DOI:
10.1039/D3RA07365C
(Paper)
RSC Adv., 2024,
14, 4089-4096
Efficient and eco-friendly detection of gabapentin using nitrogen-doped carbon quantum dots: an analytical and green chemistry approach†
Received
29th October 2023
, Accepted 22nd January 2024
First published on 29th January 2024
Abstract
This study presents the development of an eco-friendly and highly selective mitrogen-doped carbon quantum dot based sensor (N-CQDs) for the detection of gabapentin – a commonly misused drug. A detailed characterization of N-CQDs spectral features and their interaction with gabapentin is provided. The optimal conditions for sensing, including pH value, buffer volume, N-CQDs concentration, and incubation time, were established. The results showed excellent fluorescence quenching at 475 nm (λex = 380 nm) due to the dynamic quenching mechanism, and the sensor demonstrated excellent linearity in the 0.5–8.0 μg mL−1 concentration range with correlation coefficients of more than 0.999, a limit of detection (LOD) of 0.160 and limit of quantification (LOQ) of 0.480 μg mL−1. The accuracy of the proposed sensor was acceptable with a mean accuracy of 99.91 for gabapentin detection. In addition, precision values were within the acceptable range, with RSD% below 2% indicating good repeatability and reproducibility of the sensor. Selectivity was validated using common excipients and pooled plasma samples. The proposed sensor accurately estimated gabapentin concentration in commercial pharmaceutical formulations and spiked plasma samples, exhibiting excellent comparability with previously published methods. The 'greenness' of the sensing system was evaluated using the Analytical GREEnness calculator, revealing low environmental impact and strong alignment with green chemistry principles with a greenness score of 0.76. Thus, the developed N-CQDs-based sensor offers a promising, eco-friendly, and effective tool for gabapentin detection in various situations, ranging from clinical therapeutics to forensic science.
1. Introduction
Carbon quantum dots are fluorescent nanoparticles that have gained tremendous attention in various fields due to their unique properties.1,2 They are characterized by their good biocompatibility, bright luminescence, high hydrophilicity, low cytotoxicity, and tunable photoluminescence.3,4 These properties make them highly versatile and suitable for numerous applications including bioimaging, drug delivery, tumour diagnosis, catalysis, sensors, and even in cancer therapy.5–9 Furthermore, carbon quantum dots are known for their low toxicity and low cost of production, making them an attractive alternative to other types of quantum dots such as semiconductor quantum dots or commercial organic dyes.10 The synthesis of carbon quantum dots can be achieved through two main methods: the “top-down” method and the “bottom-up” method. Traditional top-down techniques involve modifying existing carbon materials like graphite or carbon nanotubes and bottom-up approaches such as hydrothermal and microwave-assisted methods have gained popularity.11 However, these methods often rely on chemical precursors that are incompatible with green analytical chemistry principles.12 In recent years, there has been a shift towards environmentally friendly methods for the synthesis of carbon quantum dots.13 To address this issue, researchers have focused on finding alternative precursors from natural sources to replace conventional ones. Biomass waste and plant-derived pharmaceuticals are particularly attractive in carbon quantum dots synthesis due to their environmental friendliness, wide availability, biocompatibility, and abundance of low-cost renewable raw materials rich in C (carbon), H (hydrogen), N (nitrogen), and O (oxygen) atoms.14 Hence, these green quantum dots have been applied extensively for the analysis of various pharmaceuticals via determining their unique optical features and exploring their potential as fluorescent probes.15–17
Gabapentin is a medication approved by the U.S. Food and Drug Administration for the treatment of seizures and nerve pain.18 It is structurally similar to the neurotransmitter gamma-aminobutyric acid but does not directly bind to GABA receptors.19 Instead, it works by modulating the activity of calcium channels in the brain, which helps to reduce the excessive nerve activity associated with seizures and neuropathic pain.19 However, gabapentin has also been misused and abused for recreational purposes.20 The misuse of gabapentin has become a growing concern, as it can lead to various adverse effects such as respiratory depression, sedation, and addiction.21 Notably in many countries, including Egypt and Saudi Arabia, gabapentin is listed as a controlled substance due to its potential for abuse.22
The current methods for detecting gabapentin include high-performance liquid chromatography (HPLC) and capillary electrophoresis (CE) with UV or fluorescence detection.23–25 In addition, gas chromatography-tandem mass spectrometry (GC-MS/MS), and liquid chromatography-tandem mass spectrometry (LC-MS/MS) have also been reported for gabapentin analysis.26,27 However, these techniques have drawbacks such as the need for derivatization to create a detectable chromophore or they often require expensive equipment, skilled operators, and time-consuming sample preparation procedures. Alternative methods like UV visible spectrophotometry28–30 and spectrofluorimetry31–33 have been also explored for gabapentin analysis but still face limitations due to the extra derivatization step required for gabapentin detection due to the lack of chromophoric group. To overcome these challenges, there is a growing interest in developing more accessible, cost-effective, and rapid detection methods for gabapentin that do not require additional steps like derivatization.
The present work aims to develop a novel green, fluorescent probe based on N-doped carbon quantum dots (N-CQDs) for the detection of gabapentin in pharmaceutical dosage forms and biological fluids. Optimization of the experimental condition has been performed to enhance the sensitivity of the N-CQDs probe for gabapentin detection. Additionally, validation of the developed method has been conducted as per ICH guidelines to ensure the linearity, accuracy, precision, and selectivity of the developed method. Aside, greenness evaluation has also been studied using the Analytical GREEnness (AGREE) tool which is based on the twelve principles of green analytical chemistry to assess the environmental impact of the developed method.
2. Experimental
2.1. Materials, reagents, and standard solutions
Gabapentin, with a confirmed purity of 99.5%, was obtained from the Egyptian Drug Authority and used as is without any additional purification. Conventin® capsules of 400 mg per capsule made by Eva pharma company and Gabapentin® film-coated tablets of 800 mg per tablet manufactured by UNI pharma were purchased from local pharmacies. All chemicals employed in the analysis were of highly pure analytical grade acquired from El Nasr Company. Fresh double distilled water was used for the procedure. Various buffer solutions with different pH levels were prepared following guidelines outlined in the US Pharmacopoeia. The synthesis of the N-CQDs from the guava fruit was conducted following the exact same procedure described in our previous work.16 Briefly, the flesh of the guava fruit (free from pulp and seeds) was sliced and subjected to carbonization in an electric oven at 250 °C for 50 minutes. After cooling, the resulting product was finely ground into a powder. A quantity corresponding to 200 mg of the powdered product was dissolved in 60 mL double-distilled water, boiled for 10 minutes, and then centrifuged for another 20 minutes. The yellow N-CQDs solution obtained after filtration was transferred into a 100 mL volumetric flask and filled with double-distilled water up to the required volume (Fig. S1†).
A stock standard solution of gabapentin was prepared at a concentration of 100 μg mL−1 by dissolving an appropriate amount of gabapentin in double distilled water. Then, a working solution of gabapentin in the concentration of 20 μg mL−1 was prepared by appropriate dilution of the stock solution.
2.2. Instrumentations
A Jasco FP-6200 spectrofluorometer with a 1 cm cell and excitation and emission monochromators slit widths of 5 nm and 10 nm, respectively, was utilized for fluorescence measurements. A Shimadzu UV-visible 1800 spectrophotometer was used for measuring the UV-visible absorption spectra.
2.3. Procedure for calibration graph
To create the calibration graph, a range of standard solutions containing different concentrations of gabapentin (ranging from 0.5 to 8 μg mL−1) were prepared. Each solution was made by adding appropriate volumes of the working solution to separate 10 mL volumetric flasks that already contained 1 mL of N-CQDs solution and 1.5 mL of borate buffer solution at pH 8. After allowing the solutions to incubate for 5 minutes, they were thoroughly mixed, and the final volume was adjusted with double distilled water. The fluorescence intensities (F) of these solutions were measured using an excitation wavelength of 380 nm and an emission wavelength of 475 nm. A blank sample was also prepared under the same identical conditions and the fluorescence intensity of this blank sample was recorded (F0). By plotting (F0/F) against the drug concentrations in μg mL−1, the calibration curve was constructed.
2.4. Validation of the developed N-CQDs-based sensor for gabapentin detection
Validation of the developed N-CQDs sensor for detecting gabapentin was performed in accordance with the ICH34 and ICHM10 (ref. 35) guidelines. Linearity was evaluated using seven different concentrations of gabapentin with the same procedures previously described under Section 2.3. The resulted calibration curve was analyzed to obtain the value of correlation coefficient (R2), slope, and y-intercept. Limits of detection (LOD) and quantification (LOQ) were determined based on the standard deviation of the response as calculated from the residual and the slope of the calibration curve based on the below equation:
LOD = 3 × (σ/S) and LOQ = 10 × (σ/S), where σ is the standard deviation of the response and S is the slope of the calibration curve.
The accuracy and precision of the developed N-CQDs sensor were assessed by comparing the measured concentrations of gabapentin in samples with their known concentrations. The accuracy was measured by analyzing three replicates of three gabapentin concentration levels (2, 4 and 6 μg mL−1) and calculating the percentage recovery. The precision of the developed sensor was evaluated by calculating the relative standard deviation (RSD%) of the measured concentrations for each level of gabapentin (2, 4 and 6 μg mL−1) under the specified conditions either in the same day (repeatability) or in three consecutive days (intermediate precision). Moreover, robustness of the developed N-CQDs sensor was assessed by evaluating the effect of small variations in experimental conditions, such as pH (±0.25), buffer volume (±0.1 mL) and incubation time (±1 min).
The selectivity of the developed N-CQDs sensor for gabapentin was evaluated by measuring its response in the presence of some interfering substances that may be present in its formulation. Besides, the plasma matrix effect was also examined as per ICHM10 guidelines to ensure that the presence of other compounds in plasma does not interfere with the detection and quantification of gabapentin using the N-CQDs sensor.
2.5. Procedure for analysis of different pharmaceutical dosage forms
The analysis of different pharmaceutical dosage forms such as tablets and capsules containing gabapentin was conducted using the developed method. The contents of five tablets or capsules were accurately weighed and triturated to obtain a fine powder. Then, an accurately weighed amount of the powder equivalent to 10 mg of gabapentin was transferred into a 100 mL volumetric flask. Next, 50 mL of double distilled water was added to the flask and sonicated for 30 minutes to ensure complete dissolution of the drug. After sonication, the solution was filtered and appropriately diluted to obtain a working concentration of 20 μg mL−1. The same working procedure was followed as described previously in creating the calibration graph using the working solution. Then, the fluorescence intensity of the sample solutions was measured using the same excitation and emission wavelengths as mentioned before. The content of gabapentin in tablets or capsules was determined from the calibration curve by applying the corresponding regression equation.
2.6. Procedure for analysis of spiked human plasma
One milliliter aliquots of human plasma samples were spiked with increasing amounts of a gabapentin standard solution (20 μg mL−1). The mixture was vortexed for one minute in centrifugation tubes. To precipitate the plasma proteins, 5 mL of acetonitrile was added, and the tubes were vortexed for about 30 seconds. After centrifuging at 3600 rpm for approximately 30 minutes, the clear supernatant was filtered using syringe filters. The filtrate was then dried using a rotary evaporator and the residues were dissolved in minimal water before being transferred to 10 mL volumetric flasks. Next, each flask was filled with 1 mL of N-CQDs solution and mixed with 1.5 mL of borate buffer solution (pH8) before being diluted to volume with water. A similar procedure without any analyte present was carried out as a control blank sample. After preparing the solutions, the fluorescence intensity of the samples was measured using the same excitation and emission wavelengths as mentioned before and the concentration of gabapentin in the spiked human plasma samples was determined using the corresponding regression equation.
3. Results and discussion
3.1. Spectral characteristics of the synthesized quantum dots
The fluorescence and UV-vis techniques were used to examine the spectral characteristics of the synthesized N-doped carbon quantum dots (N-CQDs) in order to assess their suitability for gabapentin detection. The UV-vis spectrum of the N-CQDs (Fig. 1A) exhibits a characteristic absorption peak at 283 nm, with an extended tail into the visible region representing the π–π* and n–π* transition of C
C and C
O bonds. In terms of emission, the N-CQDs displayed a potent emission at a maximum wavelength of 475 nm when excited at 380 nm (Fig. 1B). Furthermore, the intensity of emission peaks exhibited by the current N-CQDs is found to be strongly reliant on excitation wavelengths. Fig. 1C demonstrates that as the excitation wavelength increased from 380 nm to 450 nm, there was a decrease in peak intensity and a shift towards higher wavelengths. Consequently, all experiments were conducted with an excitation wavelength of 380 nm. Additionally, when compared to quinine sulfate as the calculated reference standard, it was determined that the fluorescence quantum yield of the obtained N-CQDs was approximately 26%.
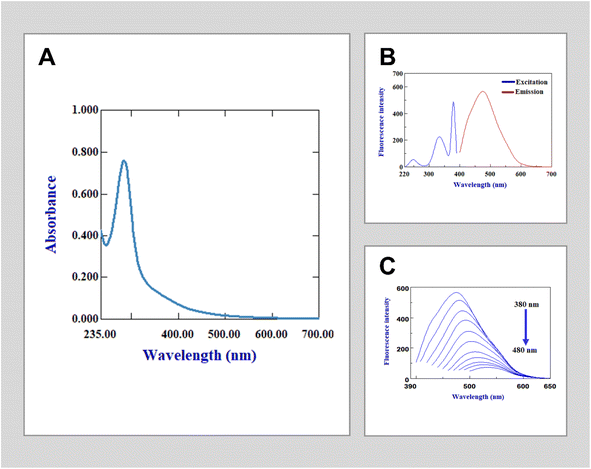 |
| Fig. 1 Spectral features of the developed N-CQDs including (A) UV-vis absorption spectrum of the N-CQDs showing λmax at 283 nm. (B) Excitation and emission fluorescence spectra showing a potent emission at a λmax of 475 nm when excited at 380 nm. (C) Excitation-dependent emission spectra of N-CQDs which show a decrease in the emission intensity as the excitation wavelengths increase. | |
3.2. Sensing principles of gabapentin based on N-CQDs
The fluorescence characteristics of N-CQDs are altered upon interaction with gabapentin, resulting in a quantitative decrease in their fluorescence intensity (Fig. 2A), a similar effect that was also observed previously in the interaction of CQDs with many compounds.7,8,11 Various mechanisms, such as static quenching, dynamic quenching, or the inner filter effect, may contribute to these interactions. In order to investigate and analyze the fluorescence quenching observed in the presence of gabapentin, additional research was conducted. The UV-vis spectrum of gabapentin was examined and found to have no absorbance beyond 300 nm, indicating that the observed fluorescence quenching cannot be attributed to the inner filter effect caused by gabapentin's absorption properties. To determine whether the quenching mechanism is dynamic or static, a temperature dependence experiment was performed where increasing temperatures would lead to an increase in diffusion coefficient and energy transfer for dynamic quenching, while for static quenching, as the temperature rises, there would be a decrease in the rate constant of quenching due to decreased stability of non-fluorescent complex. These mechanisms can be investigated using the Stern–Volmer equation which relates the fluorescence intensity of a fluorophore to the concentration of the quencher. The Stern–Volmer analysis of the fluorescence quenching of N-CQDs in the presence of gabapentin revealed that the quenching mechanism is likely dynamic as the slope increases with increasing temperature (Fig. 2B).
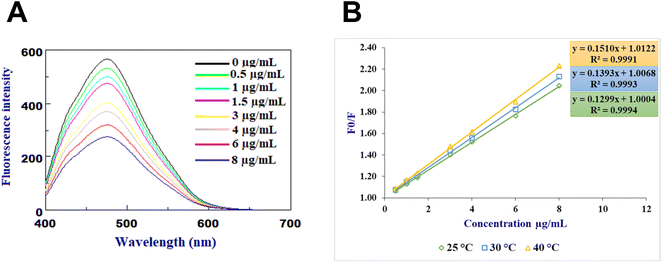 |
| Fig. 2 (A) Emission fluorescence spectra obtained from the quenching reaction of N-CQDs with different concentrations of gabapentin, and (B) Stern–Volmer plots of the gabapentin interaction with the developed N-CQDs at different temperatures. | |
3.3. Optimization of sensing conditions
Before conducting the quantitative analysis of gabapentin using the recommended probe, multiple experiments were conducted to optimize the detection conditions including pH value, buffer volume, concentration of N-CQDs used in the sensor, and incubation time. Various buffers at different pH values were tested, including phthalate buffer (pH 3.0–5.0), phosphate buffer (pH 6.0–7.5) and borate buffer (pH 8.0–10.0). Among these buffers, the borate buffer at pH 8 was found optimum with uniform fluorescence intensity and minimal interference from other factors (Fig. 3A). It is worth noting that the pH value of the buffer greatly affects the stability and fluorescence intensity of the sensor, as well as the binding affinity between gabapentin and N-CQDs. Lower pH values were observed to lower the quenching efficiency and fluorescence intensity of the developed sensor, while higher pH values >10 led to decreased stability and increased background interference. Hence, the selection of borate buffer with a pH value of 8 was crucial for achieving consistent and reliable results in the gabapentin detection process. Consequently, the volume of the added buffer was also studied revealing that a buffer volume of 1.5 mL was suitable for achieving a stable and consistent fluorescence signal (Fig. 3B). The concentration of N-CQDs used in the sensor was also optimized, and it was found that 1 mL of the N-CQDs solution (containing 200 μg mL−1 N-CQDs) provided the best fluorescence quenching efficiency (Fig. 3C). Additionally, the incubation time between N-CQDs and gabapentin was investigated to determine the duration that would result in optimal quenching. Based on the research conducted, an incubation time of 5 minutes was found to yield the highest fluorescence quenching efficiency (Fig. 3D).
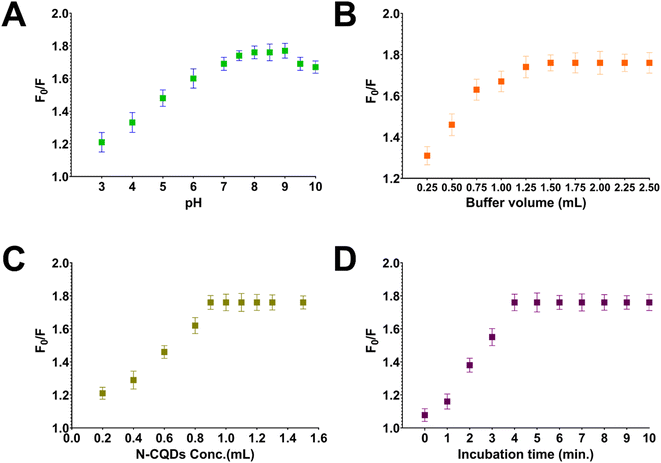 |
| Fig. 3 Optimization of experimental parameters influencing the stability of fluorescence quenching of N-CQDs by gabapentin including (A) buffer pH, (B) buffer volume, (C) N-CQDs volume, and (D) incubation time. The error bars represent the standard deviation of three independent measurements. | |
3.4. Method validation
The method validation of the proposed N-CQDs-based sensor for the detection of gabapentin was investigated (Table 1). The sensor exhibited good linearity in the concentration range of 0.5–8.0 μg mL−1 with a correlation coefficient (R2) value of 0.9994. The limits of detection (LOD) and quantification (LOQ) were found to be 0.1600 and 0.4801 μg mL−1, respectively. The results of this sensor transcended some reported methods for gabapentin detection, particularly the spectrophotometric methods,28,30 in terms of sensitivity and linearity. Furthermore, the linearity and sensitivity of the developed sensor were comparable to some chromatographic methods and reported spectrofluorometric methods without the need for the extra derivatization reactions for gabapentin detection.27,31
Table 1 Validation data for determination of gabapentin by the developed N-CQDs fluorescent probe
Parameters |
Gabapentin |
Average of 9 determinations (3 concentrations repeated 3 times). RSD% of 9 determinations (3 concentrations repeated 3 times) measured in the same day. RSD% of 9 determinations (3 concentrations repeated 3 times) measured in the three consecutive days. |
Excitation wavelength (nm) |
380 |
Emission wavelength (nm) |
475 |
Linearity range (μg mL−1) |
0.5–8.0 |
Slope |
0.1299 |
Intercept |
1.0004 |
Correlation coefficient (R2) |
0.9994 |
LOD (μg mL−1) |
0.1600 |
LOQ (μg mL−1) |
0.4801 |
Accuracy (R%)a |
99.91 ± 1.580 |
Repeatability precision (RSD%)b |
0.875 |
Intermediate precision (RSD%)c |
1.721 |
The accuracy of the proposed N-CQDs-based sensor for gabapentin detection was evaluated by comparing the measured concentrations of gabapentin with their known concentrations. The results showed that the sensor achieved high accuracy, with percentage recoveries ranging from 99.74 to 100.07 with mean accuracy of 99.91. The precision of the sensor was also assessed via repeatability and intermediate precision experiments. The repeatability experiment, performed within the same day, yielded a RSD% value of less than 2% for all gabapentin concentration levels. The intermediate precision experiment, conducted over three consecutive days, also demonstrated a RSD% value below 2% for all gabapentin concentration levels. Detailed results of the accuracy and precision evaluation of the proposed N-CQDs-based sensor for gabapentin detection can be found in Table S1.†
Robustness studies were also conducted to assess the impact of small variations in experimental conditions on the performance of the proposed N-CQDs-based sensor for gabapentin detection. These studies revealed that the method remained robust and reliable, as small changes in parameters such as pH, buffer volume, and reaction time did not significantly affect the accuracy of gabapentin detection using the N-CQDs-based sensor (Table S2†).
A critical aspect of quantum dots validation is the selectivity of the synthesized probe. In the case of detecting gabapentin, the selectivity of the N-CQDs-based sensor was evaluated by studying the interference from commonly co-existing excipients in the formulation of gabapentin. The results of this evaluation demonstrated that the proposed sensor exhibited excellent selectivity, as no noticeable interference was observed from common excipients present in gabapentin formulations such as starch, lactose, and magnesium stearate (Fig. 4). Aside, to ensure the applicability of the method in the biological fluids, the selectivity of the N-CQDs-based sensor was also tested against pooled plasma samples from different individuals. The results showed that the proposed sensor was highly selective for gabapentin detection in plasma samples, with no interference observed from other components present in the samples (Fig. 4). However, the developed sensor may face challenges in terms of selectivity and specificity when exposed to other drugs that share similar functional groups, like risperidone.16 Cross-reactivity with N-CQDs-based sensors is a known concern and to tackle this issue in future research, the sensor could be functionalized36 or doped with gabapentin analogs or analog-specific recognition elements, to enhance its selectivity and mitigate potential cross-reactivity with structurally similar drugs. Additionally, molecular imprinting techniques37 could be employed to establish specific recognition sites for gabapentin within the N-CQDs, thus improving the sensor's selectivity towards gabapentin and reducing potential cross-reactivity with structurally similar drugs.
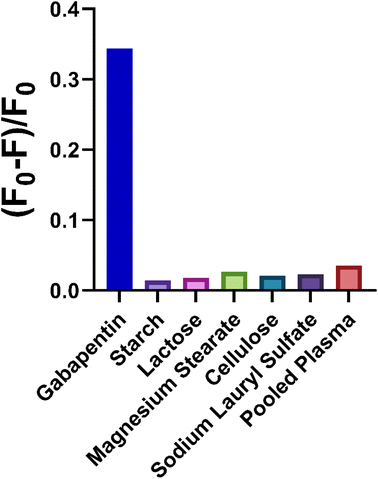 |
| Fig. 4 The competitive selectivity experiments showing the fluorescence quenching factor [(F0 − F)/F0] responses of the developed N-CQDs interaction with gabapentin and various interfering compounds in addition to plasma samples. | |
The plasma matrix effect has also been calculated as per ICHM10 guidelines. Such guideline has been developed to provide recommendations on the validation of bioanalytical assays for chemical and biological drugs and their metabolites in biological matrices.35 The procedures for conducting the matrix effect involve spiking two concentration levels of gabapentin to blank plasma samples from different individuals, and the results indicated that there was no significant matrix effect observed, suggesting that the proposed N-CQDs-based sensor can provide accurate detection of gabapentin in complex biological matrices (Table S3†).
3.5. Application of the proposed sensor for gabapentin analysis in pharmaceutical dosage form and spiked human plasma
The proposed N-CQDs-based sensor was successfully applied for the analysis of gabapentin in pharmaceutical dosage forms. The results showed that the concentrations of gabapentin in the commercial formulations were within the expected range (100.09–100.29%), demonstrating the accuracy and reliability of the sensor for real sample analysis (Table 2). In addition, comparing the results with a previously published spectrophotometric method,29 the proposed sensor showed no significant statistical difference in mean and variance, indicating its potential as a reliable method for gabapentin analysis in pharmaceutical dosage forms.
Table 2 Quantitative analysis of gabapentin in the commercial Conventin® capsules and Gabapentin® film-coated tablets by the proposed methods and statistical comparison with the reported method
|
N-CQDs probe |
Reported method24 |
Conventin®capsules |
Gabapentin®tablets |
Conventin®capsules |
Gabapentin®tablets |
Average of five determinations. The values in parenthesis are tabulated values of “t“and “F” at (P = 0.05). |
(Recovery% ± RSD%)a |
100.29 ± 1.58 |
100.09 ± 1.16 |
99.80 ± 1.61 |
99.54 ± 1.23 |
t-test (2.306)b |
0.491 |
0.729 |
|
F-test (6.388)b |
1.027 |
1.110 |
Furthermore, the applicability of the N-CQDs-based sensor was tested for gabapentin detection in spiked human plasma at different concentration levels (Table 3). The results of this analysis demonstrated that the proposed sensor was able to accurately detect gabapentin in spiked human plasma samples, without any noticeable interference from other components present in the samples. Overall, the proposed N-CQDs-based sensor showed excellent selectivity and accuracy for the detection of gabapentin in pharmaceutical dosage forms and spiked human plasma samples, making it a promising tool for the analysis of this misused drug in both clinical and forensic samples.
Table 3 Application of the proposed method for the determination of gabapentin in spiked plasma samples
Amount added |
Amount found |
Recovery (%) |
0.5 |
0.56 |
112.49 |
2 |
2.15 |
107.27 |
4 |
4.24 |
105.90 |
7 |
7.52 |
107.49 |
8 |
8.16 |
101.95 |
Mean ± RSD% |
107.02 ± 3.53 |
3.6. Greenness evaluation of the proposed sensor and eco-impact assessment of the method
In addition to its analytical performance, the proposed N-CQDs-based sensor also offers several advantages in terms of its eco-friendliness. The preparation of the sensor from plant material not only reduces the reliance on synthetic materials but also minimizes the generation of hazardous waste. Aside, using water as a solvent in all the method procedures eliminates the need for organic solvents that are harmful to the environment. In order to assess such impact, the Analytical GREEnness calculator (AGREE) was used to evaluate the environmental impact of the proposed sensor.38 This tool is based on the 12 principles of green analytical chemistry, which aim to minimize the use and generation of hazardous substances in chemical processes. In addition, the tool assesses factors such as waste generation, energy consumption, and resource utilization. The results of the greenness evaluation indicated that the proposed N-CQDs-based sensor has a low environmental impact achieving a score of 0.76 and is considered a green analytical method (Fig. 5).
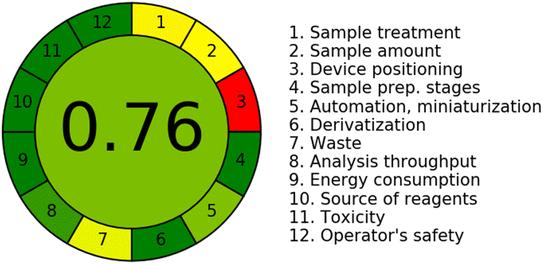 |
| Fig. 5 The analytical greenness score obtained by AGREE software for the proposed spectrofluorimetric method. | |
4. Conclusion
In conclusion, this study offered the effective development of a N-CQDs sensor capable of precisely detecting gabapentin without any extra derivatization or use of chromogenic reagent procedures. Demonstrating commendable selectivity and accuracy, the sensor accurately measured gabapentin concentrations in varying contexts, such as commercial pharmaceutical formulations and plasma samples spiked with gabapentin. Moreover, it successfully adhered to the principles of green chemistry, positioning it as an environmentally considerate analytical method. However, certain limitations i.e., the conductance of the analysis under restricted validation against certain common gabapentin excipients and plasma components, dictate the need for further research for enhancing method selectivity and specificity. Future prospects involve the extension of these findings to real-world environments and inclusion of more comprehensive potential interfering substances. Additionally, investigating the potential of N-CQDs in detecting other misused drugs could amplify the application spectrum of this nascent, green sensing technology. Therefore, the N-CQDs-based sensor shows immense promise as a vital, eco-friendly tool for comprehensive drug detection in clinical therapeutics and forensic science.
Conflicts of interest
There are no conflicts of interest to declare.
Acknowledgements
We would like to thank Princess Nourah bint Abdulrahman University Researchers Supporting Project number (PNURSP2024R466), Princess Nourah bint Abdulrahman University, Riyadh, Saudi Arabia.
References
- Y. Wang and A. Hu, J. Mater. Chem. C, 2014, 2, 6921–6939 RSC.
- J. Shen, Y. Zhu, X. Yang and C. Li, Chem. Commun., 2012, 48, 3686–3699 RSC.
- F. Yuan, S. Li, Z. Fan, X. Meng, L. Fan and S. Yang, Nano Today, 2016, 11, 565–586 CrossRef.
- T. Dutta, O. Chatterjee, B. Chakraborty and A. L. Koner, in Carbon Quantum Dots for Sustainable Energy and Optoelectronics, ed. S. K. Batabyal, B. Pradhan, K. Mohanta, R. R. Bhattacharjee and A. Banerjee, Woodhead Publishing, 2023, pp. 1–28, DOI:10.1016/B978-0-323-90895-5.00015-1.
- S. N. Baker and G. A. Baker, Angew. Chem., Int. Ed., 2010, 49, 6726–6744 CrossRef PubMed.
- N. Dubey, S. Dhiman and A. L. Koner, ACS Appl. Nano Mater., 2023, 6, 4078–4096 CrossRef.
- N. Dubey, S. Ramteke, N. K. Jain, T. Dutta and A. Lal Koner, ChemistrySelect, 2022, 7, e202201604 CrossRef.
- N. Dubey, S. Ramteke, N. K. Jain, T. Dutta and A. L. Koner, New J. Chem., 2023, 47, 16390–16398 RSC.
- S. Mallick, P. Kumar and A. L. Koner, ACS Appl. Nano Mater., 2019, 2, 661–666 CrossRef.
- S. Zhu, Q. Meng, L. Wang, J. Zhang, Y. Song, H. Jin, K. Zhang, H. Sun, H. Wang and B. Yang, Angew. Chem., Int. Ed., 2013, 52, 3953–3957 CrossRef CAS.
- H.-L. Yang, L.-F. Bai, Z.-R. Geng, H. Chen, L.-T. Xu, Y.-C. Xie, D.-J. Wang, H.-W. Gu and X.-M. Wang, Mater. Today Adv., 2023, 18, 100376 CrossRef.
- H. H. Jing, F. Bardakci, S. Akgöl, K. Kusat, M. Adnan, M. J. Alam, R. Gupta, S. Sahreen, Y. Chen, S. C. B. Gopinath and S. Sasidharan, J. Funct. Biomater., 2023, 14, 27 CrossRef.
- M. Kurian and A. Paul, Carbon Trends, 2021, 3, 100032 CrossRef.
- R. Das, R. Bandyopadhyay and P. Pramanik, Mater. Today Chem., 2018, 8, 96–109 CrossRef.
- X. Jiang, D. Qin, G. Mo, J. Feng, C. Yu, W. Mo and B. Deng, J. Pharm. Biomed. Anal., 2019, 164, 514–519 CrossRef.
- S. I. Alaqel, O. Abdullah, A. Alharbi, Y. S. Althobaiti, M. S. Alturki, S. Ramzy and A. H. Almalki, RSC Adv., 2023, 13, 17765–17774 RSC.
- S. l. Alaqel, M. A. Algarni, A. Alharbi, A. H. Almalki, M. S. Alzahrani, M. H. Abdelazim and A. H. Abdelazim, Spectrochim. Acta, Part A, 2024, 304, 123418 CrossRef CAS PubMed.
- W. J. Curry and D. L. Kulling, Am. Fam. Physician, 1998, 57, 513–520 Search PubMed.
- G. J. Sills, Curr. Opin. Pharmacol., 2006, 6, 108–113 CrossRef PubMed.
- R. V. Smith, J. R. Havens and S. L. Walsh, Addiction, 2016, 111, 1160–1174 CrossRef PubMed.
- G. C. Quintero, J. Exp. Pharmacol., 2017, 13–21 CrossRef PubMed.
- A. A. Alkhalaf, R. A. Bukhari, E. A. Alshehri, S. O. Alshehri and A. F. Badr, J. Taibah Univ. Medical Sci., 2021, 16, 700–705 Search PubMed.
- A. M. Zeid, N. Kaji, J. J. M. Nasr, F. F. Belal, Y. Baba and M. I. Walash, J. Chromatogr. A, 2017, 1503, 65–75 CrossRef PubMed.
- A. M. Zeid, J. J. M. Nasr, F. Belal, M. I. Walash, Y. Baba and N. Kaji, Spectrochim. Acta, Part A, 2021, 246, 119021 CrossRef CAS PubMed.
- S. T. Ulu and E. Kel, J. Chromatogr. Sci., 2011, 49, 417–421 CAS.
- F. Kolocouri, Y. Dotsikas and Y. L. Loukas, Anal. Bioanal. Chem., 2010, 398, 1339–1347 CrossRef CAS PubMed.
- C. Gambelunghe, G. Mariucci, M. Tantucci and M. V. Ambrosini, Biomed. Chromatogr., 2005, 19, 63–67 CrossRef CAS.
- S. A. Abdulrahman and K. Basavaiah, Drug Test. Anal., 2011, 3, 748–754 CrossRef.
- A. A. Gouda and Z. A. Malah, Spectrochim. Acta, Part A, 2013, 105, 488–496 CrossRef PubMed.
- W. Winotapun, K. Kongpakwattana, S. Dejpittayanunt, S. Pathomcharoensukchai, U. Suksaran, N. Nuntharatanapong and T. Rojanarata, Talanta, 2012, 99, 997–1003 CrossRef PubMed.
- E. M. Hassan, F. Belal, O. A. Al-Deeb and N. Y. Khalil, J. AOAC Int., 2001, 84, 1017–1024 CrossRef.
- F. Belal, H. Abdine, A. Al-Majed and N. Y. Khalil, J. Pharm. Biomed. Anal., 2002, 27, 253–260 CrossRef PubMed.
- T. Z. Attia, M. Elnady and S. M. Derayea, RSC Adv., 2019, 9, 29942–29948 RSC.
- ICH Harmonised Tripartite Guideline, Q2 (R1), 2005, vol. 1, p. 5 Search PubMed.
- ICH Harmonised Guideline, Geneva, Switzerland, 2022 Search PubMed.
- M. E. El Sharkasy, M. M. Tolba, F. Belal, M. I. Walash and R. Aboshabana, RSC Adv., 2022, 12, 13826–13836 RSC.
- A. A. Ensafi, P. Nasr-Esfahani and B. Rezaei, Sens. Actuators, B, 2018, 257, 889–896 CrossRef.
- F. Pena-Pereira, W. Wojnowski and M. Tobiszewski, Anal. Chem., 2020, 92, 10076–10082 CrossRef.
|
This journal is © The Royal Society of Chemistry 2024 |
Click here to see how this site uses Cookies. View our privacy policy here.