DOI:
10.1039/D4RA02571G
(Paper)
RSC Adv., 2024,
14, 16560-16573
Cool green-emissive Y2Si2O7:Tb3+ nanophosphor: auto-combustion synthesis and structural and photoluminescence characteristics with good thermal stability for lighting applications†
Received
5th April 2024
, Accepted 26th April 2024
First published on 22nd May 2024
Abstract
A cheap, versatile, sustainable and energy-efficient gel-combustion method was applied to develop a series of green-emitting down-converted Y2Si2O7:Tb3+ (YPS:Tb3+) nanophosphors. Employing XRD-based Rietveld refinement approach, the phase purity and crystallographic evaluation of the produced phosphor were conducted, revealing a triclinic crystal with P
space group. EDX and TEM analyses were performed on the synthesized samples to determine their elemental composition and morphological properties. Diffuse reflectance spectra yielded 5.61 eV and 5.79 eV optical energy band gaps for the host and the optimized (0.04 mole of Tb3+) sample, respectively. UV light has the ability to excite the nanocrystalline phosphor in an efficient manner, leading to significant luminosity qualities attributed to the radiative relaxation of 5D4 → 7FJ (J = 6, 5, 4, 3). The bi-exponential decay function was derived by the PL decay curves. With an activation energy of 0.2206 eV, the Y1.96Si2O7:0.04Tb3+ phosphor exhibits good thermal quenching capabilities. Improved photometric attributes including CIE coordinates, CCT and color purity confirmed the green glow, indicating a strong competitor for cool-green emission in lighting applications.
1 Introduction
Conserving energy is crucial and has great significance for the well-being of future generations as it actively contributes to environmental preservation.1,2 In recent times, there has been a growing focus on the study of solid-state lighting, predominantly pc-WLEDs. This attention is driven by the need to raise awareness about energy consumption, conservation efforts, and the associated environmental challenges.3,4 WLEDs exhibit significant advantages in this context owing to their impressive features including high energy-efficacy, small power consumption, absence of mercury, extended lifespan, compact design, ecological friendliness, and superior illumination compared to conventional fluorescent lamps.5–8 In the present day, WLEDs have found extensive applications across diverse fields including optical waveguides, backlighting, sensors, automobile headlights, flashlights, solid-state lasers, and common lighting.9–13 Generally, commercial wLEDs are typically produced by coalescing blue-emanating InGaN-chips with yellow-emitting Y3Al5O12:Ce3+ nanophosphors.14,15 However, these WLEDs often lack a red module in the visible range, resulting in a white emission with a subpar color-rendering-index and high correlated-color-temperature.16 To address this issue, an alternative approach involves coating primary red, green, and blue nanophosphors onto a NUV-LED chip, aiming to achieve WLEDs with high rendering-index, proper CCT and adjustable color characteristics.17–19
Few studies have been conducted on yttrium orthosilicates (YSO) and for a long time, the focus of research on the luminous characteristics of RE-activated yttrium silicates was only on yttrium pyrosilicates (YPS). The magnetic and electrical characteristics of binary disilicates, particularly those of rare earth disilicates, have been thoroughly studied.20 Specifically, on activation with RE elements, these materials show optimal performance for luminous applications such as plasma displays, laser materials, and high energy nanophosphors.21 These phosphors provide very efficient luminescence when excited by UV and cathode rays. Because of their chemical stability, yttrium disilicates, also known as yttrium pyrosilicates (Y2Si2O7), are among the most suitable silicates.22 The photoluminescence of Ce3+ and Eu3+ was particularly explored with respect to the luminescence characteristics of rare earth-doped Y2Si2O7. Nowadays, the green phosphor is quite popular in the lighting industry. As a result, interest in an effective new green phosphor has grown. The green color-emitter trivalent terbium (Tb3+) is one of the rare earth ions that are most frequently employed as an active center. Its many emission peaks encompass a broad wavelength range from blue to orange light. The ground state spectrum characteristics of the Tb3+ ion are 7Fj (j = 0–6), while its outermost electron arrangement is 4f8. The 5D4 → 7Fj (j = 0–6) transitions are often the source of the distinctive green emission of Tb3+ ions, while the concurrently existing 5D3 → 7Fj transitions that produce blue or ultraviolet light are also conceivable.23,24 Moreover, the luminescence of 4f-electrons is mainly a characteristic inherent to the RE ions and remains unaffected by the crystal field surrounding the luminescent center. This is because the 4f-electrons within the inner layer of Tb3+ are protected by the outer electrons of 5s25p6. Consequently, it is believed that Tb3+-activated phosphors would show outstanding stability for the particular emission. This work presents a thorough exploration of the structural and optical features of Tb3+-doped Y2Si2O7 (YPS) phosphor that is produced using the gel-combustion process. X-ray diffraction, transmission electron microscopy, energy dispersive X-ray spectroscopy, diffuse reflectance spectroscopy and photoluminescence spectroscopy were employed to comprehensively examine the structural, morphological, and spectroscopic aspects of the prepared series of Y2−xSi2O7:xTb3+ (x = 0.01–0.06 mole) samples to ascertain the suitability of the prepared phosphors in pc-WLEDs.
2 Experimental
2.1 Synthesis and characterization
Employing ecologically-sound gel-combustion synthesis, triclinic symmetry type Y2−xSi2O7:xTb3+ phosphors with variable dopant ion content as x = 0.01–0.06 mole were developed, yielding a product that exhibits excellent crystallinity and homogeneity (Fig. 1). For the implementation of this procedure, precise amounts of [Y(NO3)3·6H2O], [Tb(NO3)3·6H2O], silica (SiO2), urea and nitric acid (HNO3) were employed. The goal is to maintain a metal nitrate-to-fuel ratio of nearly one. Subsequently, the beaker containing the previously prepared mixture was placed on a hot plate adjusted to 80 °C and warmed for 20 minutes. This step is crucial to ensure the effective infiltration of activator ions (Tb3+) into the Y2Si2O7 matrix. Following that, the uniform mixture is initially warmed and subsequently transferred to a muffle furnace preheated to 600 °C for a duration of 15 minutes. During this process, a self-restricted exothermic reaction occurs, resulting in the release of combustible gases (CO2 and N2).25,26 This reaction contributes to the formation of a fluffy powder. Subsequently, the obtained powders were finely grounded, after which they were transferred to an alumina crucible for sintering at 1100 °C for 3 hours. To evaluate the phase purity of the produced phosphors, X-ray diffraction examination was performed using a Rigaku Ultima X-ray diffractometer running at 40 kV and 40 mA with Cu-Kα radiation. Using BaSO4 as the reference standard, an integrated sphere-mounted UV-Vis-NIR spectrophotometer (UV-3600, SHIMADZU) was used to produce the diffuse reflection spectra. Using a transmission electron microscope (JEOL JEM-1400 Plus) running at 120 kV, the morphology of the doped nanopowders was examined. A Hitachi SU-8010 SERIES device was used to carry out the energy dispersive X-ray analysis. Additionally, using a Fluorolog-3-Horiba spectrophotometer fitted with a 150 W xenon lamp, the material's emission and excitation spectra were obtained.
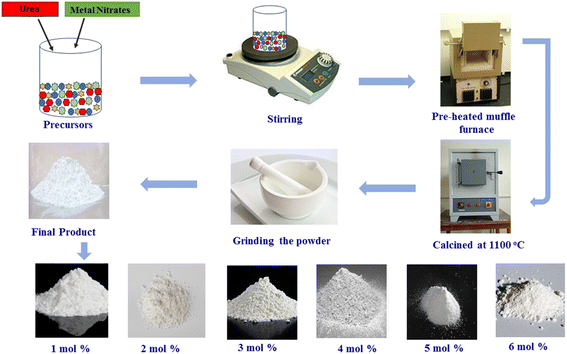 |
| Fig. 1 Synthesis diagram of the gel-combustion process. | |
3 Results and discussion
3.1 XRD evaluation
XRD is an approach employed for the structural assessment of the produced phosphors. The resulting XRD patterns for the undoped and Y2−xSi2O7:xTb3+ (x = 0.01–0.06 mole) phosphors are depicted in Fig. 2(a). It was found that the comparable intensity of Tb3+-triggered phosphors and undoped phosphors is essentially indistinguishable. The XRD lines of the Y2Si2O7 and Y2−xSi2O7:xTb3+ (x = 0.01–0.06 mole) phosphors were verified via the standard JCPDS card [38-0223], which followed the standard pattern of the Y2Si2O7 (YPS) material with triclinic phase and P
space group.27,28 It is conceivable to conclude the emergence of single-phase crystal structure since there are no diffraction peaks from the dopant or other phases. XRD patterns remain essentially the same, but a little shift in the diffractions lines toward lower angles, as seen in Fig. 2(b), suggests a propensity for the lattice to expand as the dopant ion's concentration increases. The interplanar distance (d) increases on doping, as listed in Table 1. In order to keep nλ constant in Bragg hypothesis (2d
sin
θ = nλ), the angle (θ) determining their left shift in this case would have to be smaller.29 The reliable doping of dopant ions in the phosphors may often be assessed by the ion radius resemblance method and the valence state. Another useful technique is to calculate the percentage of variance in the ionic radius of the dopant and the substituted ions. Less than 30% of a percentage difference generally implies the efficient doping of dopant ions.30 The following eqn (1) may be used to get the percentage difference.31 |
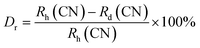 | (1) |
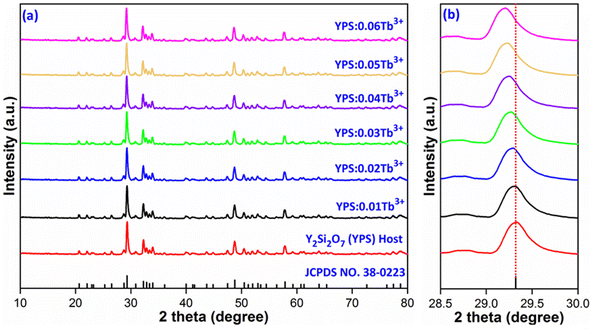 |
| Fig. 2 (a) Diffraction patterns of Y2Si2O7 and Y2−xSi2O7:xTb3+ (x = 0.01–0.06 mole) phosphors. (b) Enlarged pattern view of all the considered samples. | |
Table 1 Interplanar d-spacing values of the host and all Tb3+-doped phosphorsa
Sample |
2θ-Angle |
d-Spacing (Å) |
Data in () shows the estimated uncertainties. |
Y2Si2O7 (YPS) |
29.32 |
3.0437 (±0.0094) |
YPS:0.01Tb3+ |
29.31 |
3.0447 (±0.0068) |
YPS:0.02Tb3+ |
29.30 |
3.0458 (±0.0072) |
YPS:0.03Tb3+ |
29.28 |
3.0477 (±0.0066) |
YPS:0.04Tb3+ |
29.25 |
3.0508 (±0.0069) |
YPS:0.05Tb3+ |
29.23 |
3.0528 (±0.0085) |
YPS:0.06Tb3+ |
29.20 |
3.0559 (±0.0081) |
In the above relation, Rh(CN) & Rd(CN) stand for the host and entering cation's respective ionic radii as well as coordination number. The results show that the Dr-value is less than 30%, which suggests that the Tb3+ ion has successfully replaced the Y3+ ion in the Y2Si2O7 host. Scherrer is acknowledged for formulating Scherrer's equation, a foundational method in X-ray crystallography used to calculate the average size of crystalline solid materials. It correlates the size of the crystalline domains to the widening of diffraction peaks noted in the X-ray diffraction patterns. The Scherrer's formula is represented by eqn (2).32
|
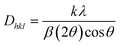 | (2) |
In the above formula, λ, β and k represent the wavelength of X-rays (0.154 nm), full width at half-maxima and Scherrer constant (0.89), respectively. The computed crystallite size for all the prepared samples is summarized in Table 2. The following eqn (3), particularly based on the Williamson–Hall formulation, was implemented to corroborate the crystallite size findings described above.33
|
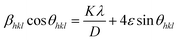 | (3) |
Table 2 Diffraction results of the Y2Si2O7 and Y2−xSi2O7:xTb3+ (x = 0.01–0.06 mole) phosphorsa
Sample (YPS) |
2 theta (2θ) |
FWHM |
Crystallite size (nm) |
Microstrain (ε × 10−4) |
Scherrer's |
W–H |
Data in () shows the estimated uncertainties. |
Y2Si2O7 (YPS) |
29.32 |
0.2997 (±0.00451) |
27.40 (±0.0431) |
35.58 (±0.0746) |
5.1421 |
YPS:0.01Tb3+ |
29.31 |
0.3012 (±0.00341) |
27.26 (±0.0254) |
34.42 (±0.0879) |
5.4532 |
YPS:0.02Tb3+ |
29.30 |
0.3032 (±0.00375) |
27.09 (±0.0233) |
33.97 (±0.0748) |
5.8315 |
YPS:0.03Tb3+ |
29.28 |
0.3071 (±0.00377) |
26.74 (±0.0371) |
33.12 (±0.0852) |
6.0345 |
YPS:0.04Tb3+ |
29.25 |
0.3142 (±0.00314) |
26.15 (±0.0322) |
32.54 (±0.0901) |
6.2931 |
YPS:0.05Tb3+ |
29.23 |
0.3188 (±0.00401) |
25.76 (±0.0298) |
31.77 (±0.0876) |
6.6214 |
YPS:0.06Tb3+ |
29.20 |
0.3219 (±0.00372) |
25.52 (±0.0286) |
31.04 (±0.0699) |
6.8891 |
This method considers the intricate effects of crystallite size and strain on the XRD peaks. It allows for a clear separation of the effects of strain-induced deformation from those caused by the crystallite size, which facilitates more accurate evaluations and interpretations of the material properties. Employing linear fitting on the curve plotted between 4
sin
θ and β
cos
θ, as illustrated in Fig. 3, the crystallite size and strain induced in the phosphor can be determined. The intercept of the linear curve provides an insight into the crystallite size, while the slope offers information about the strain present in the material. This analytical method facilitates a straightforward extraction of both the crystallite size and strain parameters from the X-ray diffraction data. The crystal lattice experienced strain as a result of the replacement of Y3+ ions with the larger Tb3+ ions. This strain may have limited the size of the particles by lowering their total free energy. The obtained results are listed in Table 2.
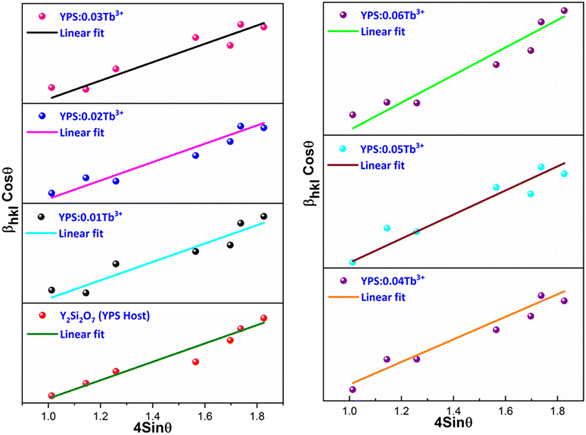 |
| Fig. 3 W–H plot of the Y2Si2O7 and various doped Y2−xSi2O7:xTb3+ (x = 0.01–0.06 mole) phosphors. | |
A strong method for determining a material's crystal structure from powder X-ray diffraction data is referred to as Rietveld refinement. Through the process of Rietveld refinement, atomic locations, lattice parameters and other structural features of crystalline materials may be precisely determined by comparing the computed patterns based on a suggested crystal structure with the experimental XRD data. The Rietveld refinement patterns for the host and optimized doped phosphors are displayed in Fig. 4(a) and (b). The chi-squared values and reliability factors being within the acceptable range show that the modified profiles show significant agreement with the original structural model. During the refinement process, it becomes evident that the resulting nanophosphor possesses a triclinic phase with the point group symmetry identified as P
. The outcomes indicate a favorable alignment between the observed and calculated XRD patterns, as evidenced by parameters such as Rwp, Rp and χ2, which fall within acceptable ranges. The refined results, including the lattice parameters, are compiled in Table 3. The convergence of reliability factors yielded Rwp values of 7.59% for YPS and 8.42% for YPS:0.04Tb3+, as well as Rp values of 5.77% for YPS and 6.21% for YPS:0.04Tb3+. These values affirm the rationality and reliability of the obtained results. The cell parameters for YPS are indeed smaller than those for the YPS:0.04Tb3+ phosphor, as indicated in Table 3. This discrepancy provides additional evidence supporting the successful substitution of Tb3+ ions into the Y3+ position within the lattice, leading to an expansion of the lattice constants.
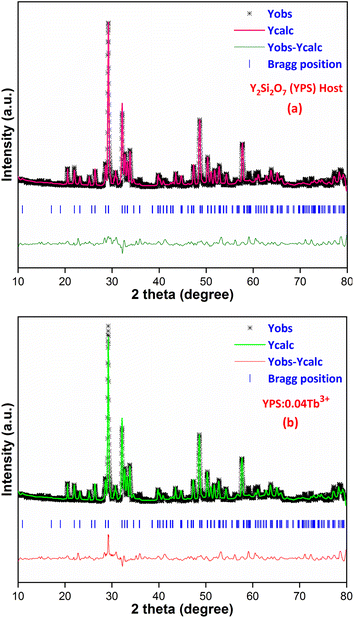 |
| Fig. 4 Rietveld profiles of the (a) Y2Si2O7 and (b) Y1.96Si2O7:0.04Tb3+ phosphors. | |
Table 3 Refinement outcomes of the Y2Si2O7 (YPS) and YPS:0.04Tb3+ phosphors
Sample |
Y2Si2O7 (YPS) |
YPS:0.04Tb3+ |
2θ range; step (deg.) |
10–80; 0.02 |
10–80; 0.02 |
System |
Triclinic |
Triclinic |
Lattice-type |
P |
P |
Wavelength (Å) |
1.541 |
1.541 |
Space group |
P![[1 with combining macron]](https://www.rsc.org/images/entities/char_0031_0304.gif) |
P![[1 with combining macron]](https://www.rsc.org/images/entities/char_0031_0304.gif) |
Space group number |
2 |
2 |
Formula unit (Z) |
4 |
4 |
α |
97.23 |
97.41 |
β |
89.80 |
89.83 |
γ |
87.50 |
87.92 |
a (Å) |
6.556 |
6.591 |
b (Å) |
6.759 |
6.781 |
c (Å) |
12.274 |
12.619 |
Volume (Å3) |
539.03 |
558.89 |
χ2 |
3.14 |
3.97 |
Rp (%) |
5.77 |
6.21 |
Rwp (%) |
7.59 |
8.42 |
3.2 Morphological analysis
The TEM profile presented in Fig. 5 offers valuable insights into the structure of the prepared Y1.96Si2O7:0.04Tb3+ sample. The image vividly illustrates the existence of an aggregated nanocrystalline material characterized by diverse shapes and sizes. The observed aggregation of crystallites is likely attributed to temperature variations experienced during the fabrication process, as suggested by previous studies. Additionally, the porosity evident in the material is linked to the release of gassy by-products through the combustion process.34,35 This phenomenon contributes to the formation of a porous structure within the material. Notably, the size information obtained from diffraction studies aligns closely with the evaluated size derived from the TEM micrograph. Both analyses indicate a nanocrystalline range, specifically within the dimensions of 20–40 nm. This congruence between the XRD and TEM results reinforces the reliability of the characterization, affirming the nanocrystalline nature of the Y2Si2O7:Tb3+ sample.
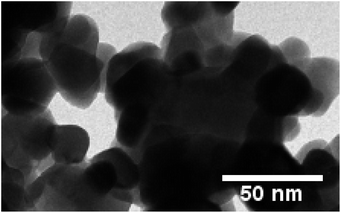 |
| Fig. 5 TEM micrograph of the YPS:0.04Tb3+ nanophosphor. | |
3.3 EDX investigation
When a high energy electron beam strikes a sample, it induces the emission of characteristic X-rays from the elements present in the sample. The energy of these X-rays is characteristic of the elements, allowing for the identification and quantification of the elemental composition. The EDX plots of the host Y2Si2O7 (YPS) and 0.04 mole-doped YPS nanophosphor are shown in Fig. 6(a) and (b). Numerous peaks that are associated with different elements (Y, Si and O) integrated with the host YPS framework can be seen in the spectrum in Fig. 6(a). The peaks in Fig. 6(b) correspond to Y, Si, O and Tb, further validating the produced phosphor's chemical compositions. The Tb characteristic peaks demonstrate the homogeneous doping of ions in the host matrix. The elemental compositions of the doped sample and the host are shown in the table present in the inset of Fig. 6(a) and (b). The formation of pure Y2Si2O7 and Y2Si2O7:Tb3+ phosphor is validated by the elements individually, which reflects the findings of XRD.
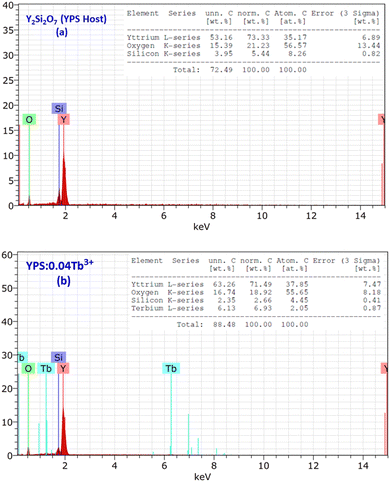 |
| Fig. 6 EDX profiles of the (a) Y2Si2O7 and (b) Y1.96Si2O7:0.04Tb3+ phosphors, and the inset represents the chemical composition of the respective samples. | |
3.4 Photoluminescence study
3.4.1 Excitation and emission spectra. Fig. 7 illustrates the photoluminescence excitation (PLE) spectrum for Y1.96Si2O7:0.04Tb3+ in the wavelength region of 200–500 nm by fixing the emission at 545 nm. The 4f8 → 4f75d1 transition of Tb3+ ions is responsible for the peak that is centered at 258 nm. Additionally, the spectrum disclosed numerous peaks at 301 nm, 325 nm, 347 nm, 367 nm, 386 nm and 489 nm, which originated from the ground state 7F6 and moved to the higher states 5H6, 5H7, 5L6, 5L9, 5G6 and 5D4, respectively.36,37 To investigate the PL characteristics of Y2Si2O7:Tb3+ nanophosphors in more detail, the PL emission spectra of powdered YPS:xTb3+ (x = 0.01–0.06 mole) nanocrystalline samples were captured at an excitation wavelength of 258 nm, as clearly illustrated in Fig. 8. Numerous emission peaks that correlate to the distinctive Tb3+ ion emission in the YPS host matrix can be seen in the spectra. In essence, emission transitions to distinct 7FJ (J = 2 to 6) energy levels occur from two emission states, 5D3 and 5D4. The 5D3 to 7F5, 7F4 and 7F3 transitions deliver emission peaks at 414 nm, 438 nm and 460 nm, correspondingly. However, four distinct luminous bands of Tb3+ ion are characterized and located at about 491 nm (5D4 → 7F6), 544 nm (5D4 → 7F5), 589 nm (5D4 → 7F4) and 624 nm (5D4 → 7F3).38,39 The emission peak at 491 nm is the only one that is allowed to be electric-dipole (ED); however, the transitions associated with the other three emission peaks are permitted to be both electric and magnetic-dipole (ED + MD).40 According to research, the green emission of the YPS:xTb3+ (x = 0.01–0.06 mole) nanophosphor is caused by the strongest emission peak that coincides with the 5D4 → 7F5 (544 nm) transition. The energy states corresponding to the Tb3+ ions in the Y2Si2O7 host materials are displayed in Fig. 9.
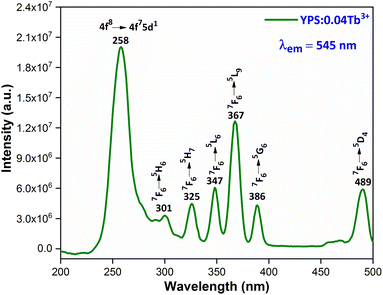 |
| Fig. 7 Excitation spectrum of the Y1.96Si2O7:0.04Tb3+ nanophosphor. | |
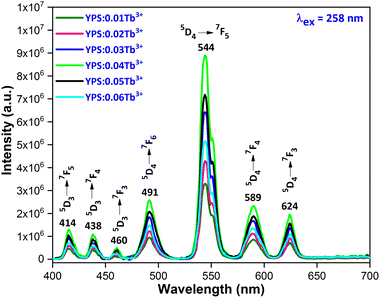 |
| Fig. 8 Emission spectrum of the Y2−xSi2O7:xTb3+ (x = 0.01–0.06 mole) phosphors. | |
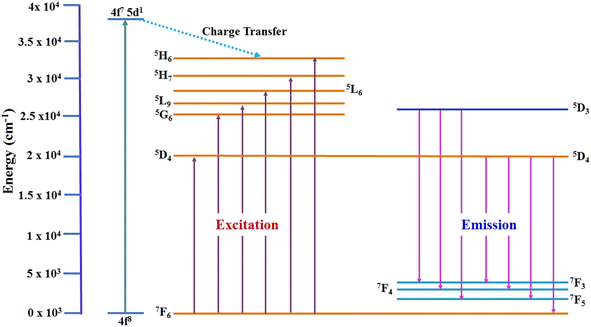 |
| Fig. 9 Energy level diagram for Tb3+ ions in the Y2Si2O7 nanophosphor. | |
3.4.2 Concentration quenching (CQ). To determine the most effective doping concentration for Tb3+ ions in YPS:xTb3+ (0.01–0.06 mole) phosphors, we analyze the variation in the integrated emission intensity with respect to the Tb3+ concentration (Fig. 10). The findings indicate that as the doping content increases from 0.01 mole to 0.04 mole, the emission intensity initially increases, reaching its maximum at x = 0.04 mole, and subsequently diminishes. This implies that the optimum amount of Tb3+ ions within the YPS host is 0.04 mole, after which the luminous intensity declines due to concentration quenching. This phenomenon, commonly observed in Tb3+ ions-doped inorganic phosphors, is characterized by increased cross-relaxation and energy relocation amid nearby Tb3+ ions at higher doping concentrations. Eventually, a portion of the excited energy of Tb3+ ions is absorbed by nearby quenching centers, resulting in a decline in the emission intensity. The concentration quenching effect primarily arises from nonradiative energy transfer among Tb3+ activators, assisted via three pathways: radiation reabsorption, exchange interaction and electric multipolar interaction. Our focus will be on elucidating the mechanism behind CQ in the YPS:Tb3+ phosphors. As both exchange interaction and multipolar interaction are influenced by the critical distance (Rc) between Tb3+ ions, we analyzed Rc for YPS:Tb3+ nanophosphors via eqn (4) suggested by Blasse.41 |
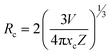 | (4) |
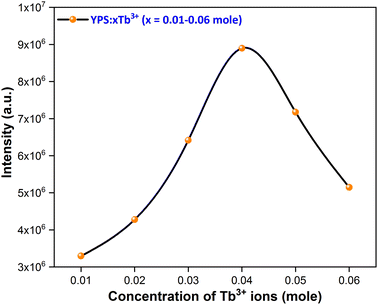 |
| Fig. 10 Concentration quenching profile of the considered nanophosphors. | |
In this context, Rc represents the separation among adjacent Tb3+ ions at optimal content, V denotes volume, xc denotes the optimal amount of dopants and Z signifies cations per unit cell. Given the values V = 558.89 Å3, xc = 0.04 and Z = 4, the calculated Rc is approximately 18.828 Å, exceeding the 5 Å threshold. If the critical distance is greater than 5 Å, then multipolar interaction us dominant over exchange interaction. In this scenario, the energy transfer is solely driven by electric multipolar interaction. As the doping amount of terbium ions rises, the distance between Tb3+ ions shrinks, thus enhancing the probability of energy transfer between them. The relationship between log(I/x) and log(x) for YPS:Tb3+ nanophosphors was evaluated using eqn (5) below.42
|
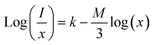 | (5) |
In above equation, x represents the doping concentration of Tb3+ ions, I signifies the PL intensity of samples, and M denotes the electric multipole index. The values of M such as M = 6, 8 and 10 correspond to dipole–dipole, dipole–quadrupole and quadrupole–quadrupole interactions, respectively.43,44 In Fig. 11, the graph between log(I/x) and log(x) is depicted, and the slope of the fitted line is determined to be −2.28. Consequently, the calculated value of M is 6.48, agreeing closely with the value of 6. Hence, the dipole–dipole interaction is identified as the primary reason responsible for the CQ effect in the Y2Si2O7:Tb3+ phosphors.
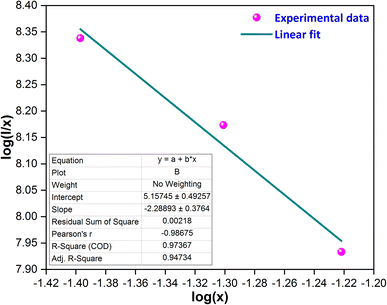 |
| Fig. 11 Straight line fitted graph between log(x) and log(I/x). | |
3.5 Luminescence lifetime
The decay curves for the 5D4 level were recorded at room temperature, employing 258 nm excitation for the Y1.96Si2O7:0.04Tb3+ phosphor, as illustrated in Fig. 12. Also, the inset of Fig. 12 contains the log-based lifetime graph for the considered sample. The experimental decay curve data were fitted using various exponential equations, and the most optimal fit was achieved with the bi-exponential eqn (6).45 |
It = I0 + A1 exp (−t/τ1) + A2 exp(−t/τ2)
| (6) |
here, It and I0 denote luminescence intensities at time t and 0, respectively. τ1 and τ2 correspond to the values of the lifetime for the fast and slow exponential components, respectively. Additionally, A1 and A2 are constants associated with the parameters for curve fitting. Formula (7) was used to determine the average lifetime for the fabricated phosphors.46 |
τavg = (A1t12 + A2t22)/(A1t1 + A2t2)
| (7) |
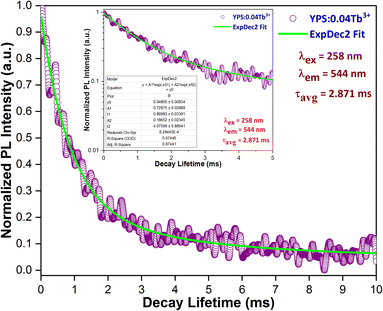 |
| Fig. 12 Lifetime curve of the Y1.96Si2O7:0.04Tb3+ nanophosphor. | |
Table 4 displays the average decay lifetimes (τavg) for all the synthesized doped samples. Notably, it is evident that the decay times exhibit a decrease with an increase in the doping amount of the trivalent terbium ion. This phenomenon can be attributed to the increasing concentration of dopant ions, causing them to be in closer proximity. Consequently, there is a swift transfer of energy between ions, leading to different decay paths and ultimately resulting in a reduced decay lifetime. Fig. 13 further illustrates that the τo value was found to be 4.339 ms, as determined through Auzel's fitting using eqn (8).47
|
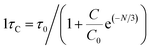 | (8) |
Table 4 Decay time and quantum efficiency of the Y2−xSi2O7:xTb3+ (x = 0.01–0.06 mole) phosphorsa
Sample |
τavg (ms) |
AnR (S−1) |
(η%) |
Data in () shows the estimated uncertainties. |
YPS:0.01Tb3+ |
3.73 (±0.014) |
37.69 |
85.96 |
YPS:0.02Tb3+ |
3.49 (±0.015) |
56.13 |
80.43 |
YPS:0.03Tb3+ |
3.17 (±0.012) |
85.05 |
73.06 |
YPS:0.04Tb3+ |
2.87 (±0.017) |
118.03 |
66.14 |
YPS:0.05Tb3+ |
2.52 (±0.013) |
166.42 |
58.07 |
YPS:0.06Tb3+ |
2.29 (±0.015) |
206.28 |
52.77 |
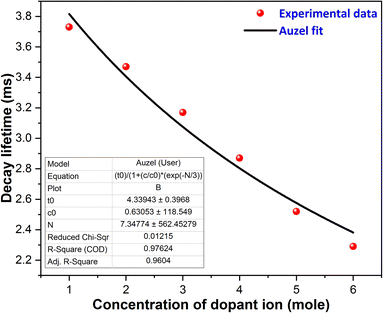 |
| Fig. 13 Auzel's fitting curve of the Y2−xSi2O7:xTb3+ (x = 0.01–0.06 mole) phosphors. | |
The quantum efficacy (η) of the produced materials was assessed by the ratio of experimental lifetime (τavg) to radiative lifetime value (τo), as given by eqn (9) below.48
The following formula (10) was employed to calculate the non-radiative transition rate (AnR) with the help of the experimental and radiative lifetime values.
|
 | (10) |
Table 4 contains the calculated values of lifetime, quantum efficiency and non-radiative transitions for all the synthesized YPS:xTb3+ (x = 0.01–0.06 mole) nanophosphors.
3.6 Optical band gap analysis
Fig. 14 illustrates the characteristic diffuse reflectance spectra (DRS) of both the host and the YPS:0.04Tb3+ nanophosphor in the 200–600 nm range. The doped sample exhibits an absorption band at 258 nm, corresponding to the f–d transition of Tb3+ ions. Additionally, the absorption peaks attributed to the f–f transitions of Tb3+ are observed in the longer wavelength spectral region, specifically at 325 nm (7F6 → 5H7), 367 nm (7F6 → 5L9) and 487 nm (7F6 → 5D4). To explore the impact of Tb3+ doping on the band gap (Eg) of the YPS host, the sample's acquired DRS data were transformed into optical absorption data employing the Kubelka–Munk formula illustrated in eqn (11).49 |
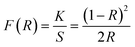 | (11) |
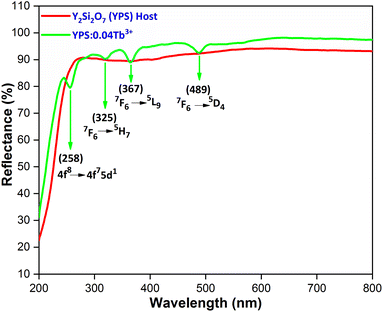 |
| Fig. 14 Diffusion reflectance spectra of the host and Y1.96Si2O7:0.04Tb3+ nanophosphor. | |
The symbols for the reflection coefficient, scattering coefficient, and molar absorption coefficient are K, S, and R, respectively. Eqn (12) explains the relationship between Eg and the absorption coefficient (α) using Tauc's formula.
|
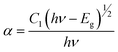 | (12) |
Band gap energy will be conceivable from eqn (11) and (12), which utilised formula (13).50
|
[F(R)hv] = C2(hν − Eg)1/2
| (13) |
The band gap (Eg) of the sample is indicated by extrapolating the resulting curve to the hν axis (x-axis), where [F(R)hν]2 = 0. This is achieved through a plot of [F(R)hν]2 versus hν, as illustrated in Fig. 15. The band gaps for the host YPS and YPS:0.04Tb3+ phosphor are 5.61 eV and 5.79 eV, respectively. Burstein–Moss (B–M) theory results in a continual rise of the optical energy gap from the host to the doped sample.
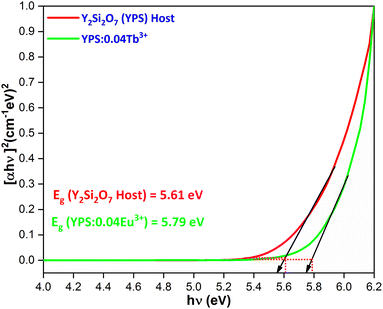 |
| Fig. 15 Tauc’s plot for the Y2Si2O7 and Y1.96Si2O7:0.04Tb3+ phosphor. | |
3.7 Temperature-dependent luminescence
The thermal stability of a phosphor at elevated temperatures is a crucial factor in applications involving solid-state lighting. The temperature-dependent photoluminescence spectra of the YPS:0.04Tb3+ phosphor were examined across a range of temperatures from 298 to 498 K, as illustrated in Fig. 16. The peak's shapes and locations in the spectra remained consistent as the temperature rose. A gradual reduction in intensity has been attributed to an increased occurrence of non-radiative transitions. To evaluate the impact of thermal quenching, the activation energy may be determined by Arrhenius eqn (14).51,52 |
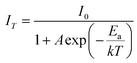 | (14) |
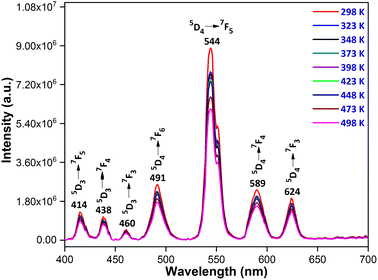 |
| Fig. 16 Temperature-dependent photoluminescence of the Y1.96Si2O7:0.04Tb3+ phosphor. | |
Eqn (14) is further modified as
|
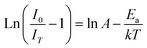 | (15) |
here,
Ea,
k,
IT and
I0 stand for activation energy, Boltzmann constant, intensity at temperature
T and original intensity, respectively. As depicted in
Fig. 17, a linear relationship is observed between ln[(
I0/
IT) − 1] and 1/
kT for the YPS:0.04Tb
3+ phosphor. In accordance with
eqn (15), the experimental data fits well with a linear trend, allowing the calculation of the activation energy (
Ea) for thermal quenching from the slope. The determined
Ea value for thermal quenching is 0.2206 eV, which is further supported by recent literature,
i.e., Ca
2LuHf
2Al
3O
12:Ce
3+, Tb
3+,
53 Ca
2YHf
2Al
3O
12:Ce
3+,Tb
3+,
54 Ca
14Al
10Zn
6O
35:Tb
3+,
55 Ba
3YB
3O
9:Tb
3+,
56 RbBaBP
2O
8:Tb
3+,
57 Ca
2Ga
2SiO
7:Tb
3+,
58 La
4GeO
8:Tb
3+, Eu
3+,
59 Ba
3(ZnB
5O
10)PO
4:Tb
3+,
60 Bi
3TeBO
9:Tb
3+61 and Na
5Lu
9F
32:Tb
3+.
62 In the present study, multiphonon-assisted nonradiative transition did not occur. The multiphonon-assisted nonradiative transition process is a mechanism that can contribute to quenching luminescence in rare-earth-doped materials. The multiphonon-assisted nonradiative transition typically occurs when there is a small energy gap between the excited state and lower-lying states. This energy gap might be relatively larger in Y
2Si
2O
7 doped with Tb
3+ ions, reducing the likelihood of multiphonon-assisted nonradiative transitions. There may be other nonradiative processes or quenching mechanisms more dominant in this system, such as cross-relaxation or energy transfer to defects. This outcome further suggests that the YPS:0.04Tb
3+ phosphor exhibits admirable thermal stability for white light-emitting diodes.
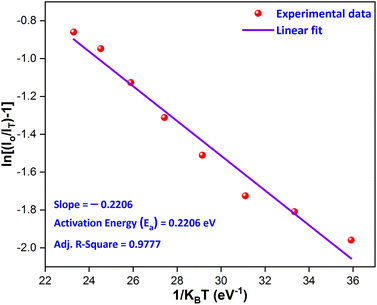 |
| Fig. 17 Linear fitted graph for the calculation of the activation energy. | |
3.8 Photometric investigation
The substance that emits light is called a phosphor and the kind of activator regulates the color that the phosphor emits. Assessing the material's photometric behavior is imperative.
(λ), ȳ(λ) and
(λ) are the color matching variables that are employed for assessing the color of the illumination. The values (X, Y and Z), representing the necessary stimulation levels to replicate a color from the spectral intensity, are provided by the eqn (16)–(18) below, where P(λ) represents the spectral power distribution of light.63 |
X = ∫ (λ)P(λ)dλ
| (16) |
|
Z = ∫ (λ)P(λ)dλ
| (18) |
The relative fraction of tristimulus values, or chromaticity coordinates, can be obtained via the resulting formula (19).
|
 | (19) |
The CIE coordinates of the YPS:xTb3+ (x = 0.01–0.06 mole) phosphors are located in the green area of the color gamut, as revealed in Fig. S1–S6.† The color purity (CP) in context of the nanophosphors typically refers to the ability of the nanophosphors to emit light of a specific color without any undesired spectral impurities or broadening. The color purity of the achieved phosphors was evaluated using the given formula (20).64,65
|
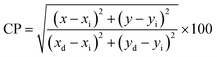 | (20) |
The CCTs in Kelvin (K) indicate the color advent of light output through an illumination source. Whether a light source appears warm or cool may be determined by examining its CCT. Cool white light is indicated by a CCT above 4000 K, whereas warm light is signified by a CCT below 3000 K. To calculate the CCT values, CIE points representing the light source were renewed to (u′, v′) by eqn (21) below. The subsequent step involved defining the temperature associated with the Planckian locus closest to the light source on the (u′, v′) uniform chromaticity chart portrayed in Fig. S7–S12.† This process allows for the identification of the temperature on the Planckian locus that is in proximity to the given light source's coordinates on the chromaticity diagram.66
|
 | (21) |
Additionally, McCamy's third-order polynomial was utilized for computing the CCT values based on the Kelvin (K) scale to assess the quality of light, given by eqn (22).67
|
CCT = −437n3 + 3601n2 − 6861n + 5514.31
| (22) |
here, (
x,
y) are CIE coordinates,
n = (
x −
xe)/(
y −
ye) is the inverse slope line, and
xe and
ye = 0.332 and 0.186 are the values of chromaticity epicenters. The values of photometric variables such as CIE, CP and CCT of all the synthesized Y
2−xSi
2O
7:
xTb
3+ (
x = 0.01–0.06 mole) phosphors are summarized in
Table 5.
Table 5 Chromaticity parameters of the Y2−xSi2O7:xTb3+ (x = 0.01–0.06 mole) phosphorsa
Sample |
(x, y) |
CP (%) |
(u′, v′) |
CCT (K) |
Data in () shows the estimated uncertainties. |
YPS:0.01Tb3+ |
0.3401, 0.5125 (±0.00011, 0.00012) |
56.4 (±0.26) |
0.1606, 0.5446 (±0.00013, 0.00014) |
5346.37 (±6.14) |
YPS:0.02Tb3+ |
0.3422, 0.5069 (±0.00013, 0.00011) |
55.3 (±0.21) |
0.1630, 0.5432 (±0.00012, 0.00011) |
5299.89 (±9.14) |
YPS:0.03Tb3+ |
0.3418, 0.5057 (±0.00013, 0.00010) |
54.8 (±0.25) |
0.1630, 0.5428 (±0.00014, 0.00016) |
5307.39 (±7.34) |
YPS:0.04Tb3+ |
0.3389, 0.5175 (±0.00012, 0.00011) |
57.5 (±0.27) |
0.1589, 0.5459 (±0.00012, 0.00014) |
5373.09 (±4.18) |
YPS:0.05Tb3+ |
0.3412, 0.5181 (±0.00014, 0.00014) |
58.4 (±0.24) |
0.1599, 0.5463 (±0.00012, 0.00014) |
5327.01 (±6.16) |
YPS:0.06Tb3+ |
0.3424, 0.5097 (±0.00015, 0.00012) |
56.2 (±0.32) |
0.1625, 0.5441 (±0.00014, 0.00013) |
5297.57 (±6.18) |
4 Conclusions
Nanophosphors composed of yttrium disilicates (Y2Si2O7) doped with terbium (Tb3+) were produced through a gel-combustion technique. The diffraction patterns of the phosphors indicated a triclinic single-phase structure. Using Scherrer's equation and W–H plot, the crystallite size was determined to be 24–36 nm, and TEM exploration validated this value. The excitation of the phosphors at 258 nm resulted in a vivid green luminescence. The blue region exhibited distinctive luminescence and luminous bands at 417 nm, 436 nm and 458 nm, which are attributed to the 5D3 → 7FJ (J = 5, 4, 3) transitions of terbium ions. The peaks in the green region correspond to the 5D4 → 7FJ (J = 6, 5, 4, 3) transitions of Tb3+ ions, which are caused by the f–f transitions of Tb3+ cations in the given lattice and are attributed to dipole–dipole interactions. Based on the emission spectra, the CIE chromaticity coordinates were computed and were found to fall inside the green region. As a result, the current phosphor is very beneficial for lighting applications.
Data availability
Data will be made available on request.
Author contributions
Pawan Kumar: data curation, writing – original draft, investigation, methodology; Devender Singh: writing – review & editing, resources, supervision; Sonika Kadyan: validation; Harish Kumar: software; Ramesh Kumar: visualization.
Conflicts of interest
The authors declare that they have no known competing financial interests or personal relationships that could have appeared to influence the work reported in this paper.
Acknowledgements
Pawan Kumar is thankful to UGC-New Delhi for providing SRF [117/(CSIRNETJUNE2019)]. Devender Singh is grateful to MDU, Rohtak for Post Seed Grant under research promotion scheme.
References
- R. Naik, S. C. Prashantha, H. Nagabhushana, S. C. Sharma, B. M. Nagabhushana, H. P. Nagaswarupa and H. B. Premkumar, Low temperature synthesis and photoluminescence properties of red emitting Mg2SiO4:Eu3+ nanophosphor for near UV-light emitting diodes, Sens. Actuators, B, 2014, 195, 140–149, DOI:10.1016/j.snb.2014.01.018.
- D. Singh, V. Tanwar, S. Bhagwan and I. Singh, Recent Advancements in Luminescent Materials and Their Potential Applications, Adv. Magn. Opt. Mater., 2016, 317–352, DOI:10.1002/9781119241966.ch10.
- V. Singh, S. Kaur and M. Jayasimhadri, Luminescence properties of orange emitting CaAl4O7:Sm3+ phosphor for solid state lighting applications, Solid State Sci., 2020, 101, 106049, DOI:10.1016/j.solidstatesciences.2019.106049.
- I. Gupta, P. Kumar, S. Singh, S. Bhagwan, S. K. Chhikara and D. Singh, Crystal configuration, spectroscopic and optical characteristics of Er3+ doped YAlO3 perovskites for advanced photonic appliances, Inorg. Chim. Acta, 2022, 543, 121183, DOI:10.1016/j.ica.2022.121183.
- J. Chen, H. Xiang, J. Wang, R. Wang, Y. Li, Q. Shan and H. Zeng, Perovskite white light emitting diodes: progress, challenges, and opportunities, ACS Nano, 2021, 15, 17150–17174, DOI:10.1021/acsnano.1c06849.
- D. Singh and S. Sheoran, Synthesis and luminescent characteristics of M3Y2Si3O12:Eu3+ (M = Ca, Mg, Sr and Ba) nanomaterials for display applications, J. Mater. Sci.: Mater. Electron., 2017, 27, 12707–12718, DOI:10.1007/s10854-016-5405-5.
- I. Gupta, S. Singh, S. Bhagwan and D. Singh, Rare earth (RE) doped phosphors and their emerging applications: a review, Ceram. Int., 2021, 47, 19282–19303, DOI:10.1016/j.ceramint.2021.03.308.
- D. Singh, V. Tanwar, A. P. Simantilleke, S. Bhagwan, B. Mari, P. S. Kadyan and I. Singh, Synthesis and enhanced luminescent characterization of SrAl4O7:Eu2+, RE3+ (RE = Nd, Dy) nanophosphors for light emitting applications, J. Mater. Sci.: Mater. Electron., 2016, 27, 5303–5308, DOI:10.1007/s10854-016-4428-2.
- R. Fouad and M. Saif, Synthesis, spectroscopic and photoluminescence studies of novel Eu3+ nanophosphor complex as fluorescent sensor for highly sensitive detection of latent fingerprints and anti-counterfeiting, J. Mol. Struct., 2020, 1217, 128472, DOI:10.1016/j.molstruc.2020.128472.
- P. Kumar, S. Singh, I. Gupta, K. Nehra, V. Kumar and D. Singh, Structural refinement and optical characteristics of single-phase Gd3Al5O12:Er3+ nanophosphors for luminescent applications, J. Lumin., 2022, 252, 119338, DOI:10.1016/j.jlumin.2022.119338.
- G. L. Bhagyalekshmi and D. N. Rajendran, Luminescence dynamics of Eu3+ activated and co-activated defect spinel zinc titanate nanophosphor for applications in WLEDs, J. Alloys Compd., 2021, 850, 156660, DOI:10.1016/j.jallcom.2020.156660.
- P. Kumar, D. Singh, I. Gupta, S. Singh, S. Nehra and R. Kumar, A study of phase evolution, crystallographic and down-conversion luminescent behaviour of monoclinic Y4Al2O9:Dy3+ nanophosphors for white light applications, Opt. Mater., 2023, 138, 113677, DOI:10.1016/j.optmat.2023.113677.
- P. Kumar, S. Singh, I. Gupta, V. Kumar and D. Singh, Er3+-activated LaAlO3 perovskite phosphor: crystal structure and down conversion photoluminescent behaviour for optoelectronic devices, Inorg. Chem. Commun., 2022, 1265, 109578, DOI:10.1016/j.inoche.2022.109578.
- V. Tanwar, S. Singh, I. Gupta, P. Kumar, H. Kumar, B. Mari and D. Singh, Preparation and luminescence characterization of Eu (III)-activated Forsterite for optoelectronic applications., J. Mol. Struct., 2022, 1250, 131802, DOI:10.1016/j.molstruc.2021.131802.
- P. Kumar, D. Singh, I. Gupta and H. Kumar, Influence of Dy3+ ion concentration on structural, photoluminescence and energy
transfer mechanism of promising GdSr2AlO5 nanophosphors for white light applications, Ceram. Int., 2023, 49, 29010–29024, DOI:10.1016/j.ceramint.2023.06.173.
- X. Yang, Y. Zhang, X. Zhang, J. Chen, H. Huang, D. Wang and B. Lei, Facile synthesis of the desired red phosphor Li2Ca2Mg2Si2N6: Eu2+ for high CRI white LEDs and plant growth LED device, J. Am. Ceram. Soc., 2020, 103, 1773–1781, DOI:10.1111/jace.16858.
- P. Kumar, S. Singh, I. Gupta, V. Kumar and D. Singh, Luminous LaAlO3:Dy3+ perovskite nanomaterials: synthesis, structural, and luminescence characteristics for white light-emitting diodes, Luminescence, 2022, 37, 1932–1941, DOI:10.1002/bio.4377.
- Y. Bai, Z. Jia, J. Gao, L. Wu, Y. Kong, Y. Zhang and J. Xu, A novel red-emitting phosphor K2MgGeO4: Eu3+ for WLEDs: zero-thermal quenching induced by heterovalent substitution, J. Mater., 2022, 10, 15957–15966, 10.1039/D2TC03500F.
- T. S. Dhapodkar, A. R. Kadam, N. Brahme and S. J. Dhoble, Efficient white light-emitting Mg21Ca4Na4(PO4)18:Dy3+, Tb3+, Eu3+ triple-doped glasses: a multipurpose glasses for WLEDs, solar cell efficiency enhancement, and smart windows applications, Mater. Today Chem., 2022, 24, 100938, DOI:10.1016/j.mtchem.2022.100938.
- P. Kantuptim, H. Fukushima, H. Kimura, D. Nakauchi, T. Kato, M. Koshimizu and T. Yanagida, VUV-and X-ray-induced properties of Lu2Si2O7, Y2Si2O7, and Gd2Si2O7 single crystals, Sens. Mater., 2021, 33, 2195, DOI:10.18494/SAM.2021.3316.
- X. Xu, Z. Xiao, Y. Wang, Y. Yan, J. Shen, Y. Nie and F. Lai, Structure and upconversion luminescence properties of Pr3+-doped Y2Si2O7 phosphor prepared by different methods, Opt. Mater., 2022, 134, 113191, DOI:10.1016/j.optmat.2022.113191.
- S. Liu, H. Cui, M. Liu, J. Chen, M. Wen, S. Li and X. Sun, Spherical red-emitting X1-Y2SiO5:Eu and α-Y2Si2O7:Eu phosphors with high color purity: the evolution of morphology, phase and photoluminescence upon annealing, Ceram. Int., 2022, 48, 8641–8652, DOI:10.1016/j.ceramint.2021.12.075.
- P. Kumar, S. Singh, I. Gupta, A. Dalal, V. Kumar and D. Singh, Preparation, structural and photometric properties of single-phased Gd3Al5O12:Tb3+ green-emitting phosphors for solid state lighting purpose, J. Mater. Sci. Eng. B, 2023, 288, 116189, DOI:10.1016/j.mseb.2022.116189.
- H. Tang, D. Yang, H. Li, M. Li and J. Zhu, A narrow-band RbBaBP2O8: Tb3+ green phosphor with high thermal stability for backlighting display application, J. Lumin., 2023, 257, 119733, DOI:10.1016/j.jlumin.2023.119733.
- I. Gupta, S. Singh, P. Kumar, S. Bhagwan, V. Kumar and D. Singh, Structural, morphological and optoelectronic aspects of YAlO3:Dy3+ doped nanocrystalline materials for NUV energized WLEDs, Curr. Appl. Phys., 2022, 43, 78–89, DOI:10.1016/j.cap.2022.08.011.
- P. Kumar, D. Singh and H. Kumar, Red-emitting Eu3+ activated LaSr2AlO5 phosphor for wLEDs: crystal structure, photoluminescence, thermal stability, Judd-Ofelt calculation and band-gap analyses, Mater. Res. Bull., 2024, 173, 112683, DOI:10.1016/j.materresbull.2024.112683.
- V. Kahlenberg, W. Wertl, D. M. Többens, R. Kaindl, P. Schuster and H. Schottenberger, Rietveld analysis and Raman spectroscopic investigations on α-Y2Si2O7, Z. Anorg. Allg. Chem., 2008, 634, 1166–1172, DOI:10.1002/zaac.200700548.
- J. Sokolnicki, Upconversion luminescence from Er3+ in nanocrystalline Y2Si2O7:Er3+ and Y2Si2O7: Yb3+, Er3+ phosphors, Mater. Chem. Phys., 2011, 131, 306–312, DOI:10.1016/j.matchemphys.2011.09.046.
- P. Kumar, S. Singh, I. Gupta, A. Hooda, V. Kumar and D. Singh, Reddish-orange color tunable Sm3+ activated Gd3Al5O12 phosphors: crystallographic and photophysical investigation for lighting applications, J. Mol. Struct., 2023, 1271, 134074, DOI:10.1016/j.molstruc.2022.134074.
- I. Gupta, P. Kumar, S. Singh, S. Bhagwan, S. K. Chhikara and D. Singh, Crystal configuration, spectroscopic and optical characteristics of Er3+ doped YAlO3 perovskites for advanced photonic appliances, Inorg. Chim. Acta, 2022, 543, 121183, DOI:10.1016/j.ica.2022.121183.
- N. Huang, G. Lu, B. Bai, H. Zhao, W. Yao, C. Cao and A. Xie, Preparation, crystal structure, and photoluminescence properties of Tb3+ activated Lu2(MoO4)3 green-emitting phosphors, J. Lumin., 2024, 269, 120475, DOI:10.1016/j.jlumin.2024.120475.
- F. T. L. Muniz, M. R. Miranda, C. Morilla dos Santos and J. M. Sasaki, The Scherrer equation and the dynamical theory of X-ray diffraction, Acta Crystallogr., Sect. A: Found. Adv., 2016, 72, 385–390, DOI:10.1107/S205327331600365X.
- P. Kumar, S. Singh, I. Gupta, V. Kumar and D. Singh, Structural and optical characterization of trivalent samarium-activated LaAlO3 nanocrystalline materials for solid-state lighting, J. Mol. Struct., 2022, 1265, 133362, DOI:10.1016/j.molstruc.2022.133362.
- C. Zuo, S. Yang, Z. Cao, H. Yu and X. Wei, Excellent energy storage and hardness performance of Sr0.7Bi0.2TiO3 ceramics fabricated by solution combustion-synthesized nanopowders, Chem. Eng. J., 2022, 442, 136330, DOI:10.1016/j.cej.2022.136330.
- I. Gupta, D. Singh, P. Kumar, S. Singh, S. Bhagwan and V. Kumar, Crystallographic and luminescence studies of Gd2Si2O7:Er3+ nanomaterials for NUV energized lighting applications, J. Mol. Struct., 2023, 1287, 135595, DOI:10.1016/j.molstruc.2023.135595.
- D. Navami, G. P. Darshan, D. R. Lavanya, H. B. Premkumar, S. C. Sharma, H. Adarsha and H. Nagabhushana, Design of green emitting CaZrO3:Tb3+ nanophosphor: luminescence based platform for real-time ultrasensitive detection of latent fingerprints and anti-counterfeiting applications, Opt. Mater., 2021, 122, 111474, DOI:10.1016/j.optmat.2021.111474.
- I. Gupta, D. Singh, S. Singh, P. Kumar, S. Bhagwan and V. Kumar, Phase recognition and spectroscopic characteristics of single-phase Tb3+ doped Gd4Al2O9 nanophosphors for NUV energized advanced photonic appliances, J. Lumin., 2022, 252, 119327, DOI:10.1016/j.jlumin.2022.119327.
- P. Kumar, D. Singh, I. Gupta, S. Singh, S. Nehra and R. Kumar, Combustion derived single phase Y4Al2O9:Tb3+ nanophosphor: crystal chemistry and optical analysis for solid state lighting applications, RSC Adv., 2023, 13, 7752–7765, 10.1039/D3RA00735A.
- I. Gupta, D. Singh, S. Singh, P. Kumar, S. Bhagwan, V. Kumar and S. K. Chhikara, Crystallographic, morphological and photoluminescent investigations of Tb3+-doped YAlO3 perovskites for lighting applications, Luminescence, 2023, 38, 585–599, DOI:10.1002/bio.4486.
- P. Kumar, D. Singh and I. Gupta, Physical insights into crystal structure and optical response of green light emitting Tb3+ activated GdSr2AlO5 nanophosphors for optical displays, Mater. Res. Bull., 2023, 167, 112413, DOI:10.1016/j.materresbull.2023.112413.
- U. Berwal, V. Singh and R. Sharma, Structural and optical studies on Dy3+ doped Gd2Ti2O7 pyrochlore as white light emission, Ceram. Int., 2023, 49, 8897–8906, DOI:10.1016/j.ceramint.2022.11.045.
- N. Hussain, S. Rubab and V. Kumar, Spectroscopic characterizations and investigation of Judd-Ofelt intensity parameters of Dy3+ doped Ba2La8(SiO4)6O2 near white light emitting phosphor, Ceram. Int., 2023, 49, 15341–15348, DOI:10.1016/j.ceramint.2023.01.118.
- P. Kumar, D. Singh, I. Gupta, S. Singh, S. Nehra and R. Kumar, Realization of warm reddish-orange light emitter single phase Y4Al2O9:Sm3+ nanophosphors for indoor lighting applications, J. Lumin., 2023, 257, 119703, DOI:10.1016/j.jlumin.2023.119703.
- Y. Tian, B. Chen, B. Tian, R. Hua, J. Sun, L. Cheng, H. Zhong, X. Li, J. Zhang, Y. Zheng, T. Yu, L. Huang and Q. Meng, Concentration-dependent luminescence and energy transfer of flower-like Y2(MoO4)3:Dy3+ phosphor, J. Alloys Compd., 2011, 509, 6096–6101, DOI:10.1016/j.jallcom.2011.03.034.
- I. Gupta, S. Singh, P. Kumar, S. Bhagwan, V. Tanwar, S. Nehra and D. Singh, Synthetic, structural and optical characteristic of novel color tunable reddish-orange Gd4Al2O9: Sm3+ nanocrystalline materials for solid-state photonic appliances, Inorg. Chem. Commun., 2023, 148, 110332, DOI:10.1016/j.inoche.2022.110332.
- I. Gupta, D. Singh, S. Singh, P. Kumar, S. Bhagwan and V. Kumar, Structural, optical and Judd-Ofelt analyses of Gd2-xEuxSi2O7 nanocrystals for lighting applications, Chem. Phys. Lett., 2023, 826, 140670, DOI:10.1016/j.cplett.2023.140670.
- Y. Tian, B. Chen, R. Hua, J. Sun, L. Cheng, H. Zhong, X. Li, J. Zhang, Y. Zheng, T. Yu, L. Huang and H. Yu, Optical transition, electron-phonon coupling and fluorescent quenching of La2(MoO4)3:Eu3+ phosphor, J. Appl. Phys., 2011, 109, 053511, DOI:10.1063/1.3551584.
- J. L. Cheng, H. Tang, X. Yu, Z. Wang, X. Mi and X. Zhang, Synthesis and photoluminescence properties of NUV-excited NaBi(MoO4)2: Sm3+ phosphors for white light emitting diodes, Opt. Laser Technol., 2022, 147, 107659, DOI:10.1016/j.optlastec.2021.107659.
- P. Kumar, D. Singh and I. Gupta, UV excitable GdSr2AlO5:Eu3+ red emitting nanophosphors: structure refinement, photoluminescence, Judd-Ofelt analysis and thermal stability for w-LEDs, J. Alloys Compd., 2023, 966, 171410, DOI:10.1016/j.jallcom.2023.171410.
- M. Luo, X. Sha, B. Chen, X. Zhang, H. Yu, X. Li, J. Zhang, S. Xu, Y. Cao, Y. Wang, X. Wang, Y. Zhang, D. Gao and L. Wang, Optical transition properties, internal quantum efficiencies, and temperature sensing of Er3+ doped BaGd2O4 phosphor with low maximum phonon energy, J. Am. Ceram. Soc., 2022, 105, 3353–3363, DOI:10.1111/jace.18299.
- P. Kumar, D. Singh, I. Gupta and H. Kumar, Synthesis, crystallographic structure, down shifting luminescence of Er(III) activated GdSr2AlO5 nanophosphors: an efficient green emitter for solid state lighting, Mater. Sci. Semicond. Process., 2023, 167, 107765, DOI:10.1016/j.mssp.2023.107765.
- P. Kumar, D. Singh and I. Gupta, Gadolinium-based Sm3+ activated GdSr2AlO5 nanophosphor: synthesis, crystallographic and opto-electronic analysis for warm wLEDs, RSC Adv., 2023, 13, 7703–7718, 10.1039/D3RA00636K.
- N. Ma, W. Li, B. Devakumar, Z. Zhang and X. Huang, Utilizing energy transfer strategy to produce efficient green luminescence in Ca2LuHf2Al3O12:Ce3+, Tb3+ garnet phosphors for high-quality near-UV-pumped warm-white LEDs, J. Colloid Interface Sci., 2021, 601, 365–377, DOI:10.1016/j.jcis.2021.05.108.
- S. Wang, B. Devakumar, Q. Sun, J. Liang, L. Sun and X. Huang, Highly efficient near- UV-excitable Ca2YHf2Al3O12:Ce3+,Tb3+ green-emitting garnet phosphors with potential application
in high color rendering warm-white LEDs, J. Mater. Chem. C, 2020, 8, 4408–4420, 10.1039/D0TC00130A.
- F. Liao, Y. Zhang and J. Hu, Enhancement of green emission from Ca14Al10Zn6O35:Tb3+ phosphors via cross-relaxation energy transfer by Li+ ions, J. Lumin., 2021, 231, 117791, DOI:10.1016/j.jlumin.2020.117791.
- W. W. Wu, Y. P. Zhang and J. X. Hu, Ba3YB3O9 based phosphor ceramic plates with excellent thermal stability for wLED applications, Dalton Trans., 2021, 50, 5287–5300, 10.1039/D1DT00194A.
- H. Tang, D. Yang, H. Li, M. Li and J. Zhu, A narrow-band RbBaBP2O8:Tb3+ green phosphor with high thermal stability for backlighting display application, J. Lumin., 2023, 257, 119733, DOI:10.1016/j.jlumin.2023.119733.
- K. Rawat, A. K. Vishwakarma and K. Jha, Thermally stable Ca2Ga2SiO7:Tb3+ green emitting phosphor for tricolor w-LEDs application, Mater. Res. Bull., 2020, 124, 110750, DOI:10.1016/j.materresbull.2019.110750.
- F. Chi, L. Pan, B. Jiang, Z. Ji, J. Cheng, B. Wang and S. Liu, Luminescence properties of multicolor emitting La4GeO8:Tb3+, Eu3+ phosphors, Ceram. Int., 2023, 49, 2522–2530, DOI:10.1016/j.ceramint.2022.09.230.
- Y. K. Zheng, T. S. Yang, Y. F. Xiang, K. Xiong, D. Yang, Z. Y. Fang, S. Q. Yang and J. Zhu, Ba3(ZnB5O10)PO4:Tb3+ green phosphor: microwave-assisted sintering synthesis and thermally stable photoluminescence, J. Alloys Compd., 2022, 911, 165087, DOI:10.1016/j.jallcom.2022.165087.
- J. L. Song, H. Li, Y. F. Xiang and J. Zhu, Content and temperature quenching of Tb3+- activated Bi3TeBO9 green phosphor excited by NUV/VIS light, J. Solid State Chem., 2022, 313, 123317, DOI:10.1016/j.jssc.2022.123317.
- J. F. Yang, H. Y. Wu, L. N. Song, X. X. Wang, J. C. Dong, S. C. Gan and L. C. Zou, A novel synthesis route to monodisperse Na5Lu9F32:Tb3+ phosphors with superior thermal stability, J. Lumin., 2018, 204, 533–538, DOI:10.1016/j.jlumin.2018.08.061.
- S. Limbu, L. R. Singh and G. S. Okram, The effect of lithium on structural and luminescence performance of tunable light-emitting nanophosphors for white LEDs, RSC Adv., 2020, 10, 35619–35635, 10.1039/D0RA05433J.
- P. Kumar, D. Singh and H. Kumar, Gel-combustion synthesis, structural and optoelectronic analyses of Tb3+ activated LaSr2AlO5 nanophosphors: a green emitter for displays, Mater. Sci. Semicond. Process., 2024, 174, 108162, DOI:10.1016/j.mssp.2024.108162.
- I. Gupta, D. Singh, S. Singh, P. Kumar, S. Bhagwan and V. Kumar, Structural and photophysical measurements of Er3+ doped Gd4Al2O9 nanophosphors for NUV excitable solid-state lighting applications, Chem. Phys. Lett., 2023, 814, 140350, DOI:10.1016/j.cplett.2023.140350.
- P. Kumar, S. Singh, I. Gupta, K. Nehra, V. Kumar and D. Singh, Structural and luminescent behaviour of Dy(III) activated Gd3Al5O12 nanophosphors for white-LEDs applications, Mater. Chem. Phys., 2023, 295, 127035, DOI:10.1016/j.matchemphys.2022.127035.
- B. Deng, Y. Yang, W. Chen, X. Xie, R. Yang, C. Li and R. Yu, Synthesis and characterization of thermostable Dy3+-doped La5NbMo2O16 yellow-emitting phosphors for w-LEDs, J. Mater. Sci.: Mater. Electron., 2022, 33, 23042–23053, DOI:10.1007/s10854-022-09071-2.
|
This journal is © The Royal Society of Chemistry 2024 |
Click here to see how this site uses Cookies. View our privacy policy here.