DOI:
10.1039/D3RA07536B
(Paper)
RSC Adv., 2024,
14, 2285-2292
Synthesis and self-assembly of dendritic–linear block copolymers containing poly(mandelic acid) with discrete molecular weights and stereochemical structures†
Received
4th November 2023
, Accepted 7th January 2024
First published on 10th January 2024
Abstract
In this work, we present the synthesis of uniform PMAs, where the number of repeat units and their stereochemical arrangement are precisely defined. Utilizing an iterative convergent approach with orthogonally protected dimandelic acid building blocks, we achieved high molecular weight PMAs with the desired number of repeat units, extending up to 144 mandelic acids. Additionally, stereochemically defined poly(L-mandelic acid)s with up to 32 repeat units were successfully synthesized. These uniform PMAs were subsequently coupled with uniform branched poly(ethylene glycol) blocks to create uniform dendritic–linear block copolymers. The self-assembly of these block copolymers in solution was systematically investigated. In solution self-assembly, the synthesized block copolymers showed multiple phases from cylinder to inverse cubic as the molecular weight of PMA increased. In the case of solvent diffusion-evaporation-mediated self-assembly, the block copolymers underwent a phase transition as the rate of water addition decreased.
Introduction
Poly(mandelic acid) (PMA) represents a biodegradable polymer that shares structural and property similarities with polystyrene (PS) but offers the additional benefit of environmental sustainability.1–3 Traditionally, PMA synthesis via bulk ring-opening polymerization of mandelide lacked control over its molecular weight and distribution. High-temperature ROP led to stereocenter epimerization and racemization.4,5 Fortunately, catalytic ROP of cyclic O-carboxyanhydrides (OCA) and 5-phenyl-1,3-dioxolane-4-one (Ph-DOX) has resolved these challenges, yielding well-defined PMAs in terms of molecular weight, stereoregularity, and chain topology.5–10 Thermal analyses of linear and cyclic PMAs have indicated high glass transition temperatures (Tg = 109 °C) and crystallinity (Tm = 180 °C) without compromising degradability.11 These results strongly support PMA as a biodegradable alternative to traditional plastics. However, recent syntheses of highly isotactic PMAs (Pm > 0.99) have resulted in varying melting temperatures (Tm) and increased molecular weight (Mn > 10k), making it challenging to maintain low polydispersity (PDI > 1.2).8–10
In this study, we present the synthesis of PMAs with precisely defined molecular weight and stereochemical structures. This was achieved through an iterative exponential growth process utilizing orthogonally protected dimandelic acid as a building block. Our approach yielded uniform PMAs with up to 144 mandelic repeat units, preventing stereocenter epimerization. We also conducted thermal property and crystallization analyses of isotactic L-PMA. As an application example of PMAs as PS alternatives, we incorporated uniform PMAs with varying molecular weight poly(ethylene glycol) (PEG) segments into the PMA backbone and explored solution self-assembly (Fig. 1).
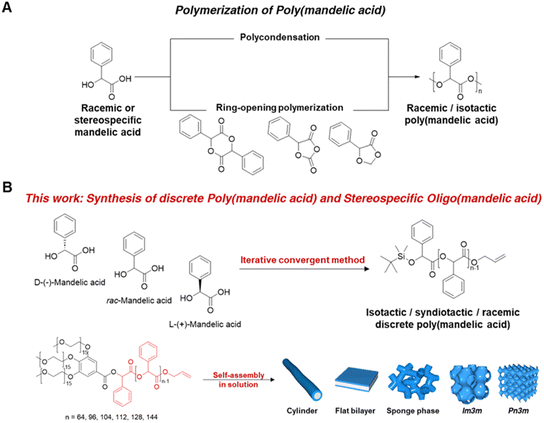 |
| Fig. 1 (A) Synthesis of racemic or isotactic poly(mandelic acid) via polycondensation and ring-opening polymerization (ref. 5–10). (B) Synthesis of racemic discrete PMA and isotactic/syndiotactic discrete oligo(mandelic acid) via iterative convergent method and solution self-assembly of dendritic–linear discrete 3mPEG15-b-uPMA. | |
Results & discussion
Synthesis of discrete poly(mandelic acid)s
We synthesized poly(mandelic acid)s with uniform molecular weights (uPMAs) through the iterative convergent pathway, employing dimandelic acid with t-butylsilyl and allyl protecting groups as a building block (MA2 in Scheme 1). The allyl ester group was selected as a protecting group owing to susceptibility of mandelic acid to decomposition via hydrogenolysis. Subsequently, the allyl protecting group was removed through an allyl exchange reaction with piperidine in the presence of Pd(PPh3)4. Following orthogonal deprotection of MA2, the carbodiimide-mediated coupling of HO-MA2-allyl and TBDMS-MA2-COOH produced the tetramer of MA after purification (MA4 in Scheme 1). This iterative convergence and purification process led to the generation of oligomeric and polymeric MAs with discrete molecular weights (Scheme 1, upper). High molecular-weight uPMAs were purified using preparative size-exclusion chromatography (prep-SEC), capitalizing on the molecular weight differences between deprotected precursors and coupled products (Fig. S1 and S2†). Additionally, we synthesized uPMA with the desired molecular weight through cross-convergence between two precursors of different molecular weights (Scheme 1, below). All uPMAs underwent comprehensive characterization through 1H and 13C NMR, gel permeation chromatography (GPC), and matrix-assisted laser desorption ionization time-of-flight (MALDI-TOF) mass spectrometry, confirming their monodispersity in molecular weight (Fig. S5, S6, S12, S14–S23† and 2). Molecular weight values of all uPMAs analyzed by MALDI-TOF and GPC are summarized in Table 1. Poly(mandelic acid)s exhibit thermal characteristics similar to polystyrene, including glass transition temperature (Tg).1–5 We determined the Tgs of PMAs through differential scanning calorimetry (DSC). The measured Tgs increased proportionally with the number of repeating units. Tgs of MAn (n > 48) reached a plateau at 360 K (Fig. 3A). A Flory–Fox plot of the Tg of MA8–MA128 against the 1/M values (Fig. 3B) corroborated that the Tg of an infinitely long MAn (Tg,∞ = 370.10 K) aligns with the literature values for amorphous PMAs.5
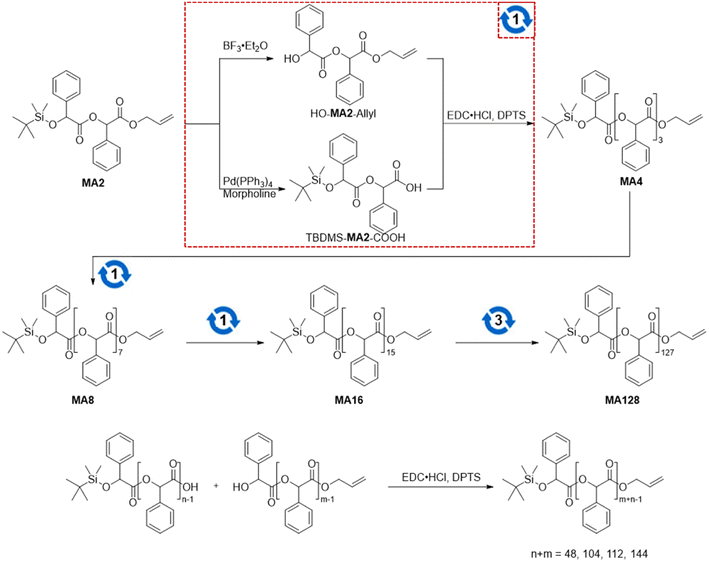 |
| Scheme 1 (upper) Iterative convergent synthesis of uPMA. The red dotted square indicates a convergence of linear precursors. The numbers inside circular arrows refer to the number of iterations of the convergence. (below) Hetero convergence of synthesizing uPMAs. | |
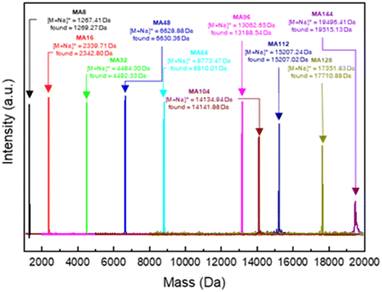 |
| Fig. 2 Combined MALDI-TOF mass spectra of uPMAs. | |
Table 1 Molecular weight analysis of uniform linear PMAs
Entry |
MALDI-TOF |
GPC |
Calculated ([M + Na]+) |
Found ([M + Na]+) |
Mna (kDa) |
Mwa (kDa) |
PDIa |
GPC was calibrated with a linear PS standard kit. |
MA16 |
2339.71 |
2342.80 |
2.10 |
2.14 |
1.02 |
MA32 |
4484.30 |
4487.45 |
3.95 |
4.07 |
1.03 |
MA48 |
6628.88 |
6630.36 |
6.06 |
6.30 |
1.04 |
MA64 |
8773.47 |
8810.01 |
7.64 |
8.17 |
1.07 |
MA96 |
13 062.65 |
13 188.54 |
11.70 |
12.17 |
1.04 |
MA104 |
14 134.94 |
14 141.88 |
12.91 |
13.56 |
1.05 |
MA112 |
15 207.24 |
15 207.02 |
13.74 |
14.43 |
1.05 |
MA128 |
17 351.83 |
17 710.88 |
15.35 |
16.12 |
1.05 |
MA144 |
19 496.41 |
19 515.13 |
15.84 |
17.27 |
1.09 |
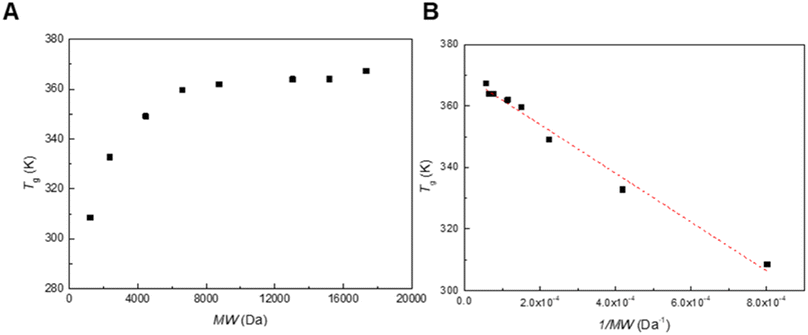 |
| Fig. 3 (A) Tg vs. MW plots of linear uPMAs. (B) The Flory–Fox plots of linear uPMAs. | |
Synthesis of isotactic and syndiotactic mandelic acid
Ring-opening polymerization of mandelide is prone to racemization of ⍺-hydrogen, resulting in stereoirregular PMAs.12,13 To overcome this issue, Buchard and coworkers reported that the ROP of cyclic O-carboxyanhydride (L-manOCA) could produce stereoregular PMAs.6 However, achieving absolute control of the stereochemistry of PMA at the monomer level through conventional ROPs remains challenging. Therefore, we synthesized isotactic and syndiotactic uPMA, with chains containing up to 32 L-mandelic acid (L-MA32).
Employing iterative convergent pathways using isotactic and syndiotactic MA2 as building blocks, we successfully synthesized discrete oligo(mandelic acid)s with defined stereochemical arrangements (Scheme 1). The stereochemical configuration of the monomer units was determined through 1H and 13C NMR spectroscopy (Fig. S7 and S8†). DSC data unveiled distinct properties for isotactic and syndiotactic oligo(mandelic acid)s. Notably, L-MA8 exhibited a melting temperature (Tm) at 57.9 °C, while DL-MA8 showed no Tm during the first heating and freezing scans (Fig. S35†). Based on these results, 3mPEG15-Me, L-MA32, and 3mPEG-b-LMA32 were synthesized (Scheme 1 and 2) and analyzed by DSC (Fig. 4A and B). L-MA32 showed Tg of 87.9 °C at first heating/freezing cycle,15 which is higher than that of amorphous MA32 (76.0 °C, Fig. 3A). Conversely, 3mPEG15-b-LMA32 showed a Tm of 95.4 °C at first heating cycle. Subsequently, crystallization-driven self-assembly (CDSA) of dendritic–linear block copolymers containing L-MA32 was performed.14–17 3mPEG15-b-LMA32 was dissolved in acetonitrile (1 mg mL−1) and heated at 90 °C, followed by a slow cooling and equilibrium at 25 °C without perturbation for 24 h. The resulting solution was diluted by acetonitrile and analyzed through TEM. The diameters of more than 100 crystals were measured by analyzing the electron microscopy images.18–20 TEM images showed a crystalline structure with the diameter of Ln = 34.8 nm, Lw = 37.5 nm, and Lw/Ln = 1.08 with various lengths (Fig. 4C and S36†).
 |
| Scheme 2 Synthesis of 3mPEG15-b-uPMA block copolymers. | |
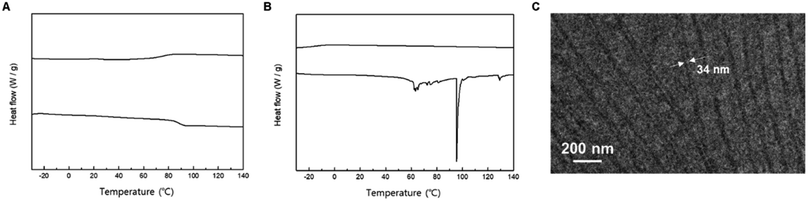 |
| Fig. 4 DSC (first heating and freezing scans, heat rate = 10 °C min−1) results of (A) L-MA32 and (B) 3mPEG-b-LMA32. (C) TEM image of 3mPEG15-b-LMA32 for crystallization-driven self-assembly in acetonitrile. | |
Synthesis of block copolymers and self-assembly
Solution self-assembly is a powerful method for exploiting the relationship between the chemical structure and self-assembly behavior of amphiphiles.21–26 In our previous studies, we investigated amphiphilic block copolymers comprising branched PEG and linear PS, where we observed inverse cubic phases with varying molecular weights.27–30 Based on the similarities in structures and properties between poly(mandelic acid) and polystyrene, we investigated the discrete molecular weight analogs of PEG-PS dendritic–linear BCPs using a branched PEG block and poly(mandelic acid)s without molecular weight distribution.
We began by synthesizing monomethoxy poly(ethylene glycol) with a uniform molecular weight distribution (mPEG), extending the chain length from 11 to 15 repeating units following the literature.31 By combining tosylated mPEG with 15 repeating units and methyl gallate, we successfully synthesized branched discrete poly(ethylene glycol) with 15 repeating units (3mPEG15). Characterization of the synthesized 3mPEG15 was conducted through 1H NMR, GPC, and MALDI-TOF (Fig. S9, S10, S13, and S24–S27†). 3mPEG15 served as the hydrophilic block for subsequent block copolymer synthesis. The carbodiimide-mediated coupling of 3mPEG15-COOH and HO-uPMA resulted in 3mPEG15-b-uPMA after purification (Scheme 2). All block copolymers underwent thorough characterization, including 1H and 13C NMR, GPC, and MALDI-TOF mass spectrometry, to ensure the absence of any remaining precursors (Fig. 5A, B and S28–S33†). Molecular weight values of all 3mPEG15-b-uPMAs analyzed by MALDI-TOF and GPC are summarized in Table 2.
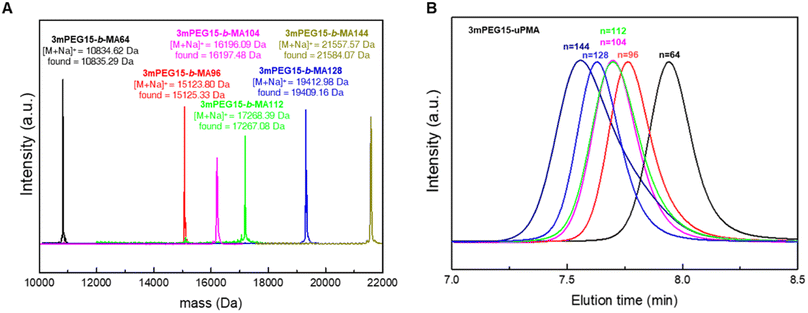 |
| Fig. 5 (A) Combined MALDI-TOF mass spectra of 3mPEG15-b-uPMAs. (B) GPC results for 3mPEG15-b-uPMAs indicating the absence of precursors left. | |
Table 2 Molecular weight analysis of 3mPEG15-b-uPMAs
Entry |
MALDI-TOF |
GPC |
Calculated ([M + Na]+) |
Found ([M + Na]+) |
Mna (kDa) |
Mwa (kDa) |
PDIa |
GPC was calibrated with a linear PS standard kit. |
3mPEG15-b-MA64 |
10 834.62 |
10 835.29 |
10.54 |
10.86 |
1.03 |
3mPEG15-b-MA96 |
15 123.80 |
15 125.33 |
14.01 |
14.43 |
1.03 |
3mPEG15-b-MA104 |
16 196.09 |
16 197.48 |
15.38 |
16.00 |
1.04 |
3mPEG15-b-MA112 |
17 268.39 |
17 267.08 |
15.53 |
16.15 |
1.04 |
3mPEG15-b-MA128 |
19 412.98 |
19 409.16 |
17.58 |
18.11 |
1.03 |
3mPEG15-b-MA144 |
21 557.57 |
21 584.07 |
18.04 |
19.30 |
1.07 |
3mPEG15-b-LMA32 |
6545.45 |
6526.73 |
7.15 |
7.36 |
1.03 |
Dendritic–linear block copolymers with uniform molecular weight, incorporating 3mPEG15 and uPMAs, were employed for solution self-assembly. Water was introduced (1 mL h−1) directly into the organic solvent containing the block copolymer (5 mg mL−1 in acetone), and phase transitions were monitored after dialysis in pure water for 24 h. As the molecular weight of uPMA increased, the self-assembled structures, analyzed by TEM and SEM, displayed a transition from cylindrical micelles to inverse cubic phases (Fig. 6). Compared to the use of dendritic–linear block copolymers containing polydisperse PEG and PS, low molecular weight uPMA exhibited an inverse cubic phase due to its high density (ρPMA = 1.25 vs. ρPS = 1.05 g cm−3)5 and high molecular weight of monomer, resulting in high packing parameter with similar molecular weights. In the range of 104 to 128 repeating units, a difference of 8 to 16 repeating units induced a phase transition in solution self-assembly. In the case of solution self-assembly of 3mPEG15-b-MA112 and 3mPEG15-b-MA128, Im3m phases were observed (Fig. 6D–E, G and H). In the case of 3mPEG15-b-MA144, phase transition from Im3m to Pn3m was observed, and the number of pores on the surface of particles reduced (Fig. 6F and I). In the range of 112 to 128 repeating units with acetone and 20% dioxane condition, Im3m phases were observed with particle and chunk formations in TEM and SEM images (Fig. S37 and S38†).
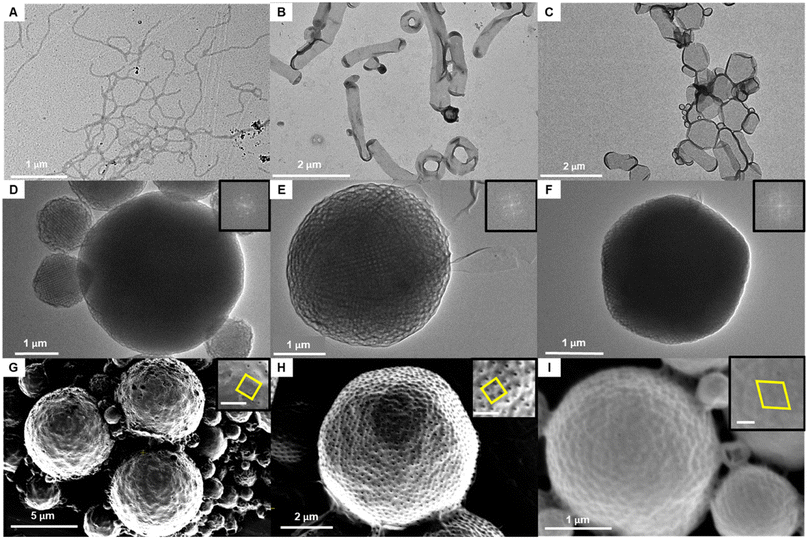 |
| Fig. 6 Phase transition of self-assembled structures of 3mPEG15-b-uPMA during solution self-assembly with increasing molecular weight of uPMA block. TEM images of (A) cylindrical micelle of 3mPEG15-b-MA64. (B) Flat bilayer and toroid of 3mPEG15-b-MA96. (C) Flat bilayer of 3mPEG15-b-MA104. (D) Polymeric cubosomes of 3mPEG15-b-MA112. (E) Polymeric cubosomes of 3mPEG15-b-MA128. (F) Polymeric cubosomes of 3mPEG15-b-MA144. The inset shows the fast Fourier transform (FFT) images. SEM images of (G) polymeric cubosomes of 3mPEG15-b-MA112 in microscopic scale. (H) Polymeric cubosomes of 3mPEG15-b-MA128 in microscopic scale. (I) Polymeric cubosomes of 3mPEG15-b-MA144 in microscopic scale. The insets show each patterns of pores on the surfaces. Scale bar is 200 nm. | |
To reduce the rate of water addition to the polymer solution,29 we conducted solvent diffusion-evaporation-mediated self-assembly (SDESA) for 3mPEG15-b-MA96, 3mPEG15-b-MA112, and 3mPEG15-b-MA128, using dioxane as the organic solvent. The organic solvent containing the block copolymer (0.5 mg mL−1) was placed in a small aluminum basket and located in a vial saturated with water. After 24 h, the organic solution became cloudy. Phase transitions were monitored after dialysis in pure water for 24 h. Comparatively, SDESA of 3mPEG15-b-MA96 exhibited a phase transition from flat bilayers to a sponge phase, while 3mPEG15-b-MA112 maintained Im3m symmetry (Fig. 7A and B). In contrast, 3mPEG15-b-MA128 underwent a transition from Im3m to Pn3m phases with angular shapes (Fig. 7C). Calculated from diameter measurements, the polydispersity index (PDI) of each block copolymer in SDESA displayed narrow values (PDI < 1.10, Fig. 7G–I and Table S8†).
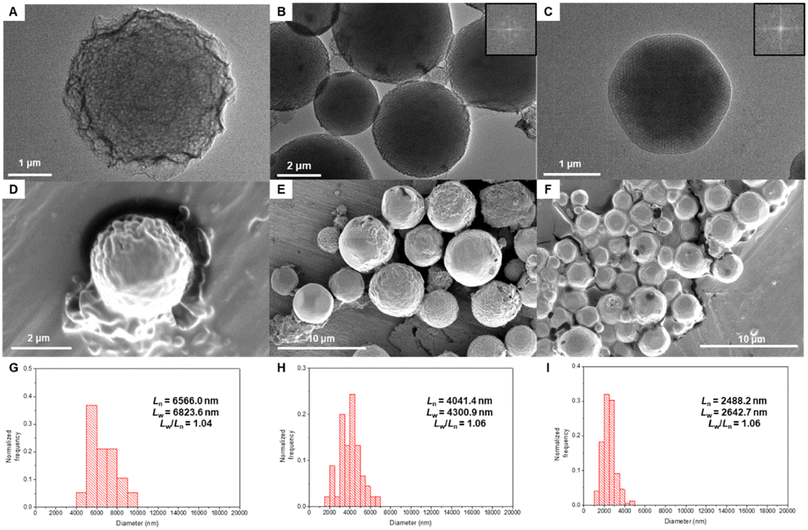 |
| Fig. 7 Phase transition of self-assembled structures of 3mPEG15-b-uPMA during solvent diffusion-evaporation self-assembly (SDESA) with increasing molecular weight of the uPMA block. TEM images of (A) sponge phase of 3mPEG15-b-MA96. (B) Polymeric cubosomes of 3mPEG15-b-MA112. (C) Polymeric cubosomes of 3mPEG15-b-MA128. The inset shows the fast Fourier transform (FFT) images. SEM images of (D) sponge phase of 3mPEG15-b-MA96 in the microscopic scale. (E) Polymeric cubosomes of 3mPEG15-b-MA112 in the microscopic scale. (F) Polymeric cubosomes with angular shapes of 3mPEG15-b-MA128 in microscopic scale. Histograms of diameter distribution in SDESA. (G) 3mPEG15-b-MA96; average diameter is 6566.0 nm, and PDI is 1.04. (H) 3mPEG15-b-MA112; average diameter is 4041.4 nm and PDI is 1.06. (I) 3mPEG15-b-MA128; average diameter is 2488.2 nm and PDI is 1.06. | |
Conclusion
In summary, we successfully synthesized discrete poly(mandelic acid) with up to 144 repeating units and stereospecific discrete oligo(mandelic acid), including 32 repeating units of discrete poly(L-mandelic acid), using an iterative convergent method. Our research demonstrates that the iterative convergent method offers a means to synthesize poly(mandelic acid) with minimal polydispersity and precise control over stereochemistry, leading to the synthesis of isotactic and syndiotactic oligo(mandelic acid)s. Additionally, we synthesized dendritic–linear discrete 3mPEG-b-uPMA block copolymers. Our findings reveal that an increase in the hydrophobic block chain length, achieved by increasing uPMA repeating units, results in self-assemblies with higher-curvature structures and smaller molecular weights compared to using PS. These results indicate that the morphologies of block copolymers can be finely controlled by adjusting block ratios with an exact number of repeating units, utilizing discrete polymers.
Conflicts of interest
There are no conflicts to declare.
Acknowledgements
This work was supported by National Research Foundation of Korea (NRF) grant funded by the Korean Government (MSIT) (NRF-2022R1A2C3013240) and the Creative Materials Discovery Program through the National Research Foundation of Korea funded by Ministry of Science and ICT (NRF-2020M3D1A1110504). We also thank Su Bin Park for help with synthesis of octameric discrete poly(ethylene glycol) and Jae Hak Lee for use of HR-SEM.
References
- H. K. Jeswani, M. R. Perry, M. P Shaver and A. Azapagic, Sci. Total Environ., 2023, 903, 166311 CrossRef CAS PubMed
. - H. Nakajima, P. Dijkstra and K. Loos, Polym, 2017, 9, 523 Search PubMed
. - A. Pellis, M. Malinconico, A. Guarneri and L. Gardossi, Nano Biotechnol., 2021, 60, 146–158 CrossRef CAS PubMed
. - A. G. Pinkus, R. Subramanyam, S. L. Clough and T. C. Lairmore, J. Polym. Sci., Part A: Polym. Chem., 1989, 27, 4291–4296 CrossRef CAS
. - T. Liu, T. L. Simmons, D. A. Bohnsack, M. E. Mackay, M. R. Smith and G. L. Baker, Macromolecules, 2007, 40, 6040–6047 CrossRef CAS
. - A. Buchard, D. R. Carbery, M. G. Davidson, P. K. Ivanova, B. J. Jeffery, G. I. Kociok-Köhn and J. P. Lowe, Angew. Chem., Int. Ed., 2014, 53, 13858–13861 CrossRef CAS PubMed
. - Y. Xu, M. R. Perry, S. A. Cairns and M. P. Shaver, Polym. Chem., 2019, 10, 3048–3054 RSC
. - M. Li, Y. Tao, J. Tang, Y. Wang, X. Zhang, Y. Tao and X. Wang, J. Am. Chem. Soc., 2018, 141, 281–289 CrossRef PubMed
. - J. Jiang, Y. Cui, Y. Lu, B. Zhang, X. Pan and J. Wu, Macromolecules, 2020, 53, 946–955 CrossRef CAS
. - Y. Wang and T. Q. Xu, Macromolecules, 2020, 53, 8829–8836 CrossRef CAS
. - G. L. Baker, E. B. Vogel and M. R. Smith III, Polym. Rev., 2008, 48, 64–84 CrossRef CAS
. - J. A. Gerlt, J. W. Kozarich, G. L. Kenyon and P. G. Gassman, J. Am. Chem. Soc., 1991, 113, 9667–9669 CrossRef CAS
. - M. Vagenende, G. J. Graulus, J. Delaey, J. Van Hoorick, F. Berghmans, H. Thienpont, S. van Vlierberghe and P. Dubruel, Eur. Polym. J., 2019, 118, 685–693 CrossRef CAS
. - Y. Kwon and K. T. Kim, Macromolecules, 2021, 54, 10487–10498 CrossRef CAS
. - J. Jiang, Y. Cui, Z. Jia, X. Pan and J. Wu, Macromolecules, 2021, 54, 2232–2241 CrossRef CAS
. - H. Tsuji, S. Nogata, H. Gamo, K. Hikima, A. Matsuda and Y. Arakawa, Polym. Degrad. Stab., 2022, 195, 109803 CrossRef CAS
. - L. MacFarlane, C. Zhao, J. Cai, H. Qiu and I. Manners, Chem. Sci., 2021, 12, 4661–4682 RSC
. - Q. Shi, C. Song, M. Chen, J. Xu, S. Zheng, J. Tan, J. Zhang, N. Wang, J. Hu and S. Liu, J. Am. Chem. Soc., 2023, 145, 23176–23187 CrossRef CAS PubMed
. - J. C. Worch, H. Prydderch, S. Jimaja, P. Bexis, M. L. Becker and A. P. Dove, Nat. Rev. Chem, 2019, 3, 514–535 CrossRef CAS
. - D. Bandelli, J. Alex, C. Weber and U. S. Schubert, Macromol. Rapid Commun., 2020, 41, 1900560 CrossRef CAS PubMed
. - M. Karayianni and S. Pispas, J. Polym. Sci., 2021, 59, 1874–1898 CrossRef CAS
. - N. A. Lynd, A. J. Meuler and M. A. Hillmyer, Prog. Polym. Sci., 2008, 33, 875–893 CrossRef CAS
. - S. Ha and K. T. Kim, Polym. Chem., 2019, 10, 5805–5813 RSC
. - M. E. Vleugels, M. E. De Zwart, J. R. Magana, B. A. Lamers, I. K. Voets, E. W. Meijer, K. Petkau-Milroy and A. R. Palmans, Polym. Chem., 2020, 11, 7170–7177 RSC
. - D. J. Adams, M. F. Butler and A. C. Weaver, Langmuir, 2006, 22, 4534–4540 CrossRef CAS PubMed
. - A. L. Schmitt, M. H. Repollet-Pedrosa and M. K. Mahanthappa, ACS Macro Lett., 2012, 1, 300–304 CrossRef CAS PubMed
. - Y. La, C. Park, T. J. Shin, S. H. Joo, S. Kang and K. T. Kim, Nat. Chem., 2014, 6, 534–541 CrossRef CAS PubMed
. - T. H. An, Y. La, A. Cho, M. G. Jeong, T. J. Shin, C. Park and K. T. Kim, ACS Nano, 2015, 9, 3084–3096 CrossRef CAS PubMed
. - C. Park, Y. La, T. H. An, H. Y. Jeong, S. Kang, S. H. Joo, H. Ahn, T. J. Shin and K. T. Kim, Nat. Commun., 2015, 6, 6392 CrossRef CAS PubMed
. - Y. La, T. H. An, T. J. Shin, C. Park and K. T. Kim, Angew. Chem., Int. Ed., 2015, 54, 10483–10487 CrossRef CAS PubMed
. - A. M. Wawro, T. Muraoka, M. Kato and K. Kinbara, Org. Chem. Front., 2016, 3, 1524–1534 RSC
.
|
This journal is © The Royal Society of Chemistry 2024 |
Click here to see how this site uses Cookies. View our privacy policy here.