DOI:
10.1039/D3QI02017G
(Research Article)
Inorg. Chem. Front., 2024,
11, 164-171
Fe doping and interface engineering-induced dual electronic regulation of CoSe2/Co9S8 nanorod arrays for enhanced electrochemical oxygen evolution†
Received
3rd October 2023
, Accepted 6th November 2023
First published on 7th November 2023
Abstract
Accurately engineering the electronic configuration of electrocatalysts to enhance their electrocatalytic performance is of importance in water splitting applications. Herein, a dual electronic regulation concept through constructing Fe-incorporated CoSe2 and Fe-incorporated Co9S8 heterostructure nanorod arrays directly grown on a carbon cloth substrate (abbreviated as Fe-(CoSe2/Co9S8)@CC hereafter) is demonstrated. Owing to the synergistic effect of Fe doping and CoSe2/Co9S8 heterointerfaces, the resultant Fe-(CoSe2/Co9S8)@CC electrode exhibits an accelerated charge transfer rate, improved electrical conductivity and sufficient active sites, thereby exhibiting excellent oxygen evolution reaction (OER) performance. Specifically, the engineered Fe-(CoSe2/Co9S8)@CC electrode only requires low overpotentials of 243 and 337 mV to afford current densities of 10 and 300 mA cm−2, respectively, and shows a low Tafel slope of 44.5 mV dec−1, an ideal faradaic efficiency of nearly 100%, and prominent long-term durability in an alkaline medium. This adopted double optimization strategy provides valuable guidance for the development of low-cost and high-performance transition metal-based electrocatalysts in the energy conversion field.
Introduction
In the face of the energy dilemma and environmental pollution issues, hydrogen has been commonly considered as a potential energy substitute to supersede fossil fuel thanks to its high atom utilization and extreme energy density.1–4 At present, overall water electrolysis involving the hydrogen evolution reaction (HER) and the oxygen evolution reaction (OER) represents an accessible and energy-efficient technology for generating green and high-purity hydrogen.5–7 Nevertheless, compared with the straightforward two-electron HER, the OER undergoes a complicated proton-coupled electron transfer process for the breakage of the O–H bond and the generation of a rigid O–O double bond, thus being the bottleneck of water splitting.8–10 As a consequence, high-powered electrocatalysts are indispensable for conquering the sluggish kinetics and ameliorating the reaction rate.11,12 Currently, commercial RuO2/IrO2 catalysts are generally recognized as OER benchmark electrocatalysts, but their expensive price and scarce reserves extremely confine their extensive application.13,14 Therefore, it is of great concern to consider more economical and effective non-precious metal catalysts as substitutes for the electro-catalysis of the OER.
In the recent few years, transition metal sulfides,15 phosphides,16 selenides,17 nitrides,18 oxides,19 hydroxides,20 perovskites,21 and so on have been extensively employed as potential non-noble electrocatalysts for the OER. Among the various alternatives, Co-based chalcogenides (e.g., CoxSy and CoxSey) have attracted fascinating attention in electrochemical water oxidation due to their tunable electronic structure and abundant reserves.22,23 However, their electrochemical activity and long-range stability still require further promotion for satisfactory practical applications. To confront the above challenges, tremendous endeavors have been made to modify the electronic structure to improve the intrinsic activities of catalysts, including but not limited to element incorporation and heterointerface engineering.24–27 Numerous studies have primely demonstrated that Fe atom doping into the lattice of Co-based electrocatalysts could generate a strong “Fe effect” to enhance OER performance through regulating the local electronic configuration of active centers, suppressing electrochemical oxidation of host metals and heightening electrical conductivity.28,29 In addition, integrating two glorious active components into a heterostructure at the atomic scale not only allows it to inherit the electrochemical ability of each species, but also creates plentiful heterointerfaces.30–33 The strong interfacial electron interaction could dramatically tailor the electronic distribution at the junction, thereby accelerating charge transfer efficiency and modulating the adsorption state of reaction intermediates.34–36 However, few reports are devoted to rationally considering dual electronic regulation strategies into one feasible approach to construct a metal-incorporated heterostructure for a high-performance electrochemical OER.
Enlightened by the abovementioned designing mentality, we herein report a “two in one” electronic modulation concept for synthesizing novel Fe-doped CoSe2/Fe-doped Co9S8 heterojunction nanorod arrays in situ grown on conductive carbon cloth (denoted as Fe-(CoSe2/Co9S8)@CC hereafter) through a simple topological conversion strategy using CC loaded Co(CO3)0.5OH·0.11H2O nanorod arrays (denoted as Fe-CoCH@CC hereafter) as reactive precursors. The synergistic effect of Fe doping and well-defined CoSe2/Co9S8 heterointerfaces generates a dual electronic modification function. On the one hand, the Fe incorporation could modulate the electronic structure of active species and improve the electrical conductivity. On the other hand, the construction of CoSe2/Co9S8 heterointerfaces could also regulate the electronic configuration at the heterojunction and expose numerous active sites. In addition, the in situ formation of the nanorod array structure on the CC substrate could generate numerous void channels for rapid mass transfer and favorable electrolyte penetration and provide robust mechanical strength. Benefiting from the valid multiple regulation, the resultant Fe-(CoSe2/Co9S8)@CC electrode demonstrates prominent OER performance, with small overpotentials of 243 and 337 mV to reach 10 and 300 mA cm−2, respectively, a low Tafel slope of 44.5 mV dec−1, and long-term stability for at least 28 h. This adopted “two in one” regulation method for electronic modification would open up a new avenue for the construction of transition metal-based electrocatalysts for water electrolysis.
Results and discussion
As schematically described in Fig. 1, the Fe-(CoSe2/Co9S8)@CC was fabricated through thermal transformation of the pre-prepared Fe-CoCH@CC via a synchronous “selenation–sulfuration” strategy. First, Fe-CoCH@CC was synthesized through a typical hydrothermal reaction. The X-ray diffraction (XRD) pattern (Fig. S1a†) manifests that the Fe-CoCH@CC exhibits the orthorhombic Co(CO3)0.5OH·0.11H2O phase. As observed from scanning electron microscopy (SEM) images (Fig. S1b–d†), numerous nanorod arrays with smooth surfaces are vertically arranged on the CC substrate. During the following low-temperature selenation–sulfuration process, Fe-CoCH nanorods could react with the chosen sulfur and selenium powders to form Fe-(CoSe2/Co9S8) heterostructure nanorods, in which Fe atoms are incorporated into the lattices of both CoSe2 and Co9S8. For comparison, Fe-doped CoSe2, Fe-doped Co9S8 and CoSe2/Co9S8 heterostructure decorated CC samples with single electronic modification were also fabricated on the basis of an identical preparation procedure apart from tuning the reactant species. As shown in Fig. S2,† under identical synthetic parameters apart from the absence of sulfur powder, the selenium could react with Fe-CoCH to form Fe-doped cubic-phased CoSe2 nanorod arrays, which are homogeneously loaded on CC (denoted as Fe-CoSe2@CC hereafter). Analogously, the absence of selenium leads to the formation of Fe-doped Co9S8 with cubic phase nanorod arrays grown on the CC (denoted as Fe-Co9S8@CC hereafter, Fig. S3†). In addition, when employing CoCH@CC as the reaction precursor, upon “selenation–sulfuration” treatment, the final product shows the CoSe2/Co9S8 heterostructure nanorod arrays aligned on the CC substrate (abbreviated as CoSe2/Co9S8@CC hereafter, Fig. S4†). The abovementioned controlled experiments unquestionably confirm that the simultaneous introduction of S, Se and Fe is essential for the generation of CoSe2/Co9S8 heterostructure nanorod arrays.
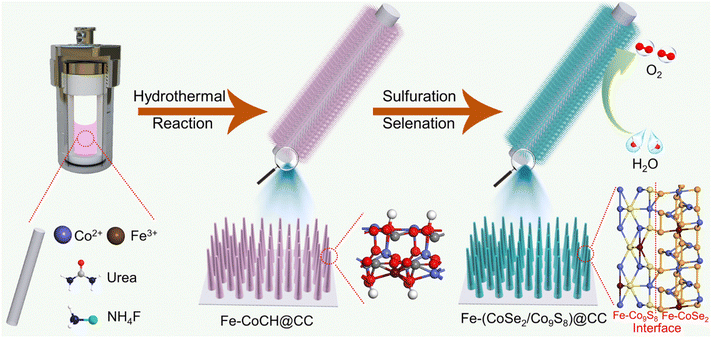 |
| Fig. 1 Schematic illustration of the preparation of Fe-(CoSe2/Co9S8)@CC. | |
As shown in the XRD pattern (Fig. 2a), the diffraction peaks of the resultant Fe-(CoSe2/Co9S8)@CC sample are assigned to cubic-phased CoSe2 and cubic-phased Co9S8, suggesting the generation of a heterojunction. The morphological and structural features of the Fe-(CoSe2/Co9S8)@CC sample were first evaluated using SEM images. As shown in Fig. 2b and c, the obtained Fe-(CoSe2/Co9S8)@CC sample inherits the nanorod array structure of parent Fe-CoCH@CC after mild selenation–sulfuration reactions. However, the magnified SEM image (Fig. 2d) demonstrates that the surface of these nanorods becomes rough, which may be due to the dehydration and decomposition of Fe-CoCH precursors during the selenation–sulfuration process.
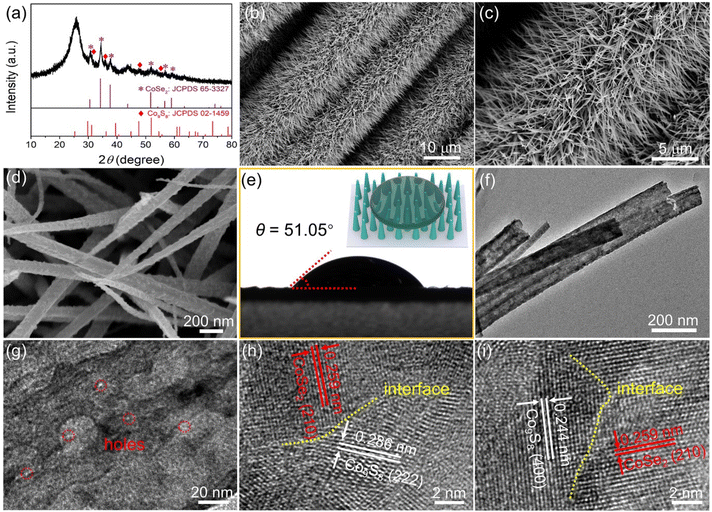 |
| Fig. 2 Compositional and morphological features of the as-synthesized Fe-(CoSe2/Co9S8)@CC. (a) XRD pattern, (b–d) SEM images, (e) contact angle test, (f and g) TEM images, and (h and i) HRTEM images. | |
As shown in Fig. 2e, the water contact angle (CA) of Fe-(CoSe2/Co9S8)@CC is determined to be 51.05°, reflecting its superior hydrophilicity. This favorable hydrophilic feature is conducive to wettability and gas bubble release on the surface of the electrocatalyst. The transmission electron microscopy (TEM) image (Fig. 2f) further confirms the rod-like morphology of Fe-(CoSe2/Co9S8). The magnified TEM image (Fig. 2g) indicates the presence of nanoholes in the nanorods. It is extensively recognized that such nanorod arrays with rough and porous structures could effectively expand the accessible area for electrolyte infiltration, enrich active sites and promote the electron transport efficiency.37,38 As shown in the high-resolution TEM (HRTEM) images (Fig. 2h and i), the well-resolved lattice fringes with an interplanar spacing of 0.259 nm belong to the (210) plane of cubic CoSe2, while the distinct interplanar spacings of 0.286 and 0.244 nm can be attributed to be (222) and (400) planes of cubic Co9S8, respectively. Notably, distinct interfaces between Co9S8 and CoSe2 could be noticed from the HRTEM images. The energy-dispersive X-ray (EDX) mapping images (Fig. S5a†) and compositional line profiles (Fig. S5b†) convincingly testify to the homogeneous dispersion of Co, Fe, S and Se elements throughout the whole Fe-(CoSe2/Co9S8) nanorods.
X-ray photoelectron spectroscopy (XPS) was employed to analyze the surface chemistry of the electrocatalyst. The XPS survey spectrum (Fig. S6†) discloses the coexistence of Co, Fe, S and Se elements in the harvested product. In the high-resolution Co 2p spectrum of Fe-(CoSe2/Co9S8)@CC (Fig. 3a), the characteristic peaks centered at 778.51 and 793.45 eV are ascribed to the metallic Co 2p3/2 and Co 2p1/2 peaks, respectively, while two broad peaks at 781.6 and 797.18 eV are assigned to the Co2+ 2p3/2 and Co2+ 2p1/2 peaks, respectively.39,40 Furthermore, as shown in Fig. 3b, the remarkable thing is that the metallic Co 2p3/2 peak in Fe-(CoSe2/Co9S8)@CC slightly positively shifts to a higher binding energy region in comparison with those of Fe-Co9S8@CC and CoSe2/Co9S8@CC, but negatively shifts to a lower binding energy region compared with that of Fe-CoSe2@CC. This result unambiguously manifests that both Fe doping and the CoSe2/Co9S8 heterostructure play important roles in the electronic modulation of active sites.41 The high-resolution Fe 2p spectrum (Fig. 3c) can be deconvoluted into three peaks, namely the Fe2+ 2p3/2 peak (710.54 eV), Fe3+ 2p3/2 peak (713.96 eV) and satellite peak (718.99 eV).42 In the high-resolution S 2p spectrum (Fig. 3d), two peaks located at 161.82 and 162.88 eV can correspond to S 2p3/2 and S 2p1/2 peaks, respectively.43 A weak peak at 168.6 eV belongs to the sulfate/sulfite species on account of the surface oxidation of sulfides.44 In addition, the other two peaks positioned at 160.6 and 165.4 eV belong to the Se 3p3/2 and Se 3p1/2 peaks, respectively.45 The high-resolution Se 3d spectrum (Fig. 3e) demonstrates three predominant peaks of Se 3d5/2 (54.23 eV), Se 3d3/2 (55.03 eV) and selenate/selenite (59.45 eV), respectively.46,47 As shown in the electron spin resonance (ESR) spectra (Fig. 3f), the resultant Fe-(CoSe2/Co9S8)@CC sample exerts the most strong signal intensity at a g value of 2.003 compared to Fe-Co9S8@CC, Fe-CoSe2@CC and CoSe2/Co9S8@CC samples, reflecting the generation of more oxygen vacancies.
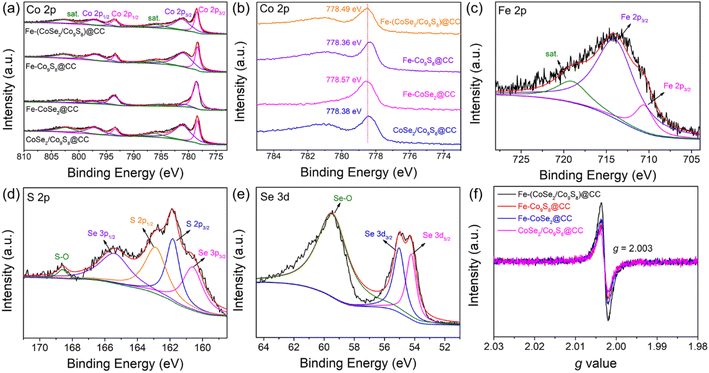 |
| Fig. 3 Surface chemistry of the as-prepared Fe-(CoSe2/Co9S8)@CC. (a and b) Co 2p spectra, (c) Fe 2p spectrum, (d) S 2p spectrum, (e) Se 3d spectrum, and (f) ESR spectra. | |
To investigate the dual electronic regulation effect of Fe incorporation and the CoSe2/Co9S8 heterostructure, the electrochemical OER performances of all the electrodes were evaluated in an O2-saturated 1.0 M KOH solution by utilizing a three-electrode system. For comparison, commercial RuO2 was also estimated as a benchmark. Fig. 4a shows the linear sweep voltammetry (LSV) curves of all the samples. Apparently, the earlier turning point and steep curve indicate that the Fe-(CoSe2/Co9S8)@CC electrode exhibits the most prominent OER activity among all the electrodes. Concretely speaking, as shown in Fig. 4b, to reach a current density of 10 mA cm−2, the Fe-(CoSe2/Co9S8)@CC electrode only requires a low overpotential of 243 mV, evidently smaller than those of Fe-Co9S8@CC (266 mV), Fe-CoSe2@CC (298 mV), CoSe2/Co9S8@CC (279 mV), Fe-CoCH@CC (309 mV) and commercial RuO2 (341 mV). Notably, even at a large current density, the Fe-(CoSe2/Co9S8)@CC electrode demonstrates superior electrocatalytic performance. For instance, when the current density is at 300 mA cm−2 (Fig. 4c), the overpotentials for the Fe-(CoSe2/Co9S8)@CC electrode is 337 mV, whereas Fe-Co9S8@CC, Fe-CoSe2@CC, CoSe2/Co9S8@CC and Fe-CoCH@CC need evidently larger overpotentials of 386, 391, 395 and 407 mV, respectively. Tafel slopes were employed to appraise the reaction kinetics and mechanisms. As displayed in Fig. 4d, the Tafel slope of the Fe-(CoSe2/Co9S8)@CC electrode is identified as 44.5 mV dec−1, visibly smaller than those of Fe-Co9S8@CC (63.7 mV dec−1), Fe-CoSe2@CC (61.4 mV dec−1), CoSe2/Co9S8@CC (64.8 mV dec−1), Fe-CoCH@CC (68.2 mV dec−1) and commercial RuO2 (95.7 mV dec−1), reflecting a favorable reaction kinetics proceeding on the Fe-(CoSe2/Co9S8)@CC electrode. These above results make clear that the OER performance of Fe-(CoSe2/Co9S8)@CC surpasses the performances of the majority of recently reported transition metal chalcogenide-based electrocatalysts, as briefly summarized in Table S1.† The electrochemical double-layer capacitance (Cdl) was calculated by cyclic voltammetry (CV) with different scan rates in a non-faradaic region (Fig. S7†). As shown in Fig. 4e, the Cdl values of Fe-(CoSe2/Co9S8)@CC, Fe-Co9S8@CC, Fe-CoSe2@CC, CoSe2/Co9S8@CC and Fe-CoCH@CC electrodes are determined to be 351.1, 290.9, 332.5, 286.9 and 112.8 mF cm−2, respectively. The larger Cdl value demonstrates that the Fe doping and CoSe2/Co9S8 heterostructure exert a positive effect on the generation of active sites, thereby promoting the OER activity. In view of the similar nanorod array structures of all the tested electrodes, the evidently enhanced OER performance of the Fe-(CoSe2/Co9S8)@CC electrode can be reasonably put down to the “two in one” regulation strategy. This phenomenon may be due to the reason that the dual optimization method makes up for the shortfall in single electronic adjustment via heteroatom doping or heterointerface engineering.
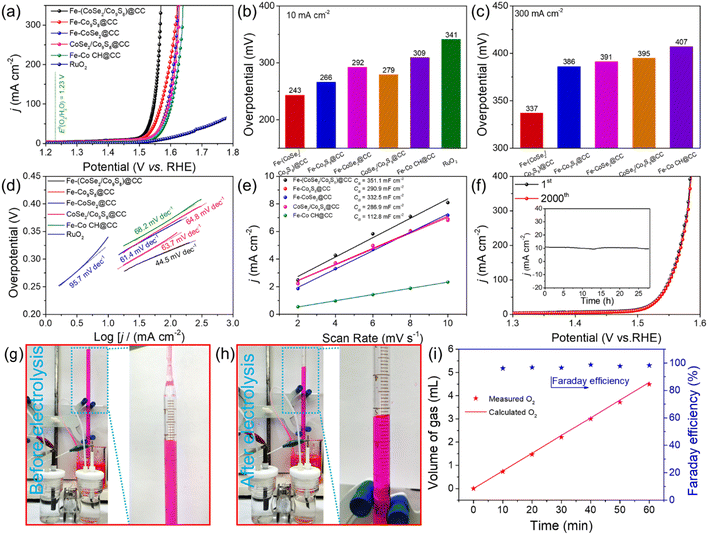 |
| Fig. 4 Evaluation of the OER performances of the harvested self-supported electrodes. (a) LSV polarization curves, (b) overpotentials at 10 mA cm−2, (c) overpotentials at 300 mA cm−2, (d) Tafel slopes, (e) calculated Cdl values, (f) LSV curves of Fe-(CoSe2/Co9S8)@CC before and after a 2000-cycle CV test. The inset of panel (f) presents the chronopotentiometry curve at a constant applied potential of 1.55 V. (g and h) Digital photographs of the electrolyzer before and after the OER, and (i) theoretical and generated volumes of O2 during the OER process at 20 mA cm−2 for 1 h, and the corresponding faradaic efficiency. | |
Besides the electrocatalytic activity, long-term stability is also an essential parameter for the estimation of electrocatalysts in practical applications. The almost coincident LSV curves before and after 2000 cycles of CV measurements (Fig. 4f) testify to the prominent electrochemical stability of the obtained Fe-(CoSe2/Co9S8)@CC electrode. As witnessed from the amperometric i–t curve (inset of Fig. 4f), the current density presents negligible degradation even after 28 h at a constant potential of 1.55 V, further confirming its prominent long-term stability. SEM, TEM and XPS techniques were carried out to investigate the physical and chemical characterization of Fe-(CoSe2/Co9S8)@CC after the stability test. SEM images (Fig. S8a and b†) and TEM images (Fig. S8c and d†) reveal that the Fe-(CoSe2/Co9S8)@CC electrode retains the nanorod array structure after the stability test. The HRTEM image (Fig. S8e†) shows that the surface of the sample is oxidized to CoOOH species in the high potential region, forming a CoOOH/Co9S8/CoSe2 heterostructure, which works as the actual active site for the OER.48,49 What is more, Co 2p spectra (Fig. S8f†) further reveal the generation of CoOOH during the OER stability test, while there is no obvious change of Fe 2p peaks (Fig. S8g†). The S 2p and Se 3d spectra (Fig. S8h and i†) certify that most of S and Se elements are oxidized to sulfate/sulfite and selenate/selenite, respectively. Moreover, as shown in Fig. 4g and h, the generated O2 was gathered by the water drainage method. Fig. 4i demonstrates that the volume of harvested O2 gas coincides well with the theoretical value, which implies almost 100% faradaic efficiency during the OER process. Considering the glorious activity and long-term stability, the synthesized Fe-(CoSe2/Co9S8)@CC electrode holds great promise for widespread application in the water splitting field.
In one word, the impressive OER performance of the Fe-(CoSe2/Co9S8)@CC electrode can be ascribed to the particular compositional and morphological advantages. First, the Fe doping dramatically alters the electronic state of active sites and improves the electrical conductivity. Second, the elaborate CoSe2/Co9S8 heterostructure also powerfully regulates the electronic configuration, thus benefiting the enormous exposure of active sites and modifying the adsorption behaviour of the reaction intermediate. Last, the vertically aligned nanorod array structure with open voids effectively promotes electrolyte permeation, accelerates mass transportation and expedites gas bubble release during the OER process.
Conclusions
All in all, this work adopts a dual modulation strategy for regulating the electronic structure of the CoSe2/Co9S8 heterojunction through Fe doping for enhanced OER performance. Electrochemical results indicate that the double electronic modification through Fe incorporation and CoSe2/Co9S8 heterostructure engineering endows the resultant Fe-(CoSe2/Co9S8)@CC electrode with a rapid charge transfer rate, plentiful active sites and tailored adsorption free energies, thus resulting in excellent electrocatalytic OER performance. Specifically, the Fe-(CoSe2/Co9S8)@CC electrode only requires small overpotentials of 243 and 337 mV to afford current densities of 10 and 300 mA cm−2, respectively, with a low Tafel slope of 44.5 mV dec−1 and long-term durability for at least 28 h. So the prominent OER property makes the Fe-(CoSe2/Co9S8)@CC a potential and economical alternative for practical water splitting. We have infinite faith in the dual electronic engineering approach in this study which could enlighten the future design of transition metal electrocatalysts in energy conversion applications.
Conflicts of interest
There are no conflicts to declare.
Acknowledgements
This work was financially supported by the National Natural Science Foundation of China (22302096), the Natural Science Foundation of Jiangsu Higher Education Institutions of China (23KJD150004) and the High-level Talents Project of Jinling Institute of Technology (jit-b-202164).
References
- S. C. Sun, H. Jiang, Z. Y. Chen, Q. Chen, M. Y. Ma, L. Zhen, B. Song and C. Y. Xu, Bifunctional WC-supported RuO2 nanoparticles for robust water splitting in acidic media, Angew. Chem., Int. Ed., 2022, 61, e202202519 CrossRef CAS PubMed.
- M. Zhao, W. Li, J. Li, W. Hu and C. M. Li, Strong electronic interaction enhanced electrocatalysis of metal sulfide clusters embedded metal-organic framework ultrathin nanosheets toward highly efficient overall water splitting, Adv. Sci., 2020, 7, 2001965 CrossRef CAS PubMed.
- Y. Pan, K. Sun, S. Liu, X. Cao, K. Wu, W. C. Cheong, Z. Chen, Y. Wang, Y. Li, Y. Liu, D. Wang, Q. Peng, C. Chen and Y. Li, Core-shell ZIF-8@ZIF-67-derived CoP nanoparticle-embedded N-doped carbon nanotube hollow polyhedron for efficient overall water splitting, J. Am. Chem. Soc., 2018, 140, 2610–2618 CrossRef CAS PubMed.
- G. Zhou, Y. Ma, C. Gu, J. Yang, H. Pang, J. Li, L. Xu and Y. Tang, Fe incorporation-induced electronic modification of Co-tannic acid complex nanoflowers for high-performance water oxidation, Inorg. Chem. Front., 2022, 9, 1091–1099 RSC.
- X. Li, L. Xiao, L. Zhou, Q. Xu, J. Weng, J. Xu and B. Liu, Adaptive bifunctional electrocatalyst of amorphous CoFe oxide @ 2D black phosphorus for overall water splitting, Angew. Chem., Int. Ed., 2020, 59, 21106–21113 CrossRef CAS PubMed.
- S. Zhang, X. Zhou, G. Zhou, B. He, H. Pang, L. Xu and Y. Tang, Template-assisted fabrication of O-doped CoP microflowers with optimal electronic modulation for electrochemical hydrogen evolution, Chem. – Eur. J., 2023, 29, e202301252 CrossRef CAS PubMed.
- R. Zhang, Z. Wei, G. Ye, G. Chen, J. Miao, X. Zhou, X. Zhu, X. Cao and X. Sun, “d-electron complementation” induced V-Co phosphide for efficient overall water splitting, Adv. Energy Mater., 2021, 11, 2101758 CrossRef CAS.
- B. He, P. Zhao, G.-X. Pan, Q. Lu, H.-Q. Li, F. Ye, Y.-W. Tang, Q.-L. Hao and Z. Su, Interface engineering of NiTe/NiCo-LDH core-shell structure to enhance oxygen evolution electrocatalysis performance, J. Alloys Compd., 2023, 938, 168673 CrossRef CAS.
- B. Qiu, C. Wang, N. Zhang, L. Cai, Y. Xiong and Y. Chai, CeO2-induced interfacial Co2+ octahedral sites and oxygen vacancies for water oxidation, ACS Catal., 2019, 9, 6484–6490 CrossRef CAS.
- X. Wang, J. Li, Q. Xue, X. Han, C. Xing, Z. Liang, P. Guardia, Y. Zuo, R. Du, L. Balcells, J. Arbiol, J. Llorca, X. Qi and A. Cabot, Sulfate-decorated amorphous-crystalline cobalt-iron oxide nanosheets to enhance O-O coupling in the oxygen evolution reaction, ACS Nano, 2023, 17, 825–836 CrossRef CAS PubMed.
- L. Han, S. Dong and E. Wang, Transition-metal (Co, Ni, and Fe)-based electrocatalysts for the water oxidation reaction, Adv. Mater., 2016, 28, 9266–9291 CrossRef CAS PubMed.
- B. Yang, C. Gu, Q. Zhao, G. Zhou, L. Xu and H. Pang, Reactive template-engaged synthesis of Ni-doped Co3S4 hollow and porous nanospheres with optimal electronic modulation toward high-efficiency electrochemical oxygen evolution, Inorg. Chem. Front., 2022, 9, 3924–3932 RSC.
- Y. Zhao, Y. Wang, Y. Dong, C. Carlos, J. Li, Z. Zhang, T. Li, Y. Shao, S. Yan, L. Gu, J. Wang and X. Wang, Quasi-two-dimensional earth-abundant bimetallic electrocatalysts for oxygen evolution reactions, ACS Energy Lett., 2021, 6, 3367–3375 CrossRef CAS.
- G. Zhou, M. Li, Y. Li, H. Dong, D. Sun, X. Liu, L. Xu, Z. Tian and Y. Tang, Regulating the electronic structure of CoP nanosheets by O incorporation for high-efficiency electrochemical overall water splitting, Adv. Funct. Mater., 2020, 30, 1905252 CrossRef CAS.
- P. Yu, L. Zeng, K. Li, C. Zhang, K. Wang, L. Li, Y. Liang, K. Yan and H. Luo, Synthesis of Co9S8 nanoflakes by a one-step solvent-free solid-state method for multiple electrocatalytic reactions, Inorg. Chem. Front., 2023, 10, 2586–2593 RSC.
- J. Wang and F. Ciucci,
In-situ synthesis of bimetallic phosphide with carbon tubes as an active electrocatalyst for oxygen evolution reaction, Appl. Catal., B, 2019, 254, 292–299 CrossRef CAS.
- C. Gu, S. Hu, X. Zheng, M. R. Gao, Y. R. Zheng, L. Shi, Q. Gao, X. Zheng, W. Chu, H. B. Yao, J. Zhu and S. H. Yu, Synthesis of sub-2 nm iron-doped NiSe2 nanowires and their surface-confined oxidation for oxygen evolution catalysis, Angew. Chem., Int. Ed., 2018, 57, 4020–4024 CrossRef CAS PubMed.
- D. Liu, H. Ai, J. Li, M. Fang, M. Chen, D. Liu, X. Du, P. Zhou, F. Li, K. H. Lo, Y. Tang, S. Chen, L. Wang, G. Xing and H. Pan, Surface reconstruction and phase transition on vanadium-cobalt-iron trimetal nitrides to form active oxyhydroxide for enhanced electrocatalytic water oxidation, Adv. Energy Mater., 2020, 10, 2002464 CrossRef CAS.
- Y. Liu, C. Ma, Q. Zhang, W. Wang, P. Pan, L. Gu, D. Xu, J. Bao and Z. Dai, 2D electron gas and oxygen vacancy induced high oxygen evolution performances for advanced Co3O4/CeO2 nanohybrids, Adv. Mater., 2019, 31, 1900062 CrossRef PubMed.
- M. Qin, G. Ma, W. Tan, Z. Fan and X. Xin, Constructing an n-n junction between CoFe-LDH and NiCoP as bifunctional electrocatalysts for efficient overall water splitting, Inorg. Chem. Front., 2023, 10, 4819–4828 RSC.
- F. Dong, L. Li, Z. Kong, X. Xu, Y. Zhang, Z. Gao, B. Dongyang, M. Ni, Q. Liu and Z. Lin, Materials engineering in perovskite for optimized oxygen evolution electrocatalysis in alkaline condition, Small, 2021, 17, 2006638 CrossRef CAS PubMed.
- P. Thangasamy, S. Oh, S. Nam, H. Randriamahazaka and I. K. Oh, Ferrocene-incorporated cobalt sulfide nanoarchitecture for superior oxygen evolution reaction, Small, 2020, 16, 2001665 CrossRef CAS PubMed.
- Z. Wang, Z. Lin, J. Deng, S. Shen, F. Meng, J. Zhang, Q. Zhang, W. Zhong and L. Gu, Elevating the d-band center of six-coordinated octahedrons in Co9S8 through Fe-incorporated topochemical deintercalation, Adv. Energy Mater., 2021, 11, 2003023 CrossRef CAS.
- M.-I. Jamesh and M. Harb, Tuning the electronic structure of the earth-abundant electrocatalysts for oxygen evolution reaction (OER) to achieve efficient alkaline water splitting-A review, J. Energy Chem., 2021, 56, 299–342 CrossRef CAS.
- X. Ji, Y. Lin, J. Zeng, Z. Ren, Z. Lin, Y. Mu, Y. Qiu and J. Yu, Graphene/MoS2/FeCoNi(OH)x and Graphene/MoS2/FeCoNiPx multilayer-stacked vertical nanosheets on carbon fibers for highly efficient overall water splitting, Nat. Commun., 2021, 12, 1380 CrossRef CAS PubMed.
- W. Jia, Q. Lu, W. Zheng, K. Wang, X. Liu, S. Yang and B. He, V-doped porous CoP nanoarrays grown on carbon cloth with optimized electronic structure for the hydrogen evolution reaction, Nanoscale Adv., 2023, 5, 4133–4139 RSC.
- Z. Zhang, X. Xie, H. Xie, X. Ding, J. Cui, C. Liu, D. Luo and Z. Lin, Dual-site doping to enhance oxygen redox and structural stability of Li-rich layered oxides, Chin. J. Struct. Chem., 2022, 41, 2204061–2204067 CAS.
- S. Anantharaj, S. Kundu and S. Noda, “The Fe Effect”: A review unveiling the critical roles of Fe in enhancing OER activity of Ni and Co based catalysts, Nano Energy, 2021, 80, 105514 CrossRef CAS.
- L. M. Cao, Y. W. Hu, S. F. Tang, A. Iljin, J. W. Wang, Z. M. Zhang and T. B. Lu, Fe-CoP electrocatalyst derived from a bimetallic prussian blue analogue for large-current-density oxygen evolution and overall water splitting, Adv. Sci., 2018, 5, 1800949 CrossRef PubMed.
- R. Li, H. Zhang, J. Chen, K. Zhang, W. Li, X. Feng, H. Zhang, I. D. Abdoulkader, X. Zhang and T. Zhang, Interfacial engineering of FeWO4/Fe2O3 homometallic heterojunctions for synergistic electrocatalytic water splitting, Inorg. Chem. Front., 2023, 10, 3675–3685 RSC.
- J. Chen, F. Zheng, S.-J. Zhang, A. Fisher, Y. Zhou, Z. Wang, Y. Li, B.-B. Xu, J.-T. Li and S.-G. Sun, Interfacial interaction between FeOOH and Ni−Fe LDH to modulate the local electronic structure for enhanced OER electrocatalysis, ACS Catal., 2018, 8, 11342–11351 CrossRef CAS.
- J. Zhu and S. Mu, Parsing the basic principles to build efficient heterostructures toward electrocatalysis, Inorg. Chem. Front., 2023, 10, 2220–2225 RSC.
- S. Xu, Y. Zhou, G. Shen and B. Dong, Ni(OH)2 derived from NiS2 induced by reflux playing three roles for hydrogen/oxygen evolution reaction, Chin. J. Struct. Chem., 2022, 41, 2208052–2208057 CAS.
- G. Zhou, S. Zhang, Y. Zhu, J. Li, K. Sun, H. Pang, M. Zhang, Y. Tang and L. Xu, Manipulating the rectifying contact between ultrafine Ru nanoclusters and N-doped carbon nanofibers for high-efficiency pH-universal electrocatalytic hydrogen evolution, Small, 2023, 19, 2206781 CrossRef CAS PubMed.
- C. Li, S. H. Kim, H. Y. Lim, Q. Sun, Y. Jiang, H. J. Noh, S. J. Kim, J. Baek, S. K. Kwak and J. B. Baek, Self-accommodation induced electronic metal-support interaction on ruthenium site for alkaline hydrogen evolution reaction, Adv. Mater., 2023, 35, 2301369 CrossRef CAS PubMed.
- M. Li and L. Feng, NiSe2-CoSe2 with a hybrid nanorods and nanoparticles structure for efficient oxygen evolution reaction, Chin. J. Struct. Chem., 2022, 41, 2201019–2201024 CAS.
- Y. Yang, H. Yao, Z. Yu, S. M. Islam, H. He, M. Yuan, Y. Yue, K. Xu, W. Hao, G. Sun, H. Li, S. Ma, P. Zapol and M. G. Kanatzidis, Hierarchical nanoassembly of MoS2/Co9S8/Ni3S2/Ni as a highly efficient eelectrocatalyst for overall water splitting in a wide pH range, J. Am. Chem. Soc., 2019, 141, 10417–10430 CrossRef CAS PubMed.
- Z.-Y. Yu, C.-C. Lang, M.-R. Gao, Y. Chen, Q.-Q. Fu, Y. Duan and S.-H. Yu, Energy Environ. Sci., 2018, 11, 1890–1897 RSC.
- E. Hu, Y. Feng, J. Nai, D. Zhao, Y. Hu and X. W. D. Lou, Ni-Mo-O nanorod-derived composite catalysts for efficient alkaline water-to-hydrogen conversion via urea electrolysis, Energy Environ. Sci., 2018, 11, 1890–1897 RSC.
- Y. Sun, K. Mao, Q. Shen, L. Zhao, C. Shi, X. Li, Y. Gao, C. Li, K. Xu and Y. Xie, Surface electronic structure modulation of cobalt nitride nanowire arrays via selenium deposition for efficient hydrogen evolution, Adv. Funct. Mater., 2022, 32, 2109792 CrossRef CAS.
- Y. Zhang, R. Lu, C. Wang, Y. Zhao and L. Qi, Electronic and vacancy engineering of Mo–RuCoOx nanoarrays for high-efficiency water splitting, Adv. Funct. Mater., 2023, 33, 2303073 CrossRef CAS.
- Z. Li, S. Ji, C. Xu, L. Leng, H. Liu, J. H. Horton, L. Du, J. Gao, C. He, X. Qi, Q. Xu and J. Zhu, Engineering the electronic structure of single-atom iron sites with boosted oxygen bifunctional activity for zinc-air batteries, Adv. Mater., 2022, 35, 2209644 CrossRef PubMed.
- C. Gu, G. Zhou, J. Yang, H. Pang, M. Zhang, Q. Zhao, X. Gu, S. Tian, J. Zhang, L. Xu and Y. Tang, NiS/MoS2 Mott-Schottky heterojunction-induced local charge redistribution for high-efficiency urea-assisted energy-saving hydrogen production, Chem. Eng. J., 2022, 443, 136321 CrossRef CAS.
- R. Fan, J. Zhou, W. Xun, S. Cheng, S. Vanka, T. Cai, S. Ju, Z. Mi and M. Shen, Highly efficient and stable Si photocathode with hierarchical MoS2/Ni3S2 catalyst for solar hydrogen production in alkaline media, Nano Energy, 2020, 71, 104631 CrossRef CAS.
- L. Liao, J. Sun, D. Li, F. Yu, Y. Zhu, Y. Yang, J. Wang, W. Zhou, D. Tang, S. Chen and H. Zhou, Highly robust non-noble alkaline hydrogen-evolving electrocatalyst from Se-doped molybdenum disulfide particles on interwoven CoSe2 nanowire arrays, Small, 2020, 16, 1906629 CrossRef CAS PubMed.
- D. Chen, R. Lu, Y. Yao, D. Wu, H. Zhao, R. Yu, Z. Pu, P. Wang, J. Zhu, J. Yu, P. Ji, Z. Kou, H. Tang and S. Mu, Duetting electronic structure modulation of Ru atoms in RuSe2@NC enables more moderate H* adsorption and water dissociation for hydrogen evolution reaction, J. Mater. Chem. A, 2022, 10, 7637–7644 RSC.
- Z. Huang, B. Xu, Z. Li, J. Ren, H. Mei, Z. Liu, D. Xie, H. Zhang, F. Dai, R. Wang and D. Sun, Accurately regulating the electronic structure of NixSey@NC core-shell nanohybrids through controllable selenization of a Ni-MOF for pH-universal hydrogen evolution reaction, Small, 2020, 16, 2004231 CrossRef CAS PubMed.
- Y.-P. Zhu, Y.-P. Liu, T.-Z. Ren and Z.-Y. Yuan, Self-supported cobalt phosphide mesoporous nanorod arrays: a flexible and bifunctional electrode for highly active electrocatalytic water reduction and oxidation, Adv. Funct. Mater., 2015, 25, 7337–7347 CrossRef CAS.
- Q. Ji, Y. Kong, H. Tan, H. Duan, N. Li, B. Tang, Y. Wang, S. Feng, L. Lv, C. Wang, F. Hu, W. Zhang, L. Cai and W. Yan, Operando identification of active species and intermediates on sulfide interfaced by Fe3O4 for ultrastable alkaline oxygen evolution at large current density, ACS Catal., 2022, 12, 4318–4326 CrossRef CAS.
|
This journal is © the Partner Organisations 2024 |
Click here to see how this site uses Cookies. View our privacy policy here.