DOI:
10.1039/D3RA07433A
(Paper)
RSC Adv., 2024,
14, 382-389
Effect of choline amino acid ionic liquids on maize seed germination and endogenous plant hormone levels†
Received
1st November 2023
, Accepted 11th December 2023
First published on 5th January 2024
Abstract
Prior research has established choline-based ionic liquids (ILs) as safe for various organisms. However, their impact on plants has been underexplored. To identify effective eco-friendly ILs, we synthesized seven choline amino acid ([Chl][AA]) ILs and analyzed their physiological influence on maize seed germination. In contrast to the traditionally used N-octyl pyridinium bromide IL, these seven [Chl][AA] ILs exhibited substantially lower toxicity. Moreover, within a broad treatment concentration range (10–100 mg L−1), these ILs notably enhanced maize germination indices and root and shoot growth. Specifically, treatment with 100 mg L−1 choline tryptophan resulted in a 21.2% increase in germination index compared to those of control maize. Compared to the control, the application of choline serine, choline aspartic acid, choline phenylalanine, and choline tryptophan at 100 mg L−1 led to respective increases of 23.9%, 21.5%, 22.5%, and 24.5% in maize shoot length. Analysis of endogenous hormones and free amino acid contents revealed elevated levels of growth-promoting plant hormones (gibberellic acid and zeatin) in maize shoot tips, as well as increased contents of major amino acids (glutamate, glycine, and arginine) following treatment with different [Chl][AA] ILs at 100 mg L−1. These findings indicate that [Chl][AA] holds promise for the development and application of novel low-toxicity ILs.
1 Introduction
Ionic liquids (ILs) have been widely studied across various fields, including chemical synthesis, pharmaceutics, clean energy, environmental protection, and the food industry.1–4 However, owing to the potential environmental risks associated with conventional imidazolium- and pyridinium-based ILs, there is a current surge in interest regarding the synthesis and application of environmentally friendly or “green” ILs.5
Choline-based ILs, which are derived from natural renewable raw materials, such as sugars, amino acids, and choline, have been extensively studied.6,7 Among these, natural amino acids and their derivatives provide the most abundant renewable natural chiral pool, making them ideal precursor materials for the framework of chiral ILs from both environmental and economic standpoints.8 In addition, amino acids exhibit amphoteric electrolyte behavior, i.e., they can function as either cations or anions during the synthesis of ILs.9 Furthermore, the combination of choline and amino acids led to the formation of choline amino acid ([Chl][AA]) ILs, which demonstrated potential properties for applications in organic synthesis and biomass pretreatment.10,11 In this regard, using a simple and relatively greener route, Hu et al. synthesized [Choline][Pro] from choline chloride and (L)-proline, which served as an effective catalyst for direct aldol reactions between ketones and aromatic aldehydes in water at room temperature.12 Liu et al. and Hou et al. synthesized a series of [Chl][AA] ILs and characterized their potential application in degrading lignin. These choline-based ILs also functioned as highly effective solvents for pretreating lignocellulosic biomass, such as wheat or rice straw, via selective lignin removal.13,14 Moreover, previous studies have demonstrated that [Chl][AA] ILs could be classified as “readily biodegradable” based on their high levels of mineralization and low toxicity towards acetyl cholinesterase, bacteria, and fish cell lines.14,15 However, relatively few studies have examined their effects on plants.
Choline analogs have been widely adopted as plant growth regulators.16,17 For example, choline chloride can undergo conversion into phosphatidylcholine and betaine in plant tissues, thereby contributing to the maintenance of biomembrane stability.18 Chlormequat chloride also functions as a plant growth regulator that can shorten and thicken stems.19 Exogenous glycine betaine can increase osmotic stress tolerance by reducing lipid peroxidation in different plants.20 In addition, exogenous free amino acids can facilitate a more rapid accumulation of seed protein and improve germination rate.21,22 Considering the effects of choline analogs and amino acids on plant growth, it is reasonable to anticipate that [Chl][AA] ILs might hold considerable potential in promoting plant germination and growth.
To explore this potential, we sought to investigate the effect of seven [Chl][AA] ILs on maize seed germination and early seedling growth in this study. As a negative control, we also examined the effects of treatment with N-octyl pyridinium bromide ([OPy][Br]), a conventionally used IL. We believe that the findings of this study will contribute significantly to an overall assessment of the ecotoxicity of green ILs derived from natural renewable raw materials and thereby serve as a basis for expanding the scope of their application.
2 Materials and methods
2.1. Chemicals and plant materials
Choline hydroxide (45 wt% in H2O) was purchased from Sigma-Aldrich, St. Louis, MO, USA. Amino acids and tetramethylsilane were obtained from J&K Scientific Ltd., Beijing, China. Details on the preparation and characterization of [Chl][AA] ILs are provided in ESI Fig. S1.† [OPy][Br] IL was purchased from the Lanzhou Institute of Chemical Physics, Chinese Academy of Sciences, China. The maize seeds (Zhengdan958, Zea mays L.) used in this study were purchased from Doneed Seeds Ltd., Beijing, China.
2.2. Seed treatment and germination
Seed germination requires suitable conditions and environmental factors. Moderate temperatures around 26–29 °C and adequate water are required for a maize seed to germinate.23 Based on the study of maize seed germination,24 maize seeds were subjected to surface sterilization through immersion in 75% ethanol for 3 min, followed by thorough rinsing with deionized water until clean. After draining, the seeds were immersed in different IL solutions at 28 °C for 12 h, after which they were transferred to a growth chamber (13 cm × 19 cm × 10 cm) on three layers of filter paper. In each chamber, 24 seeds were sown with two replications. Different ILs were dissolved in distilled water. Initially, 30 mL of deionized water (control) or IL solutions of different concentrations were introduced into each chamber. Over the following 4 days, 5 mL of these solutions were added daily. Maize seeds were germinated on moistened, clean filter paper at 28 °C in the dark for 6 days. The number of germinated seeds was counted daily; the seeds were considered to have germinated when approximately 5 mm of the primary root had emerged. The experiment was repeated thrice.
2.3. Germination assay
During the 6 day (cultured for 144 h) germination period, the number of germinated seeds was counted daily, and the germination rate, potential, and index were calculated using the following equations:
Germination rate (%) = (number of germinated seeds at 5 d/total number of test seeds) × 100 |
Germination potential (%) = (number of germinated seeds at 3 d/total number of test seeds) × 100 |
Germination index = ∑GT/dt, where GT is the number of germinated seeds on day t, and dt is the germination days |
A total of 12 measurements were taken for shoot and root lengths per germination test. Next, the shoots and roots from all germinated seeds in each germination box were excised and collected separately for the determination of fresh weight. The collected shoots and roots were heated at 65 °C for 48 h, after which dry weights were measured. The relative rates of increase in shoot length, root length, and dry weight were calculated as follows:
where,
Xn represents the average length or weight in each treatment, and
X0 represents the average length or weight in the control treatment.
2.4. Determination of endogenous hormone and free amino acid contents
The contents of amino acids and endogenous hormones in maize shoot tips were analyzed using liquid chromatography-mass spectrometry following previously described methods.25–28 Briefly, fresh tissues of maize shoot-tips (2 cm) were ground to a fine powder in liquid nitrogen. To extract amino acids, 0.5 g of the fine powder was subjected to extraction at 4 °C for 15 h with 1 mL 50% ethanol (containing 0.1 mol L−1 HCl). After centrifugation at 12
000 × g and 4 °C for 10 min, the resulting supernatant was collected and diluted 20-fold with methanol for measurement. To obtain endogenous hormones, 0.5 g of fine powder was subjected to extraction at 4 °C with 2 mL of extraction solution (isopropanol
:
H2O
:
HCl = 2
:
1
:
0.002). After agitation for 30 min at 4 °C, dichloromethane (4 mL) was added, followed by centrifugation at 12
000 × g and 4 °C for 10 min. After centrifugation, the lower phase was dried under a stream of nitrogen at room temperature and re-suspended in 1 mL methanol for measurement.28
Following filtration using a 0.22 μm filter, samples were injected into an Inertsil OSD-4 C18 chromatographic column (150 mm × 3 mm, 3.5 μm) and analyzed using a binary solvent system. This system comprised mobile phase A (0.5% methanoic acid and 0.1% methanoic acid for amino acid and endogenous hormone detection, respectively) and mobile phase B (methanol), with a flow rate of 0.3 mL min−1. Contents were calculated with reference to standard curves.
2.5. Statistical analysis
The data presented in tables and figures were analyzed using a one-way analysis of variance with Duncan's multiple comparison test. A p-value <0.05 was considered statistically significant.
3 Results and discussion
3.1. Synthesis and characterization of [Chl][AA] ILs
[Chl][AA] ILs were synthesized via neutralization reactions conducted by mixing aqueous solutions of amino acids and choline hydroxide at an appropriate molar ratio, followed by the vacuum evaporation of water. In all cases, we obtained yields exceeding 95%. The structures and purity of the [Chl][AA] ILs were confirmed via 1H NMR analysis (ESI Table S1†), which corroborated with those reported in previous studies.13,29
3.2. Effect of [OPy][Br] on germination indices
The effects of the application of different concentrations of the conventionally used [OPy][Br] IL on germination rate, potential, and index of maize seeds are presented in Table 1. The application of [OPy][Br] at low concentrations did not significantly affect the germination rate. However, concentrations exceeding 50 mg L−1 led to concentration-dependent significant decreases in the indicators of maize seed germination, consistent with the findings of previous studies. For example, wheat germination has been demonstrated to be inhibited by 4.4 mmol L−1 1-butyl-3-methylimidazolium tetrafluoroborate, whereas minimal effect was observed at a concentration of 0.4 mmol L−1.30 Similarly, the inhibition of the seed germination of six tree species was more pronounced when higher concentrations of two ILs were used.31
Table 1 Effect of N-octyl pyridinium bromide on maize seed germinationa
Concentration (mg L−1) |
Germination rate (%) |
Germination potential (%) |
Germination index |
Values represent the means ± standard error (n = 3). Different letters in the same column indicate significant differences among different treatments at the 0.05 level of significance. |
0 |
97.22 ± 1.96a |
94.44 ± 1.96a |
18.99 ± 0.48a |
1 |
98.61 ± 1.96a |
95.83 ± 3.40a |
19.38 ± 0.83a |
5 |
97.22 ± 1.96a |
94.44 ± 1.96a |
18.55 ± 0.83a |
10 |
95.83 ± 3.40a |
93.06 ± 3.93a |
18.24 ± 0.23a |
50 |
95.83 ± 3.40a |
88.89 ± 3.93a |
18.01 ± 0.13a |
100 |
83.33 ± 5.89b |
70.83 ± 3.40b |
15.46 ± 0.19b |
200 |
63.89 ± 5.20c |
37.50 ± 3.40c |
11.62 ± 0.87c |
Owing to the high germination vigor of the examined seeds, the effect of [OPy][Br] on seed germination rate was relatively less pronounced than that on growth. The morphological characteristics of the seedlings emerging from seeds treated with different concentrations of [OPy][Br] are shown in Fig. 1. Notably, even at a low concentration of 5 mg L−1, [OPy][Br] markedly inhibited the shoot and root growth of maize; this inhibitory effect increased with higher concentrations. We attributed this significant growth inhibition to the action of lipophilic octyl substituents. These substituents can interact with the phospholipid bilayers of biomembranes and the hydrophobic domains of membrane proteins, thereby disrupting membrane physiological functions.32 At a [OPy][Br] concentration exceeding 10 mg L−1, the main root length was less than 5 cm, and both the length and weight of the shoots and roots decreased proportionally with increasing concentration. By fitting a logistic regression model, we calculated the effective concentration 50 for shoot and root lengths at 5 days as 30.51 and 5.06 mg L−1, respectively, indicating that maize roots were notably more sensitive to [OPy][Br] treatment than maize shoots. These findings are consistent with those of previous studies on the toxic effects of imidazolium-based or pyridinium-based compounds on different organisms.6,30,33
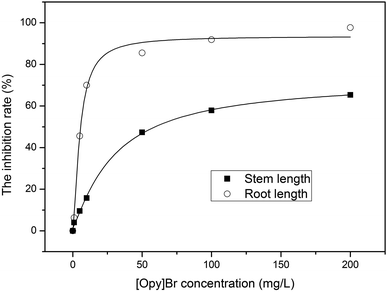 |
| Fig. 1 Effects of different concentrations of N-octyl pyridinium bromide on maize seed growth. | |
3.3. Effect of [Chl][AA] ILs on maize seed germination indices
Compared to the conventionally used [OPy][Br] IL, [Chl][AA] ILs exhibited substantially lower toxicity, which promoted the early growth of seedlings over a wide concentration range. The effects of different concentrations of the seven examined [Chl][AA] ILs on the germination rate, potential, and index of maize seeds are shown in Fig. 2.
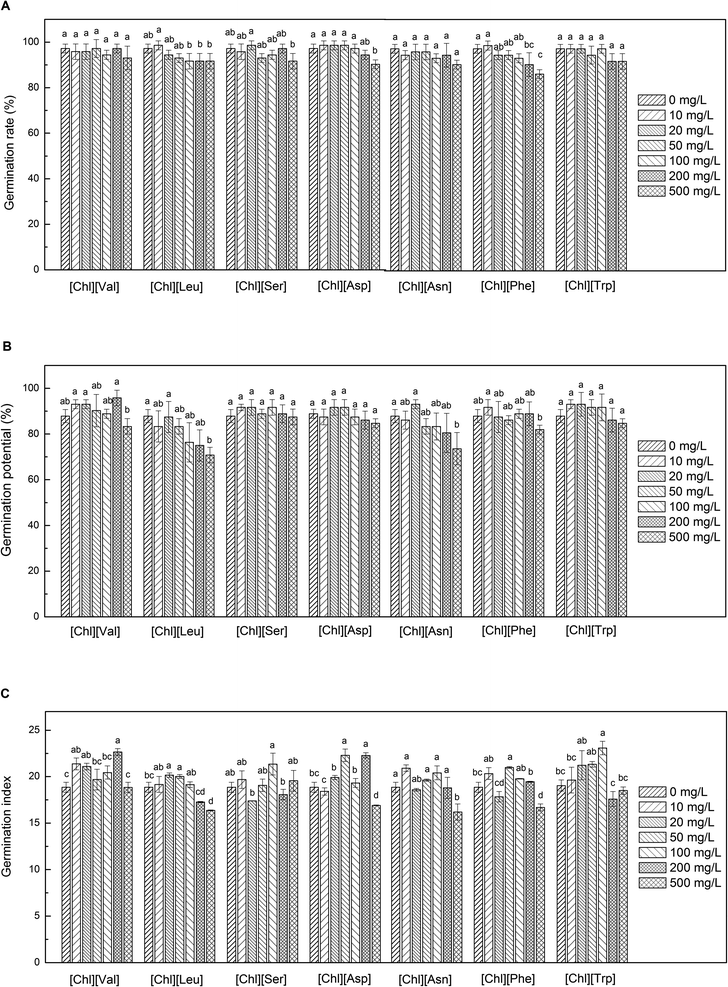 |
| Fig. 2 Effects of [Chl][AA] ILs on indicators of maize seed germination at applied concentrations (10, 20, 50, 100, 200, and 500 mg L−1). Control (CK): deionized water (0 mg L−1). (A) Germination rate; (B) germination potential; (C) germination index. Bars represent the means ± standard deviation (SD) of four replicates for each exposure concentration. The letters represent significant differences between varying concentrations of the same IL. | |
All the applied [CHL][AA] ILs had minimal to no impact on the maize germination rate. Even at a concentration of 500 mg L−1, the germination rate was higher than 87%. In contrast, differences were observed in the effects of different [Chl][AA] IL treatments on the germination potential of maize seeds. At concentrations up to 200 mg L−1, choline valine ([Chl][Val]), choline serine ([Chl][Ser]), and [Chl][Trp] promoted the germination potential that reached a maximum value of 95.8% with 200 mg L−1 [Chl][Val]. Differences were also observed in the effects of different [Chl][AA] IL treatments on the germination index of maize seeds. Compared to the control group (18.85), the seven examined [Chl][AA] ILs exhibited a promoting effect on germination indices at low concentrations, with [Chl][Trp] at a concentration of 100 mg L−1 showing the strongest effect (23.08). Based on the data obtained for these three germination indicators, it is evident that the seven [Chl][AA] ILs investigated in this study can be beneficially applied at low concentrations (<200 mg L−1) to enhance maize seed germination, thereby positioning them as environmentally friendly solvents. These findings are consistent with those of previous results that indicate that choline and its analogs exhibit low toxicity toward microbes and fish cell lines.14,34 Moreover, appropriate concentrations of exogenous choline and amino acids can promote seed germination and plant growth. Such as ammonium salts or binary mixtures derived from amino acids, glycine betaine, choline and indole-3-butyric acid can significantly enhance mustard seed germination and seedling growth.35 Exogenous amino acids increased the germination rate of onion seeds and enhanced the synthesis of photosynthetic pigments in seedlings.
3.4. Effect of [Chl][AA] ILs on the shoot and root growth of maize seedlings
The effects of the seven examined [Chl][AA] ILs on the lengths of the shoots and roots of maize seedlings are shown in Fig. 3. [Chl][AA] ILs promoted shoot and root growth to varying degrees at low concentrations (<200 mg L−1), with the optimal concentration of choline leucine ([Chl][Leu]), [Chl][Ser], choline aspartic acid ([Chl][Asp]), choline phenylalanine ([Chl][Phe]), and [Chl][Trp] being 100 mg L−1. At this concentration, the rates of increase in shoot length were 7.0%, 23.9%, 21.5%, 22.5%, and 24.5%, respectively, whereas that of root lengths were 17.8%, 28.1%, 17.1%, 12.8%, and 16.7%, respectively.
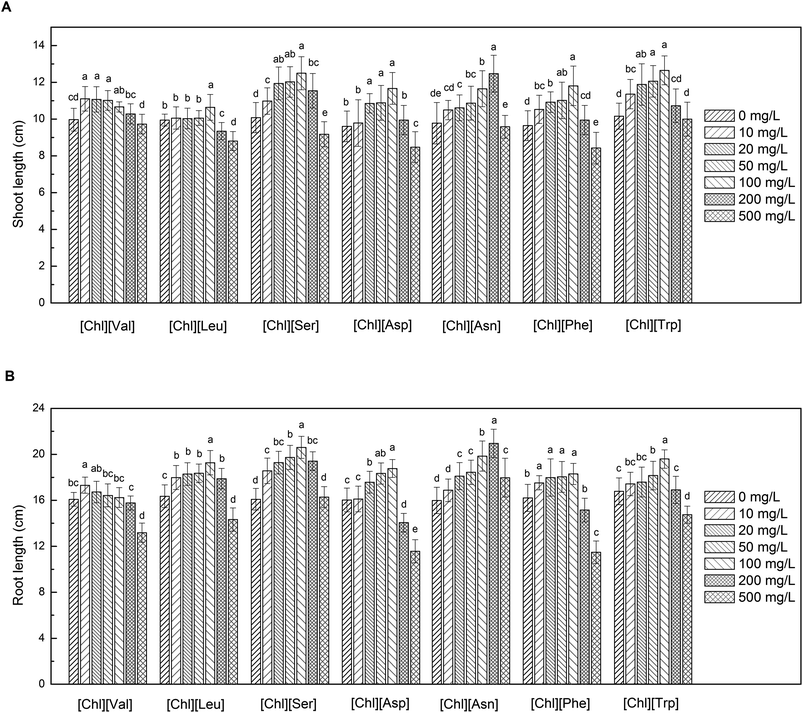 |
| Fig. 3 Effects of the seven examined [Chl][AA] ILs on the lengths of maize shoots and roots at applied concentrations (10, 20, 50, 100, 200, and 500 mg L−1). (A) Increasing rate of shoot length; (B) increasing rate of root length. Bars represent the means ± SD of six replicates for each exposure concentration. The letters represent significant differences between varying concentrations of the same IL. | |
Although the relatively lower toxicity of choline-based ILs has been reported in other studies,14,36,37 there is currently a dearth of experimental data regarding the phytotoxicity of [Chl][AA] ILs, and their role in promoting plant growth is rarely discussed. These potential growth-promoting effects could be attributable to the capacity of [Chl][AA] ILs to effectively eliminate accumulated toxic substances in seeds during storage and reduce the levels of membrane lipid peroxidation during seed germination, consistent with the role of vitamin E.38 The process of seed germination and seedling growth is accompanied by the transformation of complex materials, such as starch, proteins, and lipids.39 Thus, applying low concentrations of [Chl][AA] to maize seeds may facilitate the degradation of these large molecular substances into micro-molecules to fuel seedling growth and induce the rapid and efficient mobilization of nutrients to the shoots and roots, thereby stimulating plant growth.40
3.5. Effects of [Chl][AA] ILs on the endogenous hormone contents of maize shoot tips
[Chl][AA] ILs, at a concentration of 100 mg L−1, induced visible changes in the contents of endogenous hormones in the shoot tips of maize (Table 2). Compared to the control, the contents of zeatin (ZT) and zeatin riboside (ZTR) exhibited varying degrees of enhancement following treatment. The most substantial increases in ZT and ZTR contents were observed with [Chl][Phe] and [Chl][Leu] treatment, achieving 4.69- and 1.94-fold higher levels than those detected in the control group, respectively. Furthermore, in all seven treatments, an increase of more than 32% was observed in the gibberellic acid (GA3) content of maize shoot tips compared to that in the control group. Consequently, the effects of [Chl][AA] ILs in promoting maize seed germination could be attributed, at least in part, to the increased levels of growth-promoting plant hormones such as ZT, ZTR, and GA3.41 In contrast, a decreasing trend in the content of jasmonic acid was observed following [Chl][AA] IL treatment.
Table 2 Effects of the seven choline amino acid ionic liquids on the concentrations of zeatin, zeatin riboside, gibberellic acid, jasmonic acid, and salicylic acid in maize shoot tipsa
Treatment |
ZT content (ng g−1 FW) |
ZTR content (ng g−1 FW) |
GA3 content (ng g−1 FW) |
JA content (ng g−1 FW) |
SA content (ng g−1 FW) |
Values represent the means ± standard error (n = 3). Different letters in the same column indicate significant differences among different treatments at the 0.05 level of significance. [Chl][Val]: choline valine, [Chl][Leu]: choline leucine, [Chl][Ser]: choline serine, [Chl][Asp]: choline aspartic acid, [Chl][Asn]: choline asparagine, [Chl][Phe]: choline phenylalanine, [Chl][Trp]: choline tryptophan, ZT: zeatin, ZTR: zeatin riboside, GA3: gibberellic acid, JA: jasmonic acid, SA: salicylic acid. |
CK |
0.74 ± 0.08 c |
0.49 ± 0.08 e |
1.76 ± 0.03 b |
44.61 ± 2.10 a |
9.17 ± 0.13 a |
[Chl] Val |
2.13 ± 0.32 b |
0.52 ± 0.03 e |
2.80 ± 0.30 a |
18.12 ± 0.81 d,e |
9.55 ± 0.22 a |
[Chl] Trp |
1.29 ± 0.16 c |
0.55 ± 0.03 d,e |
2.34 ± 0.18 a |
19.57 ± 1.60 c,d |
9.33 ± 0.19 a |
[Chl] Phe |
3.47 ± 0.07 a |
0.83 ± 0.04 ab |
2.51 ± 0.21 a |
23.74 ± 0.50 b |
9.55 ± 0.30 a |
[Chl] Ser |
2.53 ± 0.40 b |
0.69 ± 0.08 b,c,d |
2.37 ± 0.03 a |
21.37 ± 1.25 c |
8.11 ± 0.29 b |
[Chl] Leu |
2.15 ± 0.01 b |
0.95 ± 0.05 a |
2.41 ± 0.33 a |
16.86 ± 0.75 e |
7.21 ± 0.56 c |
[Chl] Asp |
2.14 ± 0.29 b |
0.74 ± 0.12 b,c |
2.57 ± 0.19 a |
17.10 ± 0.53 e |
9.17 ± 0.73 a |
[Chl] Asn |
2.67 ± 0.08 b |
0.63 ± 0.02 c,d,e |
2.51 ± 0.10 a |
12.44 ± 0.48 f |
7.30 ± 0.27 b,c |
Choline compounds may affect plant growth by regulating plant hormone metabolism. For example, 2-chloroethyltrimethylammonium chloride and 2-ethyltrimethylammonium chloride increased plant chlorophyll content, which was related to its increase in leaf cytokinin levels.42 Genetic engineering of the biosynthesis of glycinebetaine could enlarge the fruit size and enhance the productivity of tomato, which was related to the contents of phytohormones, such as auxin, brassinolide, gibberellin, and cytokini.43 Moreover, JA-GA network influences the intensity of defense responses.44 In this study, exogenous choline amino acids significantly increased cytokinin and gibberellin content, while reducing jasmonic acid content, which is consistent with previous researches.
3.6. Effects of [Chl][AA] ILs on the endogenous free amino acid contents of maize shoot tips
Amino acid metabolism provides the basis for plant nitrogen metabolism and protein synthesis. [Chl][AA] ILs, at a concentration of 100 mg L−1, induced notable increases in the contents of glutamic acid (Glu), glycine (Gly), and arginine in maize shoot tips (Table 3). Glu and Gly are major metabolic precursors for the synthesis of other amino acids and serve as active sources for nitrogen assimilation and transport.45 However, given its high nitrogen-to-carbon ratio, Arg plays an important role in plant nitrogen storage.46 Collectively, our findings indicate that exogenous [Chl][AA] IL treatment may contribute to enhancing the nitrogen metabolic status of maize shoot tips. However, reduced levels of certain amino acids, including tryptophan and proline, were observed following different exogenous [Chl][AA] IL treatments.
Table 3 Effects of seven choline amino acid ionic liquids (100 mg L−1) on the contents of free amino acids in maize shoot tipsa
Treatment |
Free amino acid content in maize shoot-tip (μg g−1 FW) |
Gly |
Arg |
Glu |
Phe |
Ser |
Thr |
Tyr |
Val |
Ala |
Pro |
Values represent the means ± standard error (n = 3). Different letters in the same column indicate significant differences among different treatments at the 0.05 level of significance. Gly: glycine, Arg: arginine, Glu: glutamic acid, Phe: phenylalanine, Ser: serine, Thr: threonine, Tyr: threonine, Val: valine, Ala: alanine, Pro: proline. |
CK |
34.8 ± 0.4g |
3.2 ± 0.3d |
30.1 ± 0.7c |
30.4 ± 0.1a |
41.0 ± 0.6c |
21.1 ± 0.1a |
14.7 ± 0.3a |
16.2 ± 0.0b |
49.6 ± 0.6b |
16.6 ± 0.2 a |
[Chl] Val |
41.1 ± 0.6d |
4.9 ± 0.7bc |
32.5 ± 0.7bc |
26.5 ± 0.6d |
40.7 ± 0.4 cd |
19.9 ± 0.2b |
12.9 ± 0.1c |
15.4 ± 0.2c |
44.6 ± 0.9c |
12.9 ± 0.23c |
[Chl] Trp |
42.4 ± 0.5c |
6.4 ± 0.1a |
32.9 ± 0.6bc |
27.7 ± 0.1c |
41.7 ± 0.3c |
20.0 ± 0.2b |
13.4 ± 0.2b |
15.5 ± 0.1c |
45.4 ± 0.4c |
13.5 ± 0.1b |
[Chl] Phe |
39.8 ± 0.5e |
4.1 ± 0.7 cd |
32.8 ± 1.0bc |
26.1 ± 0.3d |
39.9 ± 0.3d |
19.4 ± 0.2c |
12.2 ± 0.2d |
14.5 ± 0.1e |
42.2 ± 0.4d |
11.5 ± 0.1e |
[Chl] Ser |
38.1 ± 0.8f |
5.3 ± 0.5b |
34.4 ± 0.9b |
25.0 ± 0.5e |
41.5 ± 0.5c |
19.1 ± 0.2c |
12.3 ± 0.2d |
14.6 ± 0.2e |
41.9 ± 0.9d |
13.0 ± 0.2c |
[Chl] Leu |
42.6 ± 0.2c |
3.5 ± 0.2d |
33.9 ± 0.3b |
25.7 ± 0.8de |
43.8 ± 0.5b |
19.0 ± 0.2c |
11.9 ± 0.1d |
15.0 ± 0.2d |
45.9 ± 0.6c |
11.8 ± 0.1e |
[Chl] Asp |
45.5 ± 0.3b |
6.4 ± 0.1a |
38.0 ± 0.4a |
29.9 ± 0.6 ab |
43.9 ± 0.35b |
19.9 ± 0.2b |
13.5 ± 0.3b |
16.0 ± 0.1b |
49.5 ± 0.4b |
12.3 ± 0.1d |
[Chl] Asn |
48.9 ± 0.5a |
6.8 ± 0.20a |
33.5 ± 4.0bc |
28.9 ± 0.3b |
45.9 ± 0.3a |
21.0 ± 0.0a |
14.3 ± 0.2a |
16.5 ± 0.1a |
52.2 ± 1.1a |
13.1 ± 0.0c |
3.7. Role of choline substances in plant growth regulation
Choline, in contrast to imidazole or pyridine, is a quaternary amine that occurs widely in living organisms.47 As a metabolic intermediate, choline plays an important role in the development of cell membrane structures and the maintenance of membrane stability. For example, phosphatidylcholine is among the most abundant phospholipid species found in cell membranes.48 Its biosynthesis from choline, as a precursor, is mediated via the cytidine 5′-diphosphocholine-choline pathway.17 Phosphatidylcholine is a key constituent in the synthesis of plant glycerides, consequently making a significant contribution to maintaining the normal structure and function of cell membranes. Phosphatidylcholine also serves as a major precursor for multiple lipid signaling molecules, including phosphatidic acid and lysophosphatidylcholine.49 Regarding chemical regulation in plants, certain choline analogs, including choline chloride, chlorocholine chloride, and betaine, serve as plant growth regulators, which have demonstrated efficacy in enhancing crop stress tolerance.18 From this perspective, exogenous [Chl][AA] ILs may play similar physiological roles as these plant growth regulators. Given the close association between germination quality and final crop yield, it is plausible to anticipate that the application of [Chl][AA] ILs at appropriate concentrations might exert stimulatory effects on maize germination and early growth, thereby contributing to higher yields.
5 Conclusions
Exposure to appropriate concentrations of selected [Chl][AA] ILs promoted the germination of maize seeds to varying degrees. Moreover, the application of 100 mg L−1 [Chl][AA] ILs led to an increase in the contents of certain growth-promoting hormones and amino acids. These stimulatory properties may provide new insights for broadening the application potential of eco-friendly ILs in the field of agriculture and in the recycling of waste ILs. Additionally, they may also contribute to reducing the potential biological toxicity of traditionally employed ILs.
Abbreviations
[Chl][AA] ILs | Choline amino acid ionic liquids |
[OPy][Br] | N-Octyl pyridinium bromide |
[Chl][Trp] | Choline tryptophan |
[Chl][Val] | Choline valine |
[Chl][Leu] | Choline leucine |
[Chl][Ser] | Choline serine |
[Chl][Asp] | Choline aspartic acid |
[Chl][Asn] | Choline asparagine |
[Chl][Phe] | Choline phenylalanine |
CK | Control |
SD | Standard deviation |
ZT | Zeatin |
ZTR | Zeatin riboside |
GA3 | Gibberellic acid |
JA | Jasmonic acid |
SA: | Salicylic acid |
Glu | Glutamic acid |
Gly | Glycine |
Author contributions
Xiaohong Chen: investigation; methodology; validation; writing – original draft. Rui Hao: investigation; methodology; validation. Wenquan Chen: investigation; methodology. Huimin Jia: investigation; methodology. Shufang Qin: investigation; methodology. Qi Wang: investigation; methodology. Dingxiang Zhang: investigation; methodology. Zhaoxue Han: Project administration. Yajun Li: Project administration; methodology; validation; writing – review & editing.
Conflicts of interest
There are no conflicts of interest to declare.
Acknowledgements
This study was supported by the National Natural Science Foundation of China (Grant No. 32272018 and 32272047). National key research and development program (2021YFD1600602-05), Shaanxi Natural Scientific Basic Research Program project (2022JQ-157 and 2021JM-101).
References
- S. Bai, P. Da, C. Li, Z. Wang, Z. Yuan, F. Fu, M. Kawecki, X. Liu, N. Sakai, J. T.-W. Wang, S. Huettner, S. Buecheler, M. Fahlman, F. Gao and H. J. Snaith, Nature, 2019, 571, 245 CrossRef CAS PubMed
. - M. Musial, A. B. Olejniczak, M. Denel-Bobrowska, E. Zorebski and M. Dzida, ACS Sustain. Chem. Eng., 2021, 9, 16459–16465 CrossRef CAS
. - J. L. Shamshina, S. P. Kelley, G. Gurau and R. D. Rogers, Nature, 2015, 528, 188–189 CrossRef CAS PubMed
. - M. Wang, P. Zhang, M. Shamsi, J. L. Thelen, W. Qian, T. Vi Khanh, J. Ma, J. Hu and M. D. Dickey, Nat. Mater., 2022, 21, 359–+ CrossRef CAS PubMed
. - Z. W. Yuan, H. Liu, W. F. Yong, Q. H. She and J. Esteban, Green Chem., 2022, 24, 1895–1929 RSC
. - S. P. F. Costa, A. M. O. Azevedo, P. C. A. G. Pinto and M. L. M. F. S. Saraiva, Chemsuschem, 2017, 10, 2321–2347 CrossRef CAS PubMed
. - H. Ohno, in Advances in Biochemical Engineering/Biotechnology, Springer, 2018, vol. 168, ch. 199–214 Search PubMed
. - P. Y. Quintas, E. F. Fiorentini, M. Llaver, R. E. Gonz and R. G. Wuilloud, Trac. Trends Anal. Chem., 2022, 157 Search PubMed
. - N. S. Mohamed Hatta, M. K. Aroua, F. Hussin and L. T. Gew, Energies, 2022, 15 Search PubMed
. - Z. Y. Zhou, F. H. Lei, P. F. Li and J. X. Jiang, Biotechnol. Bioeng., 2018, 115, 2683–2702 CrossRef CAS PubMed
. - Y. Cao, A. T. Nakhjiri and M. Ghadiri, Eur. Phys. J. Plus, 2022, 137 Search PubMed
. - S. Q. Hu, T. Jiang, Z. F. Zhang, A. L. Zhu, B. X. Han, J. L. Song, Y. Xie and W. J. Li, Tetrahedron Lett., 2007, 48, 5613–5617 CrossRef CAS
. - Q. P. Liu, X. D. Hou, N. Li and M. H. Zong, Green Chem., 2012, 14, 304–307 RSC
. - X. D. Hou, Q. P. Liu, T. J. Smith, N. Li and M. H. Zong, PLoS One, 2013, 8, e59145 CrossRef CAS PubMed
. - K. Radosevic, J. Zeleznjak, M. C. Bubalo, I. R. Redovnikovic, I. Slivac and V. G. Srcek, Ecotox. Environ. Safe., 2016, 131, 30–36 CrossRef CAS PubMed
. - F.-S. Che, C. Cho, S.-B. Hyeon, A. Isogai and A. Suzuki, Plant Cell Physiol., 1990, 31, 45–50 CAS
. - Y. Lin, K. Kanehara and Y. Nakamura, New Phytol., 2019, 223, 1904–1917 CrossRef CAS PubMed
. - I. Hussain, M. H. Saleem, S. Mumtaz, R. Rasheed, M. A. Ashraf, F. Maqsood, M. Rehman, H. Yasmin, S. Ahmed and M. Ishtiaq, J. Plant Growth Regul., 2021, 1–21 Search PubMed
. - W. Rademacher, Plant Physiol., 1992, 100, 625–629 CrossRef CAS PubMed
. - M. Anwar-ul-Haq, I. Iftikhar, J. Akhtar and M. Maqsood, J. Plant Nutr. Soil Sci., 2023, 23(3), 3682–3694 CrossRef CAS
. - H. M. Wang, C. Y. Ren, L. Cao, Q. Zhao, X. J. Jin, M. X. Wang, M. C. Zhang, G. B. Yu and Y. X. Zhang, Front. Plant Sci., 2022, 13, 865758 CrossRef PubMed
. - M. Abdelkader, L. Voronina, M. Puchkov, N. Shcherbakova, E. Pakina, M. Zargar and M. Lyashko, Horticulturae, 2023, 9(1), 80 CrossRef
. - X. F. Xue, S. Y. Du, F. C. Jiao, M. H. Xi, A. G. Wang, H. C. Xu, Q. Q. Jiao, X. Zhang, H. Jiang, J. T. Chen and M. Wang, Crop J., 2021, 9, 718–724 CrossRef
. - S. Y. Zuo, J. Li, W. R. Gu and S. Wei, Agriculture, 2022, 12(4), 548 CrossRef
. - Q. Chen, Y. Wang, Z. Zhang, X. Liu, C. Li and F. Ma, Front. Plant Sci., 2022, 12, 772086 CrossRef PubMed
. - X. Jin, T. Liu, J. Xu, Z. Gao and X. Hu, BMC Plant Biol., 2019, 19, 48 CrossRef PubMed
. - X. Pan, R. Welti and X. Wang, Nat. Protoc., 2010, 5, 986–992 CrossRef CAS PubMed
. - X. Pan, R. Welti and X. Wang, Phytochemistry, 2008, 69, 1773–1781 CrossRef CAS PubMed
. - P. Moriel, E. J. Garcia-Suarez, M. Martinez, A. B. Garcia, M. A. Montes-Moran, V. Calvino-Casilda and M. A. Banares, Tetrahedron Lett., 2010, 51, 4877–4881 CrossRef CAS
. - L. S. Wang, L. Wang, L. Wang, G. Wang, Z. H. Li and J. J. Wang, Environ. Toxicol., 2009, 24, 296–303 CrossRef CAS PubMed
. - J. Salgado, J. J. Parajo, T. Teijeira, O. Cruz, J. Proupin, M. Villanueva, J. A. Rodriguez-Anon, P. V. Verdes and O. Reyes, Chemosphere, 2017, 185, 665–672 CrossRef CAS PubMed
. - H. J. Liu, S. X. Zhang, X. N. Hu and C. D. Chen, Environ. Pollut., 2013, 181, 242–249 CrossRef CAS PubMed
. - S. A. Perez, M. G. Montalban, G. Carissimi, P. Licence and G. Villora, J. Hazard. Mater., 2020, 385 Search PubMed
. - I. J. Ferreira, A. Paiva, M. Diniz and A. R. Duarte, Environ. Sci. Pollut. Res., 2023, 30, 40218–40229 CrossRef CAS PubMed
. - D. K. Kaczmarek, A. Parus, M. Lozynski and J. Pernak, RSC Adv., 2020, 10, 43058–43065 RSC
. - S. Zhang, L. Ma, P. Wen, X. Ye, R. Dong, W. Sun, M. Fan, D. Yang, F. Zhou and W. Liu, Tribol. Int., 2018, 121, 435–441 CrossRef CAS
. - A. R. Bhat, M. Ahmed, F. A. Wani, Y. Kumar and R. Patel, Colloids Surf., A, 2022, 650, 129388 CrossRef CAS
. - S. E. Sattler, L. U. Gilliland, M. Magallanes-Lundback, M. Pollard and D. Dellapenna, Plant Cell, 2004, 16, 1419–1432 CrossRef CAS PubMed
. - I. A. Graham, Annu. Rev. Plant Biol., 2008, 59, 115–142 CrossRef CAS PubMed
. - S. L. Luis, G. R. Marina, D. P. David, G. C. Fernando, C. A. Viridiana, Z. V. Viridiana, L. L. Viridiana, M. S. Rafael, B. L. Irma and S. N. Sobeida, J. Exp. Bot., 2012, 63, 4513–4526 CrossRef
. - J. Xia, X. Hao, T. Wang, H. Li, X. Shi, Y. Liu and H. Luo, J. Plant Growth Regul., 2022, 42(1), 319–334 CrossRef
. - V. Kreslavski, E. Kobzar, E. Ivanova and E. Kuznetsov, Plant Growth Regul., 2005, 47, 9–15 CrossRef CAS
. - T. P. Zhang, J. A. Liang, M. W. Wang, D. X. Li, Y. Liu, T. H. H. Chen and X. H. Yang, Plant Sci., 2019, 280, 355–366 CrossRef CAS PubMed
. - A. Wiszniewska, A. Kozminska, E. Hanus-Fajerska, K. Dziurka and M. Dziurka, Plant Soil, 2021, 463, 55–76 CrossRef CAS
. - X. S. Liu, L. M. Wang, Z. Z. Li and D. F. Huang, J. Plant Nutr., 2014, 37, 765–776 CrossRef CAS
. - M.-T. Llebrés, M.-B. Pascual, S. Debille, J.-F. Trontin, L. Harvengt, C. Avila and F. M. Cánovas, Tree Physiol., 2017, 38, 471–484 CrossRef PubMed
. - C. R. Warren, New Phytol., 2013, 198, 476–485 CrossRef CAS PubMed
. - M. Tannert, G. U. Balcke, A. Tissier and M. Köck, Plant J., 2021, 107, 1072–1083 CrossRef CAS PubMed
. - W. Chen, M. C. Taylor, R. A. Barrow, M. Croyal and J. Masle, Plant Physiol., 2019, 179, 124–142 CrossRef CAS PubMed
.
|
This journal is © The Royal Society of Chemistry 2024 |
Click here to see how this site uses Cookies. View our privacy policy here.