH* and HCO3* groups, respectively.57 Generally speaking, these intermediates are regarded as the primary products in the process of converting CO2 to CH4 or CO. There is a small change in these groups’ positions for the Nb2O5 and NB@CN (1
:
5) catalysts in Fig. 6d–i. However, in general, it complies with group characteristic peak position requirements. The peaks at about 1073 and 1502 cm−1 reveal the formation of CHO* and CH2O* groups, which are typically considered to be the critical intermediates for the photocatalytic reduction of CO2 to CH4 and thus provide evidence that a CO2 reduction reaction is occurring.58,59
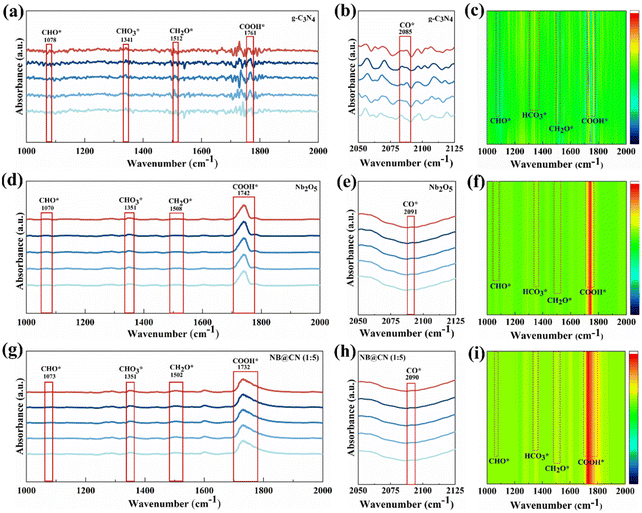 |
| Fig. 6
In situ DRIFTS spectra for co-adsorption of a mixture of CO2 and H2O on (a) and (b) g-C3N4, (d) and (e) Nb2O5 and (g) and (h) NB@CN (1 : 5) during 30–40 min. Contour maps of in situ DRIFT of (c) g-C3N4, (f) Nb2O5 and (i) NB@CN (1 : 5). | |
The catalysts exhibit weaker CO* intermediates, which may be the result of rapid conversion.60 Compared with pristine Nb2O5 and g-C3N4, the bulge peak corresponding to COOH* of NB@CN (1
:
5) at 1732 cm−1 is stronger, indicating that the building of type II heterojunction between Nb2O5 and g-C3N4 can accelerate the CO2 adsorption and activation by stabilizing the reaction intermediate COOH*. Furthermore, in the NB@CN (1
:
5) composite, the stronger CH2O* peak corresponding to 1502 cm−1 further indicates that the interaction of Nb2O5 and g-C3N4 to form a type II heterojunction is the key factor for the large amount of CH4 formation in the CO2 photoreduction process. These results reveal that NB@CN (1
:
5) possesses improved photocatalytic CO2 reduction performance and increased CH4 selectivity, which is in line with the outcomes of the performance tests.
4. Conclusions
In summary, NB@CN type II heterojunction composite photocatalysts are successfully prepared by a straightforward hydrothermal method, which combines the stable physical and chemical properties of Nb2O5 and g-C3N4 to establish a novel kind of catalytic system. The successful construction of a type II heterojunction demonstrates the effect of “killing two birds with one stone”, which not only greatly boosts the separation and migration efficiency of photo-induced charges, but also broadens the visible light response range of the composites. The above-mentioned benefits greatly facilitate the photocatalytic capacity of composites for CO2 reduction under irradiation. The results show that the NB@CN (1
:
5) composite photocatalyst has a higher selectivity for CO2 photoreduction to CH4, and its CH4 yield (19.06 μmol g−1) is 15 and 20 times that of pristine Nb2O5 (1.23 μmol g−1) and g-C3N4 (0.96 μmol g−1) for 6 h, respectively. Moreover, the mechanism of photoreduction of CO2 was thoroughly studied by in situ DRIFTS and photochemical characterization. This research offers a novel approach for achieving large-scale emission reduction and efficiently converting CO2 into clean fuels.
Author contributions
Xiaofeng Wang: writing – original draft preparation and methodology. Jingwen Jiang: editing and investigation. Lilian Wang: validation and data curation. Hong Guo: research design, funding, and supervision.
Conflicts of interest
The authors declare no conflict of interest.
Acknowledgements
The authors acknowledge the support provided by the National Natural Science Foundation of Yunnan Province (202301AS070040), the Scientific Research Foundation of Education Department of Yunnan Province (2023Y0266), the Key Laboratory of Solid-State Ions for Green Energy of Yunnan University, the Yunnan Key Laboratory of Carbon Neutrality and Green Low-carbon Technologies, the Electron Microscope Center of Yunnan University, the MCP-WS1000 Photochemical Workstation (Beijing Perfectlight), and the GC9790Plus Gas Chromatograph (ZHEJIANG FULI ANALYTICAL INSTRUMENTS INC), and the authors would like to thank Jiao Kang from Shiyanjia Lab (https://www.shiyanjia.com) for the XPS analysis.
References
- Q. J. Xu, J. W. Jiang, X. L. Sheng, Q. Jing, X. F. Wang, L. Y. Duan and H. Guo, Understanding the synergistic effect of piezoelectric polarization and the extra electrons contributed by oxygen vacancies on an efficient piezo-photocatalysis CO2 reduction, Inorg. Chem. Front., 2023, 10, 2939–2950 RSC.
- X. Wang, J. Jiang, L. Yang, Q. An, Q. Xu, Y. Yang and H. Guo, Enhanced piezoelectric polarization by subtle structure distortion to trigger efficient photocatalytic CO2RR, Appl. Catal., B, 2024, 340, 123177 CrossRef CAS.
- L. Shi, Y. Yan, Y. Wang, T. Bo, W. Zhou, X. Ren and Y. Li, Efficient and selective photocatalytic CO2 reduction over Ga single atom decorated quantum dots under visible light, Inorg. Chem. Front., 2023, 10, 2731–2741 RSC.
- Q. Xu, J. Jiang, X. Wang, L. Duan and H. Guo, Understanding oxygen vacant hollow structure CeO2@In2O3 heterojunction to promote CO2 reduction, Rare Met., 2023, 42, 1888–1898 CrossRef CAS.
- F. Chen, Y. Zhang and H. Huang, Layered photocatalytic nanomaterials for environmental applications, Chin. Chem. Lett., 2023, 34, 107523 CrossRef CAS.
- S. Cao, B. Shen, T. Tong, J. Fu and J. Yu, 2D/2D heterojunction of ultrathin MXene/Bi2WO6 nanosheets for improved photocatalytic CO2 reduction, Adv. Funct. Mater., 2018, 28, 1800136 CrossRef.
- K. T. G. Carvalho, A. E. Nogueira, O. F. Lopes, G. Byzynski and C. Ribeiro, Synthesis of g-C3N4/Nb2O5 heterostructures and their application in the removal of organic pollutants under visible and ultraviolet irradiation, Ceram. Int., 2017, 43, 3521–3530 CrossRef CAS.
- Y. Peng, X. Guo, S. Xu, Y. N. Guo, D. Zhang, M. Wang, G. Wei, X. Yang, Z. Li, Y. Zhang and F. Tian, Surface modulation of MoS2/O-ZnIn2S4 to boost photocatalytic H2 evolution, J. Energy Chem., 2022, 75, 276–284 CrossRef CAS.
- J. Lei, Y. Chen, F. Shen, L. Wang, Y. Liu and J. Zhang, Surface modification of TiO2 with g-C3N4 for enhanced UV and visible photocatalytic activity, J. Alloys Compd., 2015, 631, 328–334 CrossRef CAS.
- X. Zheng, D. Li, X. Li, J. Chen, C. Cao, J. Fang, J. Wang, Y. He and Y. Zheng, Construction of ZnO/TiO2 photonic crystal heterostructures for enhanced photocatalytic properties, Appl. Catal., B, 2015, 168–169, 408–415 CrossRef CAS.
- J. Yang, X. Zhu, Z. Mo, J. Yi, J. Yan, J. Deng, Y. Xu, Y. She, J. Qian, H. Xu and H. Li, A multidimensional In2S3-CuInS2 heterostructure for photocatalytic carbon dioxide reduction, Inorg. Chem. Front., 2018, 5, 3163–3169 RSC.
- Z. Tang, W. He, Y. Wang, Y. Wei, X. Yu, J. Xiong, X. Wang, X. Zhang, Z. Zhao and J. Liu, Ternary heterojunction in rGO-coated Ag/Cu2O catalysts for boosting selective photocatalytic CO2 reduction into CH4, Appl. Catal., B, 2022, 311, 121371 CrossRef CAS.
- K. M. Kamal, R. Narayan, N. Chandran, S. Popović, M. A. Nazrulla, J. Kovač, N. Vrtovec, M. Bele, N. Hodnik, M. M. Kržmanc and B. Likozar, Synergistic enhancement of photocatalytic CO2 reduction by plasmonic Au nanoparticles on TiO2 decorated N-graphene heterostructure catalyst for high selectivity methane production, Appl. Catal., B, 2022, 307, 121181 CrossRef CAS.
- S. Cao and J. Yu, g-C3N4-based photocatalysts for hydrogen generation, J. Phys. Chem. Lett., 2014, 5, 2101–2107 CrossRef CAS PubMed.
- Y. Ding, C. Wang, L. Pei, S. Maitra, Q. Mao, R. Zheng, M. Liu, Y. H. Ng, J. Zhong, L. H. Chen and B. L. Su, Emerging heterostructured C3N4 photocatalysts for photocatalytic environmental pollutant elimination and sterilization, Inorg. Chem. Front., 2023, 10, 3756–3780 RSC.
- L. Xu, L. Li, Z. Hu and J. C. Yu, Boosting alkaline photocatalytic H2O2 generation by incorporating pyrophosphate on g-C3N4 for effective proton shuttle and oxygen activation, Appl. Catal., B, 2023, 328, 122490 CrossRef CAS.
- Q. Han, B. Wang, Y. Zhao, C. Hu and L. Qu, A graphitic-C3N4 “seaweed” architecture for enhanced hydrogen evolution, Angew. Chem., Int. Ed., 2015, 54, 11433–11437 CrossRef CAS PubMed.
- S. Cao, J. Low, J. Yu and M. Jaroniec, Polymeric photocatalysts based on graphitic carbon nitride, Adv. Mater., 2015, 27, 2150–2176 CrossRef CAS PubMed.
- Z. Su, J. Zhang, Z. Tan, J. Hu, F. Zhang, R. Duan, L. Yao, B. Han, Y. Zhao and Y. Yang, Facilitated photocatalytic H2 production on Cu-coordinated mesoporous g-C3N4 nanotubes, Green Chem., 2023, 25, 2577–2582 RSC.
- H. Wang, L. Zhang, Z. Chen, J. Hu, S. Li, Z. Wang, J. Liu and X. Wang, Semiconductor heterojunction photocatalysts: design, construction, and photocatalytic performances, Chem. Soc. Rev., 2014, 43, 5234–5244 RSC.
- X. Sun, L. Li, S. Jin, W. Shao, H. Wang, X. Zhang and Y. Xie, Interface boosted highly efficient selective photooxidation in Bi3O4Br/Bi2O3 heterojunctions, eScience, 2023, 3, 100095 CrossRef.
- D. W ang, Y. Zheng, H. Zhao, X. Zhu, D. Ye, Y. Yang, R. Chen and Q. Liao, Core-shell β-SiC@PPCN heterojunction for promoting photo-thermo catalytic hydrogen production, ACS Catal., 2023, 13, 10104–10114 CrossRef CAS.
- Y. Yao, F. Lu, J. Qin, F. Wei, C. Xu and S. Wang, Magnetic ZnFe2O4–C3N4 hybrid for photocatalytic degradation of aqueous organic pollutants by visible light, Ind. Eng. Chem. Res., 2014, 53, 17294–17302 CrossRef CAS.
- T. Zhu, Y. Song, H. Ji, Y. Xu, Y. Song, J. Xia, S. Yin, Y. Li, H. Xu, Q. Zhang and H. Li, Synthesis of g-C3N4/Ag3VO4 composites with enhanced photocatalytic activity under visible light irradiation, Chem. Eng. J., 2015, 271, 96–105 CrossRef CAS.
- Y. Xu and W. D. Zhang, CdS/g-C3N4 hybrids with improved photostability and visible light photocatalytic activity, Eur. J. Inorg. Chem., 2015, 2015, 1744–1751 CrossRef CAS.
- Y. Tian, B. Chang, J. Lu, J. Fu, F. Xi and X. Dong, Hydrothermal synthesis of graphitic carbon nitride–Bi2WO6 heterojunctions with enhanced visible light photocatalytic activities, ACS Appl. Mater. Interfaces, 2013, 5, 7079–7085 CrossRef CAS.
- S. M. Lam, J. C. Sin, I. Satoshi, A. Z. Abdullah and A. R. Mohamed, Enhanced sunlight photocatalytic performance over Nb2O5/ZnO nanorod composites and the mechanism study, Appl. Catal., A, 2014, 471, 126–135 CrossRef CAS.
- O. F. Lopes, E. C. Paris and C. Ribeiro, Synthesis of Nb2O5 nanoparticles through the oxidant peroxide method applied to organic pollutant photodegradation: A mechanistic study, Appl. Catal., B, 2014, 144, 800–808 CrossRef CAS.
- J. Zhang, D. Li, J. Qiu, Z. Wen, X. Luo, C. Bian, J. Chen and M. Luo, Insights into the photocatalytic degradation of triclosan over amorphous Nb2O5 catalysts, Mater. Res. Express, 2020, 7, 115502 CrossRef CAS.
- Z. Yue, D. Chu, H. Huang, J. Huang, P. Yang, Y. Du, M. Zhu and C. Lu, A novel heterogeneous hybrid by incorporation of Nb2O5 microspheres and reduced graphene oxide for photocatalytic H2 evolution under visible light irradiation, RSC Adv., 2015, 5, 47117–47124 RSC.
- L. C. A. Oliveira, M. Gonçalves, M. C. Guerreiro, T. C. Ramalho, J. D. Fabris, M. C. Pereira and K. Sapag, A new catalyst material based on niobia/iron oxide composite on the oxidation of organic contaminants in water via heterogeneous Fenton mechanisms, Appl. Catal., A, 2007, 316, 117–124 CrossRef CAS.
- A. E. Nogueira, O. F. Lopes, A. B. S. Neto and C. Ribeiro, Enhanced Cr(VI) photoreduction in aqueous solution using Nb2O5/CuO heterostructures under UV and visible irradiation, Chem. Eng. J., 2017, 312, 220–227 CrossRef CAS.
- T. A. Sedneva, E. P. Lokshin, M. L. Belikov and A. T. Belyaevskii, TiO2- and Nb2O5-based photocatalytic composites, Inorg. Mater., 2013, 49, 382–389 CrossRef CAS.
- M. Liang, T. Borjigin, Y. Zhang, B. Liu, H. Liu and H. Guo, Controlled assemble of hollow heterostructured g-C3N4@CeO2 with rich oxygen vacancies for enhanced photocatalytic CO2 reduction, Appl. Catal., B, 2019, 243, 566–575 CrossRef CAS.
- Z. Xu, J. Jiang, Q. Zhang, G. Chen, L. Zhou and L. Li, 3D graphene aerogel composite of 1D-2D Nb2O5-g-C3N4 heterojunction with excellent adsorption and visible-light photocatalytic performance, J. Colloid Interface Sci., 2020, 563, 131–138 CrossRef CAS PubMed.
- J. Jiang, X. Wang, Q. Xu, Z. Mei, L. Duan and H. Guo, Understanding dual-vacancy heterojunction for boosting photocatalytic CO2 reduction with highly selective conversion to CH4, Appl. Catal., B, 2022, 316, 121679 CrossRef CAS.
- G. Ge, X. Guo, C. Song and Z. Zhao, Reconstructing supramolecular aggregates to nitrogen-deficient g-C3N4 bunchy tubes with enhanced photocatalysis for H2 production, ACS Appl. Mater. Interfaces, 2018, 10, 18746–18753 CrossRef CAS PubMed.
- L. He, M. Fei, J. Chen, Y. Tian, Y. Jiang, Y. Huang, K. Xu, J. Hu, Z. Zhao, Q. Zhang, H. Ni and L. Chen, Graphitic C3N4 quantum dots for next-generation QLED displays, Mater. Today, 2019, 22, 76–84 CrossRef CAS.
- W. Xing, C. Li, Y. Wang, Z. Han, Y. Hu, D. Chen, Q. Meng and G. Chen, A novel 2D/2D carbonized poly-(furfural alcohol)/g-C3N4 nanocomposites with enhanced charge carrier separation for photocatalytic H2 evolution, Carbon, 2017, 115, 486–492 CrossRef CAS.
- K. C. Christoforidis, T. Montini, M. Fittipaldi, J. J. D. Jaén and P. Fornasiero, Photocatalytic hydrogen production by boron modified TiO2/carbon nitride heterojunctions, ChemCatChem, 2019, 11, 6408–6416 CrossRef CAS.
- Y. Tan, Z. Shu, J. Zhou, T. Li, W. Wang and Z. Zhao, One-step synthesis of nanostructured g-C3N4/TiO2 composite for highly enhanced visible-light photocatalytic H2 evolution, Appl. Catal., B, 2018, 230, 260–268 CrossRef CAS.
- X. Lin, C. Liu, J. Wang, S. Yang, J. Shi and Y. Hong, Graphitic carbon nitride quantum dots and nitrogen-doped carbon quantum dots co-decorated with BiVO4 microspheres: A ternary heterostructure photocatalyst for water purification, Sep. Purif. Technol., 2019, 226, 117–127 CrossRef CAS.
- Y. Li, S. Wang, W. Chang, L. Zhang, Z. Wu, S. Song and Y. Xing, Preparation and enhanced photocatalytic performance of sulfur doped terminal-methylated g-C3N4 nanosheets with extended visible-light response, J. Mater. Chem. A, 2019, 7, 20640–20648 RSC.
- B. M. Armstrong, R. I. Sayler, B. H. Shupe, T. A. Stich, R. D. Britt and A. K. Franz, EPR evidence for the origin of nonlinear effects in an enantioselective Cu(II)-catalyzed spiroannulation, ACS Catal., 2019, 9, 1224–1230 CrossRef CAS.
- H. Jiang, C. Zang, Y. Zhang, W. Wang, C. Yang, B. Sun, Y. Shen and F. Bian, 2D MXene-derived Nb2O5/C/Nb2C/g-C3N4 heterojunctions for efficient nitrogen photofixation, Catal. Sci. Technol., 2020, 10, 5964–5972 RSC.
- P. Chen, W. Zhou, H. Zhang, Q. Pan, X. Zhang and B. Chu, Large thermal-electrical response and rectifying conduction behavior in asymmetrically reduced ferroelectric ceramics, ACS Appl. Electron. Mater., 2019, 1, 478–484 CrossRef CAS.
- S. Zhang, B. Zhang, D. Chen, Z. Guo, M. Ruan and Z. Liu, Promising pyro-photo-electric catalysis in NaNbO3 via integrating solar and cold-hot alternation energy in pyroelectric-assisted photoelectrochemical system, Nano Energy, 2021, 79, 105485 CrossRef CAS.
- J. Jiang, X. Zou, Z. Mei, S. Cai, Q. An, Y. Fu, H. Wang, T. Liu and H. Guo, Understanding rich oxygen vacant hollow CeO2@MoSe2 heterojunction for accelerating photocatalytic CO2 reduction, J. Colloid Interface Sci., 2022, 611, 644–653 CrossRef CAS.
- X. Wang, J. Jiang, Q. Xu, L. Duan and H. Guo, Understanding inclusive quantum dots hollow CN@CIZS heterojunction for enhanced photocatalytic CO2 reduction, Appl. Surf. Sci., 2022, 604, 154601 CrossRef CAS.
- J. Jiang, X. Wang and H. Guo, Enhanced interfacial charge transfer/separation by LSPR-induced defective semiconductor toward high CO2RR performance, Small, 2023, 2301280 CrossRef CAS.
- S. J. A. Moniz, S. A. Shevlin, D. J. Martin, Z. X. Guo and J. Tang, Visible-light driven heterojunction photocatalysts for water splitting—a critical review, Energy Environ. Sci., 2015, 8, 731–759 RSC.
- J. D. Yi, R. Xie, Z. L. Xie, G. L. Chai, T. F. Liu, R. P. Chen, Y. B. Huang and R. Cao, Highly selective CO2 electroreduction to CH4 by in situ generated Cu2O single-type sites on a conductive MOF: stabilizing key intermediates with hydrogen bonding, Angew. Chem., Int. Ed., 2020, 59, 23641–23648 CrossRef CAS.
- Y. Wang, K. Wang, J. Meng, C. Ban, Y. Duan, Y. Feng, S. Jing, J. Ma, D. Yu, L. Gan and X. Zhou, Constructing atomic surface concaves on Bi5O7Br nanotube for efficient photocatalytic CO2 reduction, Nano Energy, 2013, 109, 108305 CrossRef.
- X. Li, Y. Sun, J. Xu, Y. Shao, J. Wu, X. Xu, Y. Pan, H. Ju, J. Zhu and Y. Xie, Selective visible-light-driven photocatalytic CO2 reduction to CH4 mediated by atomically thin CuIn5S8 layers, Nat. Energy, 2019, 4, 690–699 CrossRef CAS.
- L. Liu, M. Li, F. Chen and H. Huang, Recent advances on single-atom catalysts for CO2 reduction, Small Struct., 2023, 4, 2200188 CrossRef CAS.
- J. Wang, C. Hu, Y. Zhang and H. Huang, Engineering piezoelectricity and strain sensitivity in CdS to promote piezocatalytic hydrogen evolution, Chin. J. Catal., 2022, 43, 1277–1285 CrossRef CAS.
- Y. Liu, S. Chen, X. Quan and H. Yu, Efficient electrochemical reduction of carbon dioxide to acetate on nitrogen-doped nanodiamond, J. Am. Chem. Soc., 2015, 137, 11631–11636 CrossRef CAS PubMed.
- J. T. Yates and R. R. Cavanagh, Search for chemisorbed HCO: The interaction of formaldehyde, glyoxal, and atomic hydrogen + CO with Rh, J. Catal., 1982, 74, 97–109 CrossRef CAS.
- L. Liu and Y. Li, Understanding the reaction mechanism of photocatalytic reduction of CO2 with H2O on TiO2-based photocatalysts: A review, Aerosol Air Qual. Res., 2014, 14, 453–469 CrossRef CAS.
- X. D. Zhang, T. Liu, C. Liu, D. S. Zheng, J. M. Huang, Q. W. Liu, W. W. Yuan, Y. Yin, L. R. Huang, M. Xu, Y. Li and Z. Y. Gu, Asymmetric low-frequency pulsed strategy enables ultralong CO2 reduction stability and controllable product selectivity, J. Am. Chem. Soc., 2023, 145, 2195–2206 CrossRef CAS PubMed.
|
This journal is © the Partner Organisations 2024 |
Click here to see how this site uses Cookies. View our privacy policy here.