DOI:
10.1039/D4RA02939A
(Review Article)
RSC Adv., 2024,
14, 17946-17988
Advancements in enzyme immobilization on magnetic nanomaterials: toward sustainable industrial applications
Received
20th April 2024
, Accepted 27th May 2024
First published on 5th June 2024
Abstract
Enzymes are widely used in biofuels, food, and pharmaceuticals. The immobilization of enzymes on solid supports, particularly magnetic nanomaterials, enhances their stability and catalytic activity. Magnetic nanomaterials are chosen for their versatility, large surface area, and superparamagnetic properties, which allow for easy separation and reuse in industrial processes. Researchers focus on the synthesis of appropriate nanomaterials tailored for specific purposes. Immobilization protocols are predefined and adapted to both enzymes and support requirements for optimal efficiency. This review provides a detailed exploration of the application of magnetic nanomaterials in enzyme immobilization protocols. It covers methods, challenges, advantages, and future perspectives, starting with general aspects of magnetic nanomaterials, their synthesis, and applications as matrices for solid enzyme stabilization. The discussion then delves into existing enzymatic immobilization methods on magnetic nanomaterials, highlighting advantages, challenges, and potential applications. Further sections explore the industrial use of various enzymes immobilized on these materials, the development of enzyme-based bioreactors, and prospects for these biocatalysts. In summary, this review provides a concise comparison of the use of magnetic nanomaterials for enzyme stabilization, highlighting potential industrial applications and contributing to manufacturing optimization.
1. Introduction
Magnetic materials are widely used in modern technologies and devices with a broad classification of chemical and physical properties directly dependent on their structural composition.1–4 Specifically, materials with constituents such as iron, nickel, aluminium, cobalt, and others that favour a response to a magnetic field are characterized as magnetic.5–11 These materials can be classified as soft and complex, and magnets can attract both; however, soft materials are attracted only temporarily, while hard materials can be magnetized indefinitely.11–17 Because of their versatility, they can be applied in various fields: sensing, smart devices, storage, biomedicine, immobilization and enzymatic stabilization, and adsorption of effluents and wastewaters.16–29 These materials can be used at different scales: macro metric, micrometric, and nanometric.11,16,30–34
Magnetic nanomaterials have attracted significant interest from various industries that synergistically apply nanoscience and nanotechnology to solve ongoing challenges.11,16,35–37 The versatility of magnetic nanomaterial-based compounds is attributed to unique properties (e.g., superparamagnetism), which result from the influence of thermal energy on a ferromagnetic nanoparticle.11,17,26 When used as reinforcement materials, these compounds can enhance existing physical or chemical properties.24–28,36,38 The synthesis of these nanomaterials is constantly evolving, and several routes have been proposed over the years.39–42 The chemical or physical route used to produce this compound is defined based on its proposal of the final application. Several methods can be used, such as co-precipitation43–45 aerosol route,46–48 hydrothermal reaction49–51 oxidative precipitation,52,53 organic precursor method54,55 sonochemical decomposition,56–59 and sol–gel synthesis technique.60–62
Immobilization and enzymatic stabilization are among the most favourable application areas for these nanomaterials because several factors favour catalytic activity and stabilization in various reactive environments unsuitable for the use of soluble enzymes.63–66 Advantages include high surface area, large surface-to-volume ratio and separation facilitated under external magnetic fields.67–70 It should be noted that magnetite nanoparticles (Fe3O4) are used more frequently compared to other types.71–73 The low toxicity, good compatibility and high surface area justify the frequent use of this enzyme immobilization matrix.72,74 One of the challenges to the efficient use of these materials in enzyme immobilization protocols is their high reactivity and easy degradation when exposed to specific environments, causing instability and better dispersion of the enzyme.66,72,75,76
However, several methods for modifying magnetic iron nanoparticles have been developed to improve the carriers for unrestricted use and with maximum efficiency.66,70,72,74,77–81 Polymeric molecules such as polyethene glycol (PEG)82,83 polyvinylpyrrolione (PVP),84–86 poly (lactic-co-glycolic acid) (PLGA)87–89 and polyvinyl alcohol (PVA)87–89 have been used as coatings for these nanoparticles, mainly enhancing various chemical and physical properties75,90–93 In addition, the surface coating is made of natural and often abundant organic molecules such as chitosan, chitin, ethyl cellulose, gelatin, starch, (3-aminopropyl) trietoxylesan (APTES), carboxymethyldextrana among others94–103 has been used. Therefore, chemical modifiers increase the versatility of these supports, allowing the immobilization of various biologically active and complex molecules.11,104–106
The enzyme immobilisation methods in magnetic nanoparticles are diverse, and their use depends on the final application of the biocatalyst.74,107,108 Immobilization by physical adsorption is one of the most common methods and one of the first developed.66,69,72,74,109 The interactions between matrix and enzyme are weak, such as electrostatic interactions, hydrogen bonds, van der Waals forces, and hydrophobic interactions.69,72 The reaction conditions directly affect these interactions, which include pH, temperature, ionic strength, and biomolecule concentration.66,70,72,75,110,111 The robustness of the support properties in these protocols is fundamental to the efficient use of these biocatalysts.66,75,112,113 The protocol of immobilizing enzymes by covalent coupling is one of the most widely used due to increased enzymatic stability, which improves enzymatic activity.112–118 In addition, other immobilization methods that use specific biologically mediated interactions are also used, such as ionic binding, trapping, and enzymatic encapsulation.119–127
For years, several reaction processes have experienced immobilisation and enzymatic stabilisation, improving protocols for synthesising and immobilising magnetic nanoparticle supports. Several enzymes have been immobilized in magnetic matrices belonging to the groups: xirreductases,128,129 transferases,130,131 hydrolases,132–134 lyases,135–137 isomerases,138 lipases,139–143 among others. The non-toxicity of magnetic nanoparticles and their surface area allow most of the above enzymes to interact efficiently, favouring immobilization parameters such as increased catalytic activity, better operational, thermal and pH stability, increased immobilization yield and more significant.144–150
Currently, enzymatic immobilization protocols are increasingly being analyzed to optimize processes, reduce costs, and improve immobilization parameters.151–154 This experimental design is a powerful tool to overcome the challenges of industrial scalability.155,156 Variable analysis using experimental design is a promising alternative that has been increasingly used.157,158 Numerous studies have been published discussing the main factors influencing enzymatic immobilization protocols.154,157,159,160 Golmohammad Khoobbakht et al. (2020) show that Burkholderia cepaciaa lipase was stabilized in magnetic nanoparticles of mesoporous silica shell–shell cores to synthesize biodiesel from residual soybean oil. Statistical optimization methods such as response surface methodology (RSM) with central composite design (CCD) were used. Notably, it predicted the biodiesel yield to be 92% under ideal conditions.161 In this sense, there is a latent need for the use of statistical tools to optimize enzyme immobilization processes.154,159,162
In summary, immobilization protocols, the development of new supports, and process optimization are based on much of the current research on enzyme immobilization on solid media.163,164 Deciding on the most appropriate immobilization protocol is fundamental to achieving maximum biocatalyst efficiency.165–167 The development of hybrid supports, focusing on those of organic–inorganic composition with magnetic properties, taking advantage of organic residues, offers a promising option for the enzymatic immobilization process with the sustainable prerogative in its synthesis.168–172 Experimental design, molecular simulation of biobehavioral, and analysis of variance are essential pillars for process optimization, focusing on the immobilization of active biomolecules, seeking maximum efficiency of support synthesis protocols, immobilization, and final application of the biocatalyst.154,159,162,166,167,173 Therefore, the following study will address the most critical and current aspects of magnetic matrices for enzymatic immobilization. It will be based on the challenges and opportunities of this field, from the synthesis of magnetic nanomaterials to the immobilization protocols to the finalization of the essential aspects of process optimization.
2. Magnetic nanomaterials
2.1 Magnetic properties
The magnetic property of a material is determined by the magnetic moments per unit volume within the material.174 Magnetic nanoparticles (MNPs) have inherent magnetic properties that make them versatile for various applications.175–178 The magnetic properties of a material are classified based on its magnetic susceptibility (χm),179 a fundamental response that indicates how a system interacts with an external magnetic field.
This parameter relates the magnetization of a material to the strength of an applied magnetic field.180 The five basic types of magnetism are diamagnetism, paramagnetism, ferromagnetism, antiferromagnetism, and ferrimagnetism.179
MNPs exhibit unique properties, including high saturation magnetization/sizeable magnetic moment, response to moderate magnetic fields, and superparamagnetism.181 These properties make them ideal for magnetic separation by application of a magnetic field.182,183 Superparamagnetism, which results from the influence of thermal energy on ferromagnetic nanoparticles, has attracted interest in recent decades.184 High saturation magnetization (Ms) and superparamagnetism are essential for applying an external magnetic field.185
MNPs address the challenges of handling and separating immobilized enzymes with low density and high dispersion, enhancing their reusability.176,186,187 This allows for extended use in continuous mode while protecting against thermal and chemical changes during manufacturing and storage.187 Magnetic recovery reduces production costs, and immobilized enzymes often exhibit higher activity and improved temperature and pH stability than unsupported enzymes.179
The excellent superparamagnetic property of MNPs allows easy separation from the reaction medium by simply applying an external magnetic field to the immobilized enzyme, followed by easy dispersion after the field is removed.183,188,189 Unlike ferromagnetic nanoparticles, superparamagnetic nanoparticles do not retain their magnetic properties once the external magnetic field is removed, which is a significant advantage for reusing nanobiocatalysts.190 Fig. 1 illustrates the importance of superparamagnetism in enzyme immobilization.
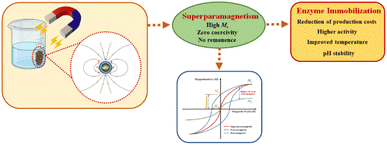 |
| Fig. 1 The superparamagnetism of MNPs in enzyme immobilization. | |
The magnetic properties of nanomaterials play a crucial role in the recovery process using an external magnetic field.178 Higher Ms indicates superparamagnetic activity,191 which is characterized by low coercivity (Hci) and retentivity (Mr).192 Superparamagnetism and high Ms allow reactor operation at relatively high flow rates and effective biocatalyst recovery.185 However, magnetic properties can be reduced after modification and enzyme immobilization due to the presence of non-magnetic nanomaterials.193,194 MNPs must be modified with functional groups to increase the enzyme binding tendency further and thus achieve efficient enzyme immobilization.195 Enzyme immobilization on MNPs can reduce Ms if a biopolymer-based coating imparts a diamagnetic quality.188 Coating MNPs improves multifunctionality and biocompatibility,196 with both aggregation197,198 and crystalline anisotropy197 influencing Ms.
Among the supports, magnetite (Fe3O4) stands out as the most widely used MNP for enzyme immobilization189 because of its cost-effectiveness, biocompatibility, low toxicity, large surface area because of small particle size, high magnetic susceptibility, high saturation magnetization, and superparamagnetic properties at room temperature.189,194,199,200 Consequently, immobilization of enzymes on MNPs significantly improves stability, catalytic performance, and reusability compared to pure enzymes.189,200,201
2.2. Superparamagnetism
Among the properties of nanomaterials, superparamagnetism occurs when the magnetic material is reduced in size, such as between 10 nm and 150 nm in diameter202,203 and presents a single-state domain in which the magnetic spins are aligned202 (Fig. 2). In nanomagnetic particles with a single domain and smaller diameters, the superparamagnetic nanomaterial has less hysteresis, and demagnetization occurs more readily, with variations in hysteresis and magnetization becoming zero after a critical size radius is reached204–206 (see Fig. 1). Overall, the practical advantages of superparamagnetic nanoparticles, such as biocompatibility, low toxicity, easy separation, and flexibility in modifying their surface, are relevant to their widespread use, especially in medicine.207,208
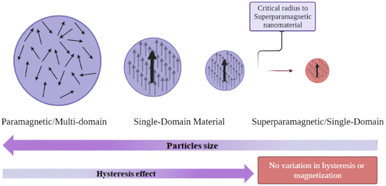 |
| Fig. 2 Changes in the physicochemical properties of nanomaterials as a function of particle size. When particles are sized at the critical radius for superparamagnetic nanomaterials, the hysteresis and magnetization of the particle become zero. | |
Several biomedical applications using superparamagnetic particles as drug-delivery systems can be found in the literature. In Neuberger et al. (2005), iron oxide-based superparamagnetic nanoparticles (SPIONs) are used as contrast agents in magnetic resonance imaging for the diagnosis of cartilage pathologies.203 In addition, SPION can also be used as an oral contrast agent to diagnose gastrointestinal tumours or as an intravenous agent to detect other tumours in the body.207,209 To use these particles as a drug-delivery system, the magnetic field is removed shortly after the particles are combined with an external magnetic field, which coordinates the delivery to the desired target area, making drug delivery more effective and less time-consuming.203,210
Using nanoparticles as a drug-delivery system is also advantageous for treating skin diseases. In Raviraj et al. (2021), SPIONs were developed to facilitate drug distribution in chemotherapy treatments of myeloma in rats in a non-invasive methodology, without needles and without controlling the local application of drugs.208,211 The authors observed that the application of SPIONs with steric stabilization showed excellent penetration into the skin and promising results in treating skin tumours, with an increase in the immune response of the system associated with leukocyte infiltration in the studied tissue.208,212 The results also suggest that using these nanomaterials as a drug-delivery system may contribute to the resumption of studies with drugs discontinued in chemotherapy regimens due to their high toxicity.208,212
2.3 Magnetic nanoparticle preparation
Magnetic nanoparticles (MNPs) consist of a magnetic material and a chemical component, and the functionality and application of MNPs are directly influenced by these two components.213 The core of MNPs is primarily synthesized from Fe3O4 and typically comprises iron oxides such as Fe3O4, hematite (α-Fe2O3), maghemite (γ-Fe2O3), and FeO (iron(II) oxide).214 The development and implementation of efficient techniques for the synthesis of high-quality nanomaterials is crucial.215,216 The synthesis method and experimental conditions strongly influence the size and morphology of magnetic particles, which determine the material's magnetic properties and its applications.215–218 Size and size distribution play a crucial role in determining the chemical and physical properties of MNPs, which further influence their functionality.215,216,218 Because of their small particle size, MNPs often exhibit superparamagnetism,196,218,219 characterized by dimensions approaching those of a single magnetic domain.196 Tailoring of magnetic properties can be achieved by controlling the magnetic moment and crystallite size by incorporating high-entropy oxides.220
Precise control of the nanoparticle production parameters is essential, as the unique properties of MNPs are highly dependent on their size and morphology.221 The synthesis method is selected based on the desired length, stability, morphology, and biocompatibility of the MNPs.218 The primary synthesis methods for MNPs include physical, wet chemical, and a few biological approaches (e.g., green synthesis or biosynthesis).218,222,223 Physical methods involve fractionating bulk material into smaller pieces through high-energy processes such as ball milling, considered a “top-down” approach.215,224 In contrast, the “bottom-up” approach includes chemical and biological methods that involve particle formation through nucleation, growth, and precipitation.215,224 Biological and chemical synthesis methods are the most widely used.218 Physical methods, while providing better control over size and shape, have limitations, such as dispersed particle size distribution, time-consuming processes, and higher costs.215 Standard methods for MNP synthesis include ball milling, coprecipitation, sol–gel, hydrothermal, thermal decomposition, microemulsion, and biological approaches,223 as shown in Fig. 3.
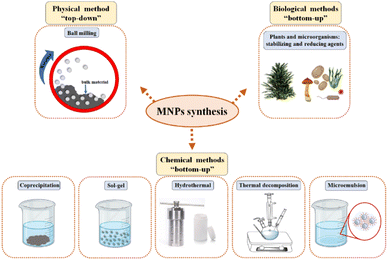 |
| Fig. 3 Standard methods for MNP synthesis. | |
Coprecipitation, a chemical synthesis method, uses a precursor salt and an essential precipitant,219,222 often employing ferric (FeCl3) and ferrous (FeCl2) chlorides with ammonium hydroxide (NH4OH).222 This method is preferred to achieve monodispersity of iron oxide MNPs and is considered the simplest for Fe3O4 synthesis.218,219 The oxidation states play a crucial role in controlling the dispersion behaviour of iron oxide MNPs, with the size and state of superparamagnetic iron oxide MNPs being modifiable by adjusting factors such as salt type, iron(II)/iron(III) molar ratio, ionic strength, temperature, and pH.218 While coprecipitation is easy to perform, it tends to result in poorer crystalline quality and magnetic behaviour due to accumulation, although stability in aqueous media is maintained.216,222 This synthesis process is suitable for applications requiring large nanocrystals, where homogeneity in size and magnetic properties is not critical.223 Compared to physical or vapour phase methods, coprecipitation provides better control over size and shape.222
Sol–gel synthesis involves gel formation at room temperature by polycondensation reactions of metal alkoxides and hydrolysis and does not require special equipment.223 The sol or colloidal solution is prepared from metal salts dissolved in water, and the gel is obtained after drying the solvent by heating and roasting.223,225 While size, shape, and composition can be controlled, the sol–gel method requires many toxic organic solvents and has some disadvantages, such as binding and high permeability.225
Hydrothermal synthesis involves using an autoclave with Teflon-lined stainless steel walls, typically containing water in a supercritical/sub-supercritical state.222,223,226 This method operates under high temperature and pressure conditions, resulting in oxidation and hydrolysis reactions that produce MNPs of uniform size. Synthesis parameters affect crystallinity, crystallite size, particle size, purity, and magnetic properties.226 Hydrothermal synthesis is preferred to produce highly crystalline MNPs with the desired size and shape.218 It allows the development of different crystalline iron oxide nanoparticles, ensuring high crystallinity, size, shape and homogeneous composition.222
Thermal decomposition is an upscaled and extended synthesis process using organic solvents and nonmagnetic organometallic precursors.222 It produces MNPs under extreme temperatures by decomposing organometallic precursors with organic surfactants.215,223 The products' morphology, size, and monodispersity can be tuned by modifying the experimental conditions, and the annealing temperature affects the size and magnetic properties of the MNPs.219,227 The obtained hydrophobic MNPs have limited applications (e.g., biomedical applications).225 The hydrophobic MNPs obtained have limited applications, and thermal decomposition is energy-, material-, and time-consuming, using expensive and hazardous substances.215
In the microemulsion process, a mixture of oil, surfactant, and water is magnetically stirred at ambient temperature, with surfactants and co-surfactants stabilizing the interphase.223,227,228 The mixed system undergoes nucleation, crystal growth, aggregation and agglomeration, followed by precipitation of spherical microdroplets in a solid phase. The desired material is obtained after adding organic solvent and centrifugation.225 While the method offers precise control over shape and particle size, high purity, good crystallinity, narrow size distribution, and the synthesis of various MNPs, it has drawbacks such as low-volume production, time consumption, and specialized equipment.229
In recent years, biosynthesis has gained popularity as an environmentally friendly and cost-effective approach compared to chemical or physical routes.230,231 In this process, plants and microorganisms stabilize and reduce gents.223,230,232 The produced MNPs are biocompatible and suitable for biomedical applications, and biosynthesis does not require expensive or hazardous chemicals, providing a simple and rapid processing route.223,231 However, biosynthesis may result in poor dispersion of nanoparticles, and the related shortcomings, such as yield and MNP dispersion, still need to be investigated.223
2.4 Structural characterizations
The techniques used to synthesize and characterize magnetic nanoparticles (MNPs) are vital in understanding their properties. Characterization is a critical preliminary step, especially given the diverse synthesis routes and applications of MNPs.175,183,184,223,233,234 Structural characterizations are essential to verify the effects on MNPs after synthesis and modifications, and different techniques are used in MNPs research.175,181 Fig. 4 summarizes the significant approaches to the structural characterization of MNPs.
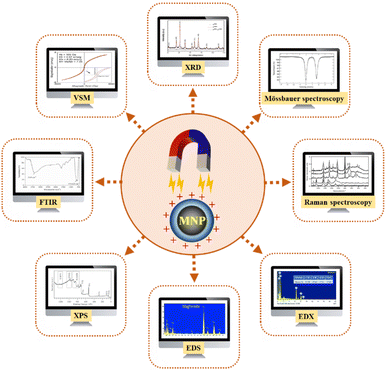 |
| Fig. 4 The main structural characterization approaches of MNPs. | |
Powder X-ray diffraction (XRD) is critical in determining nanomaterials' crystal structure and crystalline nature.233,234 It provides insight into the crystallinity, diameter, and structural changes introduced by coatings, functionalization, and immobilized materials on MNPs.196,233,235,236 However, XRD alone may not distinguish iron oxide nanoparticles such as γ-Fe2O3 and Fe3O4 because of their similar patterns originating from identical cubic spinel structures. Mössbauer spectroscopy, a precise technique, is used to study the local structure of Fe and provides detailed information about the composition.192,237 Mössbauer spectroscopy, a precise technique, is used to investigate the local structure of Fe, providing detailed information about the composition.238 While giving a screening approach, Raman spectroscopy does not determine the exact amount of Fe3O4, a capability that Mössbauer spectroscopy has.239
Energy dispersive X-ray (EDX) analysis and energy dispersive spectroscopy (EDS) are used to identify the chemical composition of synthesized MNPs.178,230,240 These techniques are critical to confirm the material's successful modification and evaluate the impact of surface modifications,178,181,230,234 such as enzyme immobilization on MNPs.235,236,241 X-ray photoelectron spectroscopy (XPS) confirms each magnetic nanoparticle surface modification step and successful enzyme binding.183,194 XPS is a powerful method to verify the existence of the Fe3O4 phase due to the coexistence of Fe2+ and Fe3+ cations.237 Fourier-transform infrared spectroscopy (FTIR) is used to evaluate functional groups and their possible interactions, providing insight into the formation of MNPs and surface modifications.189,194,214,235,241
Vibrating sample magnetometer (VSM) measurements assess nanostructured magnetic materials' magnetic behaviour and magnetic moment when subjected to vibrations perpendicular to a uniform magnetic field.233,242 VSM reveals changes in magnetization that may indicate the presence of a non-magnetic layer at the core of the material.196 The magnetization curve obtained from VSM can provide information about the behaviour of immobilized enzymes on MNPs and verify the superparamagnetic properties of the composite material.188,214 Successful immobilization of enzymes on MNPs is often confirmed by studying their saturation magnetization Ms.241
Magnetization curves indicate superparamagnetism without hysteresis, visible coercivity and remanence, and a fully reversible magnetization process.194,196 The analysis of superparamagnetism can be further refined by calculating the material's diamagnetic properties by comparing the sample's mass and the corresponding magnetic properties.233
3. Enzyme immobilization onto magnetic nanoparticles
3.1 Enzymes and enzymes immobilization techniques
Enzymes are essential biocatalysts involved in biosynthesis and biodegradation that enable a wide range of human activities,243 providing energy for most of the metabolic processes of the cell and assisting in a variety of biochemical reactions that generally occur under favourable conditions in the physiological environment.243–245
In addition, they have high chemo-, regio-, and stereoselectivity, resulting in more pure and selective reactions that can even reduce the need for functional group protection, reduce purification steps, and increase the atom economy of the process, resulting in shorter synthetic routes.246,247
Become an essential pillar of the bio-economy, indispensable for the sustainable development of various scientific and technological sectors, industry, medicine, and society. Playing a notable role in several segments (such as energy production processes, biofuels, pharmacists, biosensors, the food industry, and textiles).248,249
However, enzymes' applications and desirable properties are constantly hampered by their instability at elevated temperatures or in aggressive solvents. Their inability to be recovered and reused makes their widespread use challenging. Immobilization can overcome these disadvantages, which enhances photocatalysts, making them more robust and resistant to thermal and solvent stress and preserving their catalytic activity under extreme conditions.248,249
Thus, immobilization improves the stability, selectivity, and kinetics of the enzyme, the main goal of which is to strengthen the biocatalyst's physical and enzymatic stability. Several methods are used to immobilize the enzymes. However, the industry always chooses the most accessible and economical ones based on physical or chemical immobilization, such as adsorption, covalent bonding, crosslinking, and encapsulation.250 Fig. 5 shows the enzymatic immobilization techniques according to their classification and approaches.251
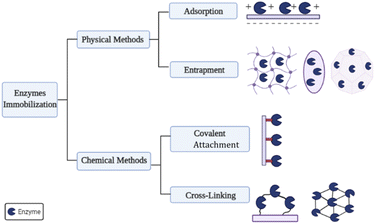 |
| Fig. 5 Methods for enzymatic immobilization. | |
3.1.1 Adsorption. The adsorption process is simple, inexpensive, reagent-free, and generally does not cause chemical changes in the enzyme as it does not involve functionalization of the support.252,253 It occurs through physical forces of attraction, and enzymes are immobilized on supports via van der Waals bonds, hydrophobic interactions, hydrogen bonds, and ionic bonds.254,255
3.1.2 Covalent attachment. Enzymes can be covalently immobilized on supports through chemical interactions, which provides high stability and enzymatic adherence to the support matrix, resulting in low leakage of the supported enzyme and attesting to the rigidity of its structure, which in turn can be naturally preserved against destructive agents such as heat, organic solvents, extreme pH, and others.256The covalent immobilization method usually involves two steps. First, the support surface is activated by bifunctional agents such as glutaraldehyde,257 and then the enzyme is immobilized on the covalently activated surface. The crosslinkers, generally used in covalent binding, link the support material and the enzyme molecules.256,257
3.1.3 Crosslinking. In this method, enzymes are crosslinked to the support matrices by bifunctional reagents, of which glutaraldehyde is usually one of the most commonly used. Based on intermolecular reactions, the enzymes are thus immobilized with solidity through covalent bonds to improve reusability and stability. However, the catalytic activities of the enzymes may disappear during crosslinking.258,259Essential cross-linking techniques are obtained by crystallizing, atomizing, and aggregating enzymes; at the end of cross-linking, the enzyme is immobilized, resulting in the production of cross-linked enzyme (CLE), cross-linked enzyme crystals (CLEC), bound enzyme aggregates (CLEA), and cross-linked spray drying enzyme (CSDE).258,259
3.1.4 Encapsulation. In immobilization by encapsulation, enzymes are held in polymeric structures with pores that allow substrates and products to pass through. Unlike adsorption, encapsulation protects the enzyme from direct contact with the reaction medium, minimizing inactivation effects because of the nature of the solvent in the medium. In addition, the method allows the enzymes to remain stable for a relatively long time and does not require extraction of the enzymes from the medium.258,259
3.2 Magnetic nanoparticles as supports
Good support material and its interaction with the enzyme are essential in immobilization, as the support properties can alter biocatalyst activity and enzyme loading.260
Thus, several nanostructured materials represent a relevant and new class of support matrices that have been investigated for the immobilization of various enzymes,261 such as nanoparticles,262 nanofibers,263 nanotubes,264 and nanosheets.265
Thus, they have promising applications in the biotechnology industry, as the catalytic activity of nanomaterials is similar to that of enzymes266 because of their low cost, flexible catalytic activity, and high operational stability.267
In addition, the magnetization of substrates before use has shown great potential for recyclable applications.268 Since nanomaterials can be easily collected and recycled by an appropriate external magnetic field through the incorporation of magnetic nanoparticles,268,269 magnetite (Fe3O4) is considered to be favourable as a nanocarrier for immobilization of enzymes due to its large surface area.268,269
Recently, several published works have developed such support for the presence of magnetic compounds to immobilize enzymes. The relevance of Fig. 6 can be seen in a Scopus search using the keywords nanoparticles and magnetic supports.
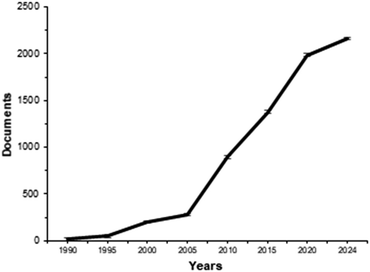 |
| Fig. 6 Trends in the number of articles retrieved from Scopus using keywords such as “nanoparticles” and “magnetic supports”. | |
Yang et al. (2016) synthesized in situ rGO-Fe3O4 nanocomposites to support the immobilization of catalase enzyme (CAT) up to 312.5 ± 12.6 mg g−1, with almost no enzymatic leaching and the recovery of CAT activity can be increased to about 98% due to the high surface area of graphene and a magnetic field effect of Fe3O4 nanoparticles. Studies direct nanocomposites as versatile nanosupports for biological or chemical reactions and separations.270
In this study, Lin et al. (2017) synthesized Fe3O4 nanoparticles and coated them with chitosan, and glutaraldehyde was used as a cross-linking reagent for cellulase immobilization. The tests showed that the immobilized particles exhibited optimum cellulase loading efficiency (LE) of 99.6% and standard recovery rate (RR) of 68.5%, with a broader range of adaptability to pH and hydrolysis temperature compared with free cellulase, in addition to hydrolyzing efficiently for five experiments, maintaining an average of 80% of free cellulase activity. They were suggested to have promising potential in applying cellulose hydrolysis.271
Mehnati-Najafabadi et al. (2018) immobilized the xylanase in graphene oxide (GOMNP) superparamagnetic nanofilms functionalized with polyethene glycol bisamine (PEGA). The results showed that the xylanase was bound to the functionalized nanocomposite, yielding 273 mg of enzyme per gram of PEGA-GOMNP. The immobilized enzyme retained approximately 40% of the initial activity after eight cycles and 35% of the initial catalytic activity after 90 days of storage at 4 °C. The study indicated that the support is biodegradable and suitable for bioengineering.272
Xue et al. (2019) immobilized lysozyme in a 1,2,3,4-butanotetracarboxylic acid-modified cellulose magnetic microsphere (BTCA), which exhibited better properties such as resistance to temperature, pH, and thermal and storage stability compared to free lysozyme. The apparent kinetics of immobilized lysozyme showed that its Km value was 1.37 times higher than that of free lysozyme, and its Vmax was slightly lower, with an acceptable reuse of 51.9 ± 2.2% of activity after six cycles.273
Bezerra et al. (2020) immobilized Thermomyces lanuginosus (TLL) on a new hetero-functional divinyl sulfone (DVS) support in superparamagnetic nanoparticles functionalized with polyethyleneimine (SPMN@PEI-DVS), the remaining DVS groups were blocked with ethylenediamine (EDA), ethanolamine (ETA) and glycine (GLY) to prevent uncontrolled enzyme support reactions. As a result, 100% immobilization yield was achieved in 1 hour at pH 10. However, at pH 5.0, they obtained the most excellent stability during thermal inactivation and good enantioselectivity for the hydrolysis of racemic methyl mandelate, the nanocatalysts blocked with EDA and ETA being 68% and 72%, respectively. They showed that the biocatalyst has excellent potential for industrial applications.274
Coutinho et al. (2020) used the co-precipitation method to synthesize hydroxyapatite (HA)/cobalt ferrite (CoFe2O4) composites with different mass ratios to evaluate the viability support for the immobilization of β-glucosidase, phytase, and xylanase enzymes. The results showed that the composite with the highest cobalt ferrite content (2
:
1 ratio) was highly effective for immobilizing the three different enzymes, with immobilization yields (IYs) between 70 and 100% and recovered activities of 78 to 100%. Biocatalysts could be recovered rapidly, especially β-glycosidase, which could be reused 10 times while retaining about 70% of its initial activity.275
Carvalho et al. (2020) used magnetic nanoparticles (Fe3O4) for the physical adsorption of Yarrowia lipolytica MUFRJ50682 lipase, achieving a high immobilization efficiency of 99%, and this biocatalyst was recycled 30 times with 70% lipase activity at the end. Moreover, they showed that immobilization on magnetic nanoparticles could achieve high pH tolerance and thermostability with a 40% improvement in thermodynamic parameters at 60 °C.276
Coşkun et al. (2021) aimed to increase the enzymatic activity and enantioselectivity of the lipase Candida antarctica B (Cal-B) by immobilization on graphene oxide (GO) nanoparticles, iron oxide (Fe3O4) and graphene oxide/iron oxide nanocomposites (GO/Fe3O4). The prepared samples were used as biocatalysts in the enantioselective transesterification reaction of (R,S)-1-phenylethanol reported for the first time in the literature.277
Perveen et al. (2021) fabricated a bioanode using nanocomposites containing magnetic particles of iron oxide (Fe3O4), carbon nanotubes (CNT), gold nanoparticles (Au), and a conductive polypyrrole polymer (PPy), which was used as a support electrode for the immobilization of glucose oxidase (GOD) and investigated for its application in an enzymatic biofuel cell (EBFC) of glucose to improve the electron transfer kinetics and electrode stability. The bioanode was considered as a prospective material for the development of better electrochemical biosensors and biofuel anodes and showed promising results, such as the maximum current density of 6.01 mA cm−2 (0.22 V vs. Ag/AgCl) in 40 mM glucose concentration at 0.38 V open circuit potential (OCV).278
Paz-Cedeno et al. (2021) synthesized magnetic graphene oxide (GO-MNP). It was used as immobilization support for an industrial preparation containing cellulase and xylanase, which showed high activity for hydrolysis of pretreated sugarcane bagasse (PSB) and related activities of endoglucanase, xylanase, β-glucosidase and β-xilosidase of 70%, 66%, 88% and 70%, respectively, after 10 cycles, also maintained about 50% and 80% of their efficiency for cellulose and xylan hydrolysis. Thus, the study indicated the biocatalyst as a potential candidate for industrial applications such as second-generation ethanol production.279
Thus, immobilising enzymes on magnetic supports has demonstrated efficacy and promise across chemical, biochemical, and industrial reactions. Studies have documented improvements in thermal stability and pH, facilitating fast and easy recovery for the reuse of biocatalysts at acceptable rates and over multiple cycles for diverse catalytic systems. In this sense, Table 1 presents the different enzyme carriers and their applications.
Table 1 Studies described in the literature on the different supports of magnetic nanoparticlesa
Enzyme |
Magnetic carrier |
Characteristics of NP |
Coupling agent |
Synthesis method |
Recovered activity |
Cycles |
Application |
References |
Note: (GLU) – glutaraldehyde; (AP) – 3-aminopropyl triethylenesilane; (EDC)- 1-etil-3-(3-dimetilaminopropil) carbodiimida; (APTES) – 3-aminopropyltriethoxysilane; (GO) – graphene oxide; (NHS) – N-hydroxysulfosuccinimide; (BTCA) – 1,2,3,4-butanetetracarboxylic; (DVS) – divinyl sulfone; (EPH) – epichlorohydrin; (GA) – gallic acid; (HA) – hydroxyapatite; glycidol – (GLY); chitosan-coated magnetic nanoparticles – (CMNP). |
Aspergillus niger |
CMNP |
Showed area amorphous and a thin chitosan layer |
GLY and GLU |
Covalent |
— |
15 |
Biotechnological processes involving magnetic separation |
280 |
C. rugosa |
Fe3O4-MCM-41 |
Spherical morphology |
GLU |
Covalent |
— |
4 |
Interesterification of soybean oil and lard |
281 |
Trypsin (EC 3.4.21.4) |
Fe3O4@GA |
17 nm |
— |
Adsorption |
— |
8 |
Use in various protein degradation processes in the chemical and food industries |
282 |
Lacase (EC 1.10.3.2) |
Fe3O4@silica |
— |
GLU |
Covalent |
36.3 U L−1 |
6 |
— |
283 |
Swine pancreas lipase (PPL) |
Fe3O4@GO |
Between 10 and 25 nm |
APTES |
Covalent |
— |
6 |
Hydrolysis of p-nitrophenyl dodecanoate |
284 |
Pseudomonas fluorescens |
Fe3O4@APTES |
15 nm |
GLU |
Covalent |
100% |
5 |
Kinetic resolution of rac-secondary alcohols with potential application in heterogeneous catalysis |
285 |
C. rugosa |
Fe3O4@GO |
— |
EDC and NHS |
Covalent |
64.9% |
5 |
Biodiesel production |
286 |
Lysozyme |
Fe3O4@cellulose |
Mean size range was 400–500 nm |
BTCA |
Covalent |
78.8% |
6 |
Improve stability and bio-catalytic activities |
287 |
Thermomyces lanuginosus |
Fe3O4@PEI-DVS |
— |
DVS |
Multipoint covalent attachment |
— |
7 |
Pharmaceutical, cosmetic, biotechnology and fine chemical industries |
288 |
β-Glucosidase, fitase e xilanase |
HA-CoFe2O4 |
— |
— |
Adsorption |
Range 75–100% |
Up to 10 hydrolysis cycles to β-glucosidase |
Biofuels, pharmaceutical drugs, paper and pulp, animal feed, food, beverage, among others |
289 |
Trichoderma asperellum laccase |
Fe3O4@SiO2-chitosan |
From 350 to 700 nm |
— |
— |
— |
8 |
Enhanced biohydrogen production from delignified lignocellulosic biomass |
290 |
Candida antarctica B |
Fe3O4 nanoparticles, GO nanosheets and GO/Fe3O4 nanocomposite |
Uneven surface after immobilization |
GLU or EPH |
Covalent |
64.9% |
5 |
Different catalytic systems |
291 |
Inulinase |
Fe3O4@Ag |
Spherical with the average particle diameter of around 100 nm |
EDC and NHS |
Covalent |
— |
— |
Produce high-fructose syrup by hydrolysis of inulin |
292 |
Cellulases and xylanases |
GO-MNP-Enz |
Morphological aspect of the graphite oxide surface has the shape of overlapping sheets |
EDC and NHS |
Covalent |
β-Glucosidase and β-xylosidase presented the best results (110% and 78%, respectively) while xylanase (15%) |
10 |
Producing second-generation ethanol (cellulosic ethanol) and other value-added bioproducts |
293 |
3.3 Immobilization via entrapment
Numerous methods are used for enzyme immobilization,294–297 each designed to improve the physicochemical properties of enzymes for various applications.296 The choice of immobilization technique and the properties of the enzymes significantly influence.
The degree of enzyme immobilization and the retained catalytic activity.295 Enzyme immobilization can be carried out on a variety of organic and inorganic materials,294 with factors such as the physicochemical properties of the enzyme and substrate, the need for robust attachment between the support and the enzyme, and the number of reuse cycles taken into account when determining the optimal immobilization method.297
The effectiveness of these methods is highly dependent on the support used. To optimize immobilization efficiency, the carrier should have a large surface area, good stability, substantial porous structures, and be readily adaptable to facilitate enzyme immobilization.296 Improved enzyme activity and thermal stability are observed when the support's pore size matches the enzyme's hydrodynamic size. Although organic and inorganic materials can be used for enzyme immobilization, hydrophobic nanomaterials often exhibit higher immobilization.294 An important consideration when selecting a matrix for immobilization via the entrapment approach is the efficient diffusion of substrate and product molecules.298
Among the various immobilisation methods, entrapment has proven to be among the most successful.294 Entrapment is a physical process for immobilizing enzymes on carriers or transporters,299 where enzymes are bound to a substrate by hydrogen bonding, ionic interactions, and van der Waals forces. This simple and inexpensive adsorption process can be purely physical or involve covalent bonding,294 an irreversible immobilization.296 Enzymes are confined with limited mobility in entrapment but remain in circulation as free entities.294 The enzyme becomes entrapped within the material matrix as the carrier material grows, making it more stable and accessible to separate and recycle.300 This entrapment prevents the unfolding of the enzyme, thus avoiding activity loss due to conformational changes.297 Entrapment methods allow easy tuning and optimization of the support, creating an ideal environment to stabilize and enhance enzyme activity.300 However, defects in the matrix300 and minimal adsorption interactions298 can lead to enzyme leaching, a challenge that can be overcome by combining entrapment with covalent immobilization methods.298
Enzyme entrapment can be achieved by four primary methods on gels: sol–gel methods (hard gel), cross-linking of biopolymers (hard or soft gels), polymerization to form insoluble polymers (hard or soft gels), and supramolecular assembly (soft gel),300 as shown in Fig. 7. The sol–gel method is particularly suitable for immobilising or encapsulating labile enzymes in inorganic oxide matrices, offering the advantage of low temperature and pressure conditions. The extent to which the molecule retains its native properties depends on the interaction between the matrix network and the encapsulated enzyme. This process has successfully encapsulated various enzymes, including therapeutic enzymes, cellobiase, amylase, and lipase.301 The sol–gel method, especially for hydrophobic enzymes such as lipase, forms a wide range of active biocatalytic materials, and the hybrid carriers produced can prevent enzyme leakage while providing increased mechanical stability.300,302
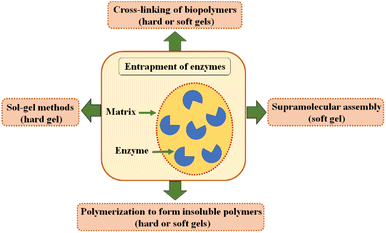 |
| Fig. 7 The four main methods for the entrapment of enzymes out on gels. | |
Recent advances in enzyme entrapment include 3D printing, metal–organic frameworks, smart gels (enzyme-responsive entrapment), ionic liquids, and hybrid materials.300 The field of 3D printing for enzyme immobilization by entrapment is, with a few exceptions, primarily dominated by hydrogel-based 3D printing, with direct ink writing being the most commonly used method.303 Enzymes such as β-galactosidase and laccase have been successfully used in 3D printable bioinks for enzyme entrapment. 3D printing is promising, particularly in industrial biocatalysis for flow reactions.300
3.4 Crosslinked enzymes
The aggregation of enzymes characterizes the method of enzyme immobilization via cross-linked enzyme aggregates (CLEAs) by cross-linking, where a collection of active molecules is linked by chemical interactions, forming cross-links that hold the enzymes together.304 Moreover, cross-linked aggregates represent an irreversible technique of enzyme immobilization, providing autonomous and reusable stable biocatalysts with high enzyme activity retention, making them applicable in various industrial fields.305,306
Enzymatic aggregates are synthesized by crosslinking enzyme aggregates prepared by mixing an aqueous protein solution with organic solvents, polymers, or anionic salts and then crosslinking with a bifunctional chemical reagent.307 The cross-linking reagent is a molecule with at least two reactive ends that bind to specific regions of the enzyme that are not essential for catalytic activity, allowing the enzyme molecules to interact with macromolecular structures308,309 physically. The resulting covalent cross-links are rigid and effectively prevent enzymatic denaturation, thereby preserving or enhancing catalytic activity and increasing enzyme stability.310 In this method of enzyme immobilization, the enzyme is not attached to a solid support.311
This enzyme immobilization protocol highlights two cross-linking immobilization approaches: cross-linking enzyme aggregate (CLEA) and cross-linking enzyme crystal (CLEC).309,311 The CLEA technique involves the addition of salts, organic solvents, or nonionic polymers to form enzyme aggregates with high catalytic activity.312 The CLEC technique is more complex and involves controlled enzyme precipitation to produce microcrystals, followed by the formation of crystal aggregates through covalent bonding with a cross-linking agent to promote this chemical interaction.313 Both protocols use cross-linking agents to form enzyme aggregates. CLECs offer several advantages, including high operational stability, catalytic activity, and ease of recycling. However, the protocol is complex and costly regarding time and resources.314–316 CLEAs, on the other hand, are advantageous due to their simplicity, low cost of protein processing, and robustness of the biocatalyst. However, cross-linking agents can sometimes lead to structural changes or enzyme formation that may block active groups.317
In their innovative research, Akkas et al. (2020) presented a novel method for immobilizing ureases. This method involves a reticulated enzyme aggregation technique using lyophilization to enhance enzyme stabilization. In this study, lyophilization of bovine serum albumin (BSA), crosslinking with polyaldehyde dextran (DPA), and pH optimization of the crosslinker were used to immobilize jack bean urease (JBU). Notably, the relative catalytic activity of urea-CLELs was approximately 1.47 times higher than free urease's. In addition, the biocatalyst exhibited enhanced thermal stability, allowing it to function in reactions at temperatures up to 85 °C while maintaining catalytic efficiency. According to the authors, the shelf life of the immobilized enzyme was extended to 4 weeks with unchanged catalytic activity. In addition, the recyclability of the enzyme was demonstrated, as its residual activity remained unchanged after 10 reaction cycles, and its thermal stability was nearly doubled. This approach opens new perspectives for enzyme engineering, providing access to new information and potential industrial applications.316
3.5 Covalent attachment
The covalent enzyme immobilization protocol is one of the most commonly used methods because it increases or maintains operational stability, thereby improving catalytic performance.318 This method involves the formation of stable covalent complexes or bonds between the functional groups of the support and the enzyme, making it a chemical method of immobilization.319 The enzyme-binding functional groups need not be essential for enzyme activity.311 The primary functional groups involved in interactions with the support are typically found in the side chains of the enzyme, such as cysteine (thiol group), aspartic acid, glutamic acid (carboxyl group), and lysine (ε-amino group).320 The major functional groups capable of interacting and forming a covalent bond with these side-chain enzyme groups include the imidazole, thiol, indole, hydroxyl, amino, and sulfhydryl groups.321–324
In most covalent coupling immobilization protocols, two essential steps are required to maintain the technique's efficiency.325 The first step involves the activation of the surface of the solid support. In this step, one region of the ligand molecule covalently interacts with the surface of the support, activating the support while leaving the other region of the ligand molecule free for interaction with the enzyme.326 Several activating reagents can be used for this purpose, including glutaraldehyde, carbodiimide, glycidol, epichlorohydrin, and formaldehyde.327 The binding molecules bridge the support surface and the enzyme at this stage through covalent interactions. These binding reagents are multifunctional, thus allowing for this covalent coupling.328 The next step involves the interaction between the activated solid support and the non-essential region of the enzyme. The pre-activated support forms a covalent bond with the enzyme, establishing a bond between the free portion of the binding reagent and the enzyme binding region.329,330 The selection of the activating reagent and the immobilization protocol is determined based on the analysis of the support surface and the structural and conformational characteristics of the enzyme.331
Covalent fixation is characterized as an efficient technique for the immobilization of enzymes.332,333 This protocol provides vital links between enzymes and solid support. Therefore, the leaching of the enzyme immobilized on the support is minimal, thus improving the stability of the immobilized enzymes and the immobilization yield.334,335 Notably, the high uniformity of the bonds between enzyme and support allows reasonable control of immobilised enzyme amounts.328,335,336
Much of the work published in the last five years has shown that the parameters associated with enzyme immobilization (e.g., immobilization yield, protein content, enzyme activity, thermal stability, and pH) are favourable.328 In particular, the expressed activity of the biocatalyst is often maintained or increased after immobilization, as the conformations of the enzymes remain unchanged.335 Therefore, the covalent fixation approach to immobilization mitigates the desorption phenomenon, reduces the spontaneous deactivation rate of the enzyme, and prolongs its useful life and operational stability.
The covalent fixation technique is often preferred as the primary immobilization protocol because of its proven efficacy in the literature. Helm et al. (2019) reported the covalent immobilization of the hydroxy-nitrile lyases HbHNL (from Hevea brasiliensis L.) and MeHNL (from Manihot esculenta C.) on porous silica substrates, achieving high immobilization performance. Because of the high enantioselectivity of these enzymes, biocatalysts have been used in kinetic resolution reactions to achieve superior chiral construction. As a result, a project was developed for a continuous flow micro-reactor with minimal HNL loads, resulting in a significant improvement in catalytic performance compared to the batch system. The application of the constant flow system enabled the rapid production of chiral cyanohydrins with high conversion (97%) and high enantiomeric excess (98%) in only 3.2 minutes, using the lowest possible enzymatic load. The monolith immobilization protocol achieved high protein loads with immobilization yields of 89% (11.3 mg total protein; 1120 U per monolith) and 72% (17.4 mg total protein; 1310 U per monolith) for HbHNL and MeHNL, respectively, demonstrating the overall versatility of the covalent immobilization method. This protocol increased enzyme activity, improving substrate conversion rates and superior chiral construction.337
4. Enzymatic magnetic nanoparticle applications
4.1 Oxirreductases
Oxidoreductase (EC 1) enzymes include at least 26 subclasses of enzymes (https://enzyme.expasy.org/enzyme-byclass.html) that play a central role in metabolic pathways critical for cell function (https://www.brenda-enzymes.org/). They catalyze oxidation–reduction reactions that involve the transfer of electrons, either as free entities or as hydrogen atoms, between a donor (reducing agent, which is oxidized) and an acceptor (oxidizing agent, which is reduced), or the transfer of oxygen atoms from O2, which is reduced, to an organic molecule, which is oxidized. These reactions account for at least one-third of all enzymatic reactions recorded in the BRENDA (Braunschweig ENzyme DAtabase).338,339 Oxidoreductases are a diverse group of enzymes that play a central role in various chemical oxidation–reduction reactions. These enzymes facilitate the transfer of electrons or the performance of oxidation and reduction reactions between different substrates.338,340 They have a variety of properties that make them useful in many fields, including agriculture, environmental management, medicine, and analytical chemistry. These enzymes include oxidases, peroxidases, dehydrogenases, and oxygenases.338,341,342
Oxidoreductases can be functionally classified into several categories: (i) oxidases catalyze oxidation reactions using oxygen as the final electron acceptor. A well-known example is laccase, one of the first oxidases studied.338,343 (ii) Peroxidases: these enzymes catalyze the oxidation of substrates using a peroxide, with hydrogen peroxide (H2O2) being the most commonly used peroxide.344 (iii) Dehydrogenases: these enzymes oxidize substrates by transferring electrons from hydrogen atoms to acceptor or donor cofactors. Some dehydrogenases can use molecules other than oxygen as electron acceptors.338,345 (iv) Oxygenases: these enzymes catalyze oxidation reactions that directly incorporate oxygen into the substrate.346
Kouasse et al. (2020) used cholesterol oxidase (CHO) to be bound to magnetic nanoparticles via carbodiimide activation. FTIR spectroscopy analyses confirmed the binding between CHO and the nanoparticles, with efficiencies ranging from 98% to 100%. After nanoparticle association, comparative kinetic studies between free and immobilized CHO showed significant stability and enzymatic activity improvements. In addition, the bound enzyme exhibited improved resistance to variations in pH, temperature, and substrate concentration.347 Cholesterol oxidase has industrial and commercial importance, particularly in bioconversions, for clinically determining total or free serum cholesterol and agricultural applications.348,349
Huang et al. 2023 covalently bound lipase to magnetic iron nanoparticles using carbodiimide activation. The efficiency of lipase binding to magnetic nanoparticles was confirmed by FTIR analysis. Compared to the free enzyme, the nanoparticle-bound lipase showed a 1.41-fold increase in activity, a 31-fold improvement in stability, and better tolerance to temperature and pH variations.350
Ren et al. 2023 carried out the immobilization of yeast alcohol dehydrogenase (AmDH) on titanium nanoparticles. First, AmDH was coated with polyethyleneimine (PEI), which created a hydrophilic environment that stimulated the hydrolysis and condensation reaction of titanium, resulting in the formation of nanoparticles. This process created a rigid matrix that acted as a pocket, preventing the enzyme structure from unfolding. The immobilized enzyme, named AmDH-PEI-Ti, retained 80% of the activity observed in the free enzyme, with an entrapment efficiency of 90%, showing potential for industrial production.351,352
Oxidoreductases represent biocatalysts of great interest, with significant potential in producing polymeric building blocks, sustainable chemicals and materials derived from plant biomass in lignocellulose biorefineries. However, despite these promising applications, the chemical industry, especially in large-scale chemical manufacturing, has not yet widely adopted enzymatic oxidation reactions.353
This reluctance is mainly attributed to the lack of biocatalysts that possess the necessary selectivity, are commercially available, and are compatible with stringent process conditions. Such conditions include high substrate concentrations, use of solvents, and strongly oxidative environments. Overcoming these challenges is crucial for effectively integrating enzymatic oxidation reactions into industrial processes, presenting the potential to contribute significantly to the sustainable production of diverse chemical products and bio-based materials.353,354
4.2. Transferases
Transferases are enzymes that are part of a group responsible for transferring various functional groups, such as the methyl group of a compound, to other groups that accept them, thereby creating a bond between the donor group and the acceptor group.355 Transferase enzymes can be divided into subgroups based on the transferred functional groups. The first subgroup, known as glutathione S-transferases (GSTs), catalyzes the transfer of a methyl group from glutathione (GSH). The second subgroup, N-acetyltransferase (NATs), transfers an acetyl group. The third subgroup, sulfotransferases (SULTs), transfer one or more sulfate groups. The fourth subgroup, UDP-glucuronosyltransferases (UGTs), transfers a glycosyl group.356,357 The scope and outcomes of transferases are still poorly understood and require further investigation. However, it is known that transferase enzymes are mainly used in the health field, especially in pharmacology and biochemistry, as their primary function is to conjugate drug metabolites, making the studied drugs more hydrophilic, facilitating their absorption and allowing for their natural elimination.
Glutathione S-transferase enzymes (GSTs) are the most extensively studied of the transferases and are known as drug-metabolizing enzymes (DMEs). Based on the sequential catalysis analysis, these enzymes can be divided into phases I, II, and III, each with a different role.358 Glutathione S-transferases (GSTs) are part of phase II and crucial enzymes in combating oxidative stress. They act as detoxification enzymes, conjugating products from phase I reactions. Phase I involves the oxidation of drugs, and the primary function of GSTs is to conjugate drug or xenobiotic metabolites to make them more hydrophilic. This occurs through several pathways, including methylation, glutathionylation, acetylation, and sulfation. Sulfotransferase enzymes (SULTs) are also part of phase II and are involved in the sulfation pathway.359 In addition to their detoxification role, GSTs have isomerase and peroxidase functions and can bind to numerous endogenous substances and exogenous ligands.360,361
Transglutaminase (TGM) enzymes are a subgroup of transferase enzymes. Transglutaminases (TGMs) facilitate intramolecular and intermolecular cross-links between glutamine and lysine residues, with the former serving as acyl donors and the latter as acyl acceptors. These residues are commonly found in peptides and various proteins.362,363 Transglutaminases (TGMs) play an essential role in the food industry, enhancing the properties of proteins and improving the texture and overall quality of food products. Viable technological methods contribute to more efficient use of raw materials, thereby improving the cost–benefit ratio of food production.364,365 TGMs also have potential applications in other industries, including the leather and textile sectors.366
In a recent study, transglutaminase enzymes (TGMs) were subjected to enzymatic immobilization using magnetic nanoparticles (MNPs). Microbial TGMs were investigated to create cleaner and more environmentally friendly industrial applications. Immobilization of TGMs was achieved by covalent attachment, starting with the preparation of MNPs, which were then modified by a co-precipitation process with Fe2+ and Fe3+. Subsequent modifications included carboxymethyl dextran (CMD) and CMD with oleic acid. The MNPs were activated with the crosslinking agent's pentamethylene hexamine (PEHA) and glutaraldehyde (GA), the latter being a common choice for enzyme immobilization techniques.367 The immobilized TGMs on magnetic nanoparticles were thoroughly analyzed, including protein concentration, activity, thermal stability (in both free and immobilized forms), stirring speed, and reuse (cycles). The study concluded that the enzymes were quickly recovered from TGMs immobilized in CMD-oleic and CMD-MNPs, with CMD-MNPs showing the highest success rate in terms of immobilization. The enzymes were also hyperactivated, showing % residual activity of 110% and excellent thermal stability at 50 °C and 70 °C. This led to the conclusion that the immobilization studies with magnetic nanoparticles of TGMs were successful and could be used in industrial applications as an economical and biodegradable biocatalyst, with potential applications mainly in the leather and wool industries.368–370 In a recent study, transglutaminases (TGMs) were subjected to enzymatic immobilization, a widely used method for enzyme stability. Two different approaches were used, magnetic cross-linked enzyme aggregates (mCLEAs) and cross-linked enzyme aggregates (CLEAs), to obtain promising results regarding the enzymatic activity of the enzymes immobilized by these methods. After studying the techniques, the TGMs were subjected to a colourimetric hydroxamate procedure using CBZ-glutamine glycine (CBZ-Gln-Gly) as an amine acceptor substrate to identify the activity of the TGMs.371 The TGMs showed the highest results among the enzymes included in the study. The ideal reagent for CLEAS TGMs was 2-propanol, resulting in a residual activity of 231%. In addition, both immobilization techniques, CLEAS and mCLEAS, showed more excellent storage stability when exposed to 4 °C for 44 days. mCLEAS TGMs showed very positive results compared to CLEAS TGMs, with residual activity of 53% under these conditions, providing more excellent stability of the immobilized TGMs. The study showed that mCLEAS had better operational stability and catalytic efficiency than CLEAS, demonstrating that magnetic nanoparticles significantly affect stabilization results. The two immobilization techniques have particular specificities and depend on the behaviour of the enzymes in specific environments and conditions to which they are subjected. Both immobilization techniques are promising, but the enzymes have no particular rules or parameters, whether CLEAS or mCLEAS. Both methods could be used in many future bioapplications.372
Scientists are increasingly studying and analysing transferases worldwide, with several studies presenting promising analyses for future applications. Although studies on immobilization in magnetic nanoparticles (MNPs) and transferases are still in their infancy, guidelines for future scientific analysis already exist, especially in the food industry.373–375 Although still in its infancy, scientific work on transferase enzymes aimed at enzymatic immobilization is already available, especially for TGMs. For example, an enzymatic membrane reactor (EMR) has been developed to recover whey protein.376 In addition, a study describes the usefulness of TGM enzymes for immobilization in poly(N-isopropylacrylamide).377 Glutathione S-transferases (GSTs) are used in enzymatic activity studies because of their high detoxifying properties.378 They are also used to separate and purify proteins when labelled with GSTs. These enzymes protect the body from chemical carcinogenesis and conjugate glutathione (GSH) to various electrophilic substrates.379 Transferases are expected to become the most studied group of enzymes for enzyme immobilization because they have desirable properties for all fields, especially for current studies focusing on cleaner, ecologically correct, and economically sustainable technologies. These enzymes have large-scale and industrial applications.
4.3. Hydrolases
Hydrolases (EC 3) catalyse hydrolysis reactions in living organisms.380,381 They are divided into subclasses based on the particular bonds they target during chemical reactions. The diversity and adaptability of these enzymes in hydrolyzing a wide range of substrates, from small to large molecules, make them particularly attractive for industrial applications.382
Of the various subclasses of hydrolases, certain enzymes such as tannases, α-amylases, β-galactosidases, proteases, phospholipases, and various lipases have been used in immobilization processes using magnetic nanoparticles as supports.383–389 Table 2 provides some examples of these applications in industrial processes.
Table 2 Applications of immobilized hydrolases on magnetic nanoparticles
Enzyme |
Support |
Immobilization yield |
Application |
Reference |
Note: magnetic nanoparticles coated with silica. Magnetic nanoparticles with glycidoxypropyltrimethoxysilane (GOPTS), 5-AIPA and Co2+. Superparamagnetic magnetite nanoparticles modified with polyethyleneimine (PEI). Core–shell cobalt ferrite nanoparticles. Magnetic nanoparticles composed of polyaniline-coated diatomaceous earth. Magnetic nanoparticles cross-linked with chitosan. Melamine-glutaraldehyde dendrimer-like polymers grafted on aminated magnetic nanoparticles. |
Rhizomucor miehei (RML) and Thermomyces lanuginosa (TLL) |
Fe3O4@SiO2a |
81–100% |
Biodiesel production |
390 |
Bacillus subtilis |
ZnO nanoparticles |
71.9–79.5% |
Detergent formulation |
391 |
Rhizopus oryzae (ROL) |
Magnetite nanoparticles |
74.7% |
Synthesis of triacylglycerols |
392 |
Pseudomonas fluorescens (PFL) |
AGMNP-Co2+b |
89% |
Biodiesel production |
393 |
Thermomyces lanuginosus (TLL) |
Fe3O4@PEIc |
69.6–74.4% |
Synthesis of ethyl valerate |
394 |
Rhizomucor miehei (RML) |
Carbon nanotubes |
95–98% |
Hydrolysis of p-nitrophenyl butyrate |
395 |
Bacillus atrophaeus (BaL) |
Graphene oxide nanosheets |
81.35% |
Synthesis green apple flavor ester |
396 |
Proteases produced by solid state fermentation |
Magnetic iron oxide nanoparticles |
93–96% |
Hydrolysis of different protein sources |
397 |
Rhizopus oryzae (ROL) |
CoFe2O4d |
77.43% |
Biodiesel production |
398 |
Candida rugosa (CRL) |
Multiwalled carbon nanotubes with Co |
88.5% |
Synthesis of fruit flavors |
399 |
Tannase from Aspergillus ficuum |
mDE-PANIe |
90% |
Removing tannins from aromatic drinks |
400 |
Bacillus subtilis A (BsLA) |
Fe3O4@SiO2 |
89.94–93.72% |
Hydrolysis of p-NPC |
401 |
Candida antarctica B (CALB) |
Fe3O4@CHIf |
95% |
Photo-curable functional esters |
402 |
Burkholderia cepacia (BCL) |
GTAMNPsg |
98.8% |
Standard esterification reaction between lauric acid and 1-dodecanol |
403 |
Studies indicate that proteases are increasingly used for enzymatic immobilization with magnetic nanoparticles.404–406 Ibrahim et al. (2021) demonstrated that the nanobiocatalyst prepared by covalent immobilization of alkaline protease from Salipaludibacillus agaradhaerens in mesoporous double-core nanospheres (DMCSS) exhibited enhanced enzyme stability in high concentrations of NaCl, solvents, surfactants, and commercial detergents. Furthermore, the immobilized protease exhibited excellent operational stability, retaining 79.8% of its activity after ten cycles, thus proving to be a promising nanocatalyst for industrial applications.407 Razzaghi et al. (2018) concluded that immobilization of the protease Penaeus vannamei in zinc sulfide (ZnS) nanoparticles improved the functionality of the enzyme at high temperatures, extreme pH conditions (pH 3 and 12) and during storage while also extending its optimal temperature range.408
In their study, Li et al. (2018) aimed to develop a novel biocatalyst for tea infusion clarification. To achieve this, they immobilized Aspergillus niger tannase on chitosan-coated magnetic nanoparticles (Fe3O4-CS). The immobilized tannase retained more than 50% of its initial activity even after eight reaction cycles. It exhibited improved pH and thermal stability and effectively enhanced the colour of both black and green tea infusions.385
In recent years, magnetite (Fe3O4)-based nanoparticles have emerged as a successful choice for immobilizing various lipases due to their numerous advantages, including high stability, low toxicity, and easy separation by an external magnetic field.392,401,409–411 Sarno et al. (2017) used citric acid-functionalized Fe3O4 nanoparticles to immobilize Thermomyces lanuginosus (TLL) lipase and applied the resulting biocatalyst in banana flavour synthesis. In particular, they achieved a remarkably high activity recovery compared with the free lipase, with values reaching up to 144% at pH 7 and 323% at pH 7.5. Furthermore, the immobilized enzyme exhibited superior stability and improved reusability, retaining 75% of its initial activity after 60 days of storage during the third cycle of banana aroma production and 64% after 120 days. These results demonstrated the improved performance of the immobilized enzyme compared to its free counterpart.412
Lipases immobilized onto magnetic nanoparticles are also widely used in biodiesel production reactions.413–418 In a recent study, Zulfiqar et al. (2021) developed a novel nanobio-catalyst by immobilizing lipase from Aspergillus niger onto titanium dioxide nanoparticle-modified polydopamine (PDA-TiO2). They used it to synthesise biodiesel via enzymatic transesterification using Jatropha curcas seed oil. The immobilized lipase exhibited greater resilience to changes in pH and temperature conditions. Moreover, the optimal biodiesel yield of 92% was achieved by conducting the transesterification process for 30 h at 37 °C with a 10% concentration of the nanobio-catalyst.419
Thus, research confirms hydrolases' considerable diversity and adaptability while demonstrating the significant benefits of using magnetic nanoparticles to aid immobilization. This has improved their properties and efficiency in various reactions, facilitating their application in numerous industrial processes.
4.4 Lyases
Lyases (EC 4) are enzymes that catalyze addition and elimination reactions. They cleave chemical bonds but do not undergo this process by oxidation or hydrolysis.420–422 Studies have demonstrated their use in vital areas such as agriculture and food423,424 and medicine.425,426 Some of the applications of these enzymes immobilized on magnetic nanoparticles are shown in Table 3.
Table 3 Applications of lyases immobilized onto magnetic nanoparticles
Enzyme |
Support |
Immobilization yield |
Application |
Reference |
Alginate extracted from Escherichia coli |
Fe3O4 |
97.8% |
Antioxidant and antiapoptotic bioactivities in human umbilical vein endothelial cells |
427 |
Pectate lyase from Clostridium thermocellum |
Fe3O4 |
96.5% |
Bioscouring of coarse cotton |
428 |
Benzaldehyde lyase from Pseudomonas fluorescens |
Epoxy-chelate magnetic support |
87% |
Synthesis of critical synthons for pharmaceutical products |
429 |
Pectate lyase |
Calcium hydroxyapatite nanoparticles and single-walled nanotube |
>70% |
Processes of both high and low temperatures |
430 |
Phenylalnine ammonia lyase |
Hybrid nanoflowers |
90% |
Biosensors |
431 |
Cystathionine γ-lyase |
TiO2 |
95% |
Biomineralization |
432 |
Pectin lyase from Acinetobacter calcoaceticus |
Magnetic carboxymethyl cellulose nanoparticles |
80% |
Purification of some fruit juices |
433 |
Like other classes of enzymes, lyases have their subclasses. Among them are alginate lyases, which are synthesized by algae, bacteria, marine molluscs, fungi, and viruses and play essential roles in the degradation and assimilation of alginate.434–436 In the study by Jiang et al. (2021), the alginate lyase AlgL17 was immobilized onto magnetite (Fe3O4) nanoparticles. In the end, they obtained a new biocatalyst that showed superior thermal and pH tolerance, excellent storage stability, and capacity for reuse. This biocatalyst was used to produce alginate oligosaccharides, which showed antioxidant activities and prevented cell self-destruction, being effective against hydrogen peroxide-induced oxidative stress in human umbilical vein endothelial cells.427
Another study by Shin et al. (2010) revealed a new biocatalyst prepared by immobilizing marine alginate lyase from Streptomyces sp. (ALG-5) in magnetic iron oxide and hybrid magnetic silica nanoparticles. It exhibited the most significant alginate degradation activity and could be reused more than ten times after magnetic separation.437
Another subclass of lyases is pectate lyases (PLs), which act on the degradation of pectin produced by pathogenic organisms and can potentially have industrial applications.438–441 Chakraborty et al. (2017) immobilized the recombinant Clostridium thermocellum pectate lyase in magnetite nanoparticles and, from this process, produced a biocatalyst with more significant activity, improved thermal stability 32 times at 80 °C and 14 times at 90 °C, and with the ability to be reused for five cycles followed by 70% of the initial activity. Its application in the biofouling of cotton fabric showed an efficient removal of pectin from the fabric surface.428
An analysis of the existing research shows that lyases are enzymes that have not been widely studied in immobilization processes using magnetic nanoparticles as a support. However, the limited number of published studies highlights the potential applicability of these enzymes and the advantages of immobilizing them on these particles.
4.5. Isomerases
Isomerases catalyze reactions that can induce intramolecular changes that convert the substrate into an isomer.
Immobilization magnetic nanoparticles increase isomerases' stability and offer advantages in the reaction medium because of their large surface area, ease of separation from the reaction medium by an external magnetic field, mobility, and mass transfer. Consequently, isomerases immobilized on magnetic particles find applications in various industrial sectors, as shown in Table 4.
Table 4 Applications of industrial isomerases
Enzyme |
Application |
References |
L-Arabinose isomerase |
Food industry |
442 |
Glucose isomerase |
High fructose corn syrup (HFCS) production |
443 and 444 |
Protein disulfide isomerase (PDI) |
Thiol-disulfide of ADAM17 and αIIbβ3 |
444 |
Triose phosphate isomerase |
High definition proton magnetic resonance study |
445 |
D-Psicose 3-epimerase |
Food additives |
444 and 445 |
The industrial production of phenylacetaldehyde is essential in the flavour and fragrance industry because it synthesises various products, including insecticides, disinfectants, and pharmaceuticals. This aromatic aldehyde is obtained from the isomerization of styrene oxide in the presence of alkali-treated silica or various zeolite compounds. In addition, oxidation of 2-phenylethanol can be performed using hexavalent chromium compounds or rhodium complexes.
The enzyme L-arabinose isomerase is of industrial importance because of its applicability. The process can occur in vivo, catalyzing the isomerization of L-arabinose to L-ribulose. In vitro, it can generate the reaction of D-galactose to D-tagatose in nutraceutical foods.446 Therefore, the overall applicability of the process can be optimized when L-arabinose isomerase is immobilized on chitosan supports with magnetic nanoparticles.447,448 Moreover, using chitosan with magnetic nanoparticles acts in applications, sewage, water treatment, and food preservatives in the food industry and presents a tremendous antioxidant specificity and antimicrobial practice.449
Lactose, a by-product of yoghurt and cheese production, is used in various food and pharmaceutical products.450 However, industrial production of lactose is limited by its low solubility, sweetness, and bioavailability.451 Concentrated lactose yields less sweet D-tagatose than sucrose.452 D-Tagatose, an isomer of D-galactose, exists in α-D-tagatose-2,6-pyranose, β-D-tagatose-2,6-pyranose, α-D-tagatose-2,5-furanose, and β-D-tagatose-2,6-furanose structures catalyzed by the enzyme L-arabinose isomerase.453 D-Tagatose synthesis involves catalytic isomerization at high pH using soluble bases that are neutralized with sulfuric acid after conversion. However, D-galactose isomerization with essential catalysts results in lower D-tagatose selectivity due to by-product formation.454 Using magnesium-based catalysts (MgO) improves the isomerization of glucose, galactose, and arabinose with satisfactory yields. Supported by various materials such as carbon nanotubes, hydrotalcite, and aluminates, the stabilization of MgO during the reaction is improved.455–457
Glucose isomerase, an enzyme that catalyzes reversible isomerization reactions, is critical in the conversion of D-glucose and D-xylose to D-fructose and D-xylulose, which are widely used in industrial contexts.458 In the food sector, its role is prominent in producing high fructose corn syrup (HFCS), a mixture of glucose and fructose suitable for people with diabetes.459,460 The process can also involve the interconversion of xylose to xylulose by glucose isomerase. Therefore, magnetic particle immobilization provides an efficient method for easy recovery and reuse while reducing costs.461 As a result, glucose isomerase and other enzymes present an opportunity for large-scale industrial mobilization and are widely used in various food industry sectors.462,463
The disulfide isomerase (PDI) enzyme is a redox chaperone with applications in thiol oxidoreductase and isomerase in nascent proteins in the endoplasmic reticulum.464 The PDI enzyme has several functions at the cell surface, primarily maintaining redox homeostasis and the thiol-disulfide isomerization process of ADAM17 and αIIbβ3.465 Therefore, the effects associated with thrombosis, platelet activation, and vascular thiol isomerases466 can be reduced or inhibited by using the PDI process as an antithrombotic criterion.467 Using chitosan-polyacrylic hybrid microspheres offers advantages regarding Hof stabilization, temperature, and operation during its application. Consequently, a technique is required for GI mobility with a high enzymatic reactivity process and stabilization at the junction of iron changes.468
Triosephosphate isomerase extracted from rabbits and chicken was analyzed by high-resolution proton magnetic resonance imaging. The analytical techniques detected five possible histidines in the chicken protein and one histidine in the rabbit enzyme over a pH 5.4 to 9 range in the amino acid sequences.469 Specifically, the resonances of histidine 100 in chicken and rabbit and only histidine 195 in the chicken enzyme were considered.470 Histidine 100 is destabilized by the addition of ligands such as D-glycerol-3-phosphate, which changes the conformation of the enzyme but remains stabilized in the presence of inhibitors such as phenyl phosphate. In this way, a peptide-NH proton exchange occurs in the histidine resonance regions, eliminating any deformation of the enzyme.471
D-Psicose production via the epimerization reaction of D-fructose using class 3 epimerases is under consideration.472 D-Psicose, the carbon-3 epimer of the sugar D-fructose, is rare and has a lower sugar content than sucrose. It is a food additive with functions such as suppressing glucose in type 2 diabetes, producing a near-defensive effect, and inhibiting hepatic lipogenic proteins.473 D-Tagatose 3-epimerase from Pseudomonas cichorii catalyzes the C-3 epimerization of D-fructose to produce D-psicose.474 The D-psicose-3-epimerase from Agrobacterium tumefaciens was selected because of its substrate preference. This enzyme is also present in several other species, such as Ruminococcus sp. and Clostridium bolted.475 However, certain factors limit the production of D-psicose-3-epimerase on an industrial scale. The immobilization process can optimize the reaction yield, and titanium dioxide (TiO2) is often used as a support material for nanoparticle immobilization due to its conductivity physical and chemical stability.476–478
4.6. Other enzymes
Trehalose is an enzyme developed by a mechanism that maintains the processability and biological properties while preserving the activity of macromolecules.479 Therefore, the reaction process through metal–organic frameworks (MOFs) is optimised using ZIF-8 to permeabilize two encapsulated enzymes from Bacillus subtilis coated with glycemic isomerase. The enzyme glucose isomerase converts trehalose synthase and the by-product glucose to fructose for industrial applications.480 Because of its stability, the trehalose-protected enzyme is used in biological studies, agriculture, and the pharmaceutical and food industries. In addition, the food industry creates a stable, protective layer to delay nutrient loss and adjust food flavour.481,482
Lipases are enzymes widely used in biodiesel synthesis due to their mild reaction conditions, easy product recovery, and environmental sustainability compared to chemical processes.483,484 Therefore, immobilization of lipases allows reuse and improves stability and catalytic activity. The support choice provides the most suitable surface area and low cost.485 Using magnetic silica/iron oxide nanoparticles promotes advantages in partitioning the material controlled by a magnetic field.486 Covalent bonds have been used on various supports to produce the reaction between an active group on the support and the amino acid residue of the lipase.487
The enzyme Burkholderia cepacialipase (BCL) is considered a chiral high-resolution catalyst.488 Immobilization in nanoparticles increases enzyme activity and stability. When immobilized in nanoparticles, they can be modified by dendrimer polymers to protect the structure of Burkholderia cepacialipase and thus increase the contact levels between substrates and the enzyme centre.489 Applications in the pharmaceutical field through drugs are chiral drugs that present differences in dynamics and kinetics in vivo, requiring the separation of drug enantiomers. The surface modification controls the morphology (i.e., improves the affinity of the compounds by the active groups), increasing the charge and favouring the recovery of the enzyme. Therefore, these chiral programs are widely used in the spice, textile, and pharmaceutical industries.490
The protein from Thermomyces lanuginosus (TLL) is a lipase with high catalytic efficiency due to its enantioselectivity and isoelectric point (pI).491 Therefore, the use of the support with the reactive group divinyl sulfone (DVS) with polyethyleneimine (PEI) provides the hetero-functionality of the DVS-PEI support to generate intense multivalent covalent bonding.492 Thus, the octyl DVS matrices in the immobilization process by superparamagnetic nanoparticles allow interfacial activation to occur, ensuring stabilization through the points of multivalent bonds.493,494 The coating by nanoparticle immobilization protects the surface from oxidation and minimizes non-specific interactions. Thus, covalent nanoparticle immobilization optimized on DVS-containing supports provides a better pH ratio, incubation time, and different blocking intermediates. Therefore, its applications are targeted to the medical and fine chemical industries.495–497
Glutathione (GSH) enzyme is a tripeptide protein (γ-L-glutamyl-L-cysteinyl-glycine). When used with GSH-agarose, it allows the isolation and purification of recombinant protein with glutathione S-transferase (GST) activity by GSH-GST interaction chromatography.498 The composition of GSH has one free amino group, two free carboxyl groups and several free thiol-reduced structures. Therefore, the GSH enzyme and agarose microbead are activated and cross-linked by the amino group medium.499 Its application in closed GSH nanoparticles is used to conjugate folic acid in cancer cell detection.500,501 Iron oxide nanoparticles are coated with GSH and are also used to promote the stability of immobilized GSH through covalent bonds such as tetraethoxysilane (TEOS).502 Thus, magnetic nanoparticles attached to GSH can couple enzymes fused to GST through the GSH-GST interaction. In this way, the protease enzyme used with nanoparticles is bound to GSH in identifying the three-dimensional structure as they improve the stability and solubility of the protein.503,504
5. Bioreactor projects with enzymatic magnetic nanoparticles
The content presented above highlights a growing interest in nanotechnology applications in bioreactor projects, focusing on using enzymatic magnetic nanoparticles. Dutta S. et al. (2023)505 highlight the use of nanoparticles in various stages of bioethanol production from lignocellulosic (LB) materials, helping to overcome challenges associated with complex compositions and inefficient degradation processes. The authors outline nanotechnological methods during pretreatment that offer significant advantages for different types of LB, improving both biofuel yield and quality. Recent experiments have shown that using magnetic nanoparticles offers remarkable advantages, facilitating the recovery and reuse of immobilized enzymes,506–508 leading to an overall reduction in process costs.
The study by M. V. C. da Silva et al. (2023)509 highlights the creation and verification of methods and processes for using 2-ethylhexyl oleate catalyzed by Candida antarctica lipase immobilized in poly(styrene-co-divinylbenzene) magnetic particles (STY-DVB-M). The detailed analysis focuses on the physical properties of the STY-DVB-M copolymer, such as the glass transition temperature of 85.68 °C and the onset of thermal degradation, which occurred at 406.66 °C, demonstrating the importance of support stability in bioreactors. Additionally, the work investigates the influence of magnetic field strength on reaction yield and productivity, emphasizing the versatility and control enabled by magnetization in the systems,510,511 crucial aspects when exploring bioreactors with magnetic nanomaterials.
N. K. Abd-Elrahman et al. (2022)512 deal with the production of biohydrogen by microbial electrolysis cells (MECs). By evaluating various parameters, including the configuration of MECs, electrode materials, substrates, pH, temperature, applied voltage, and nanomaterials, the MEC stands out for its efficiency in converting substrates to hydrogen, achieving between 80 and 100% efficiency, compared to dark fermentation with 33% and water electrolysis with 65%. The preferential choice of carbon materials due to their high porosity highlights the importance of careful material selection,513,514 while the use of nanomaterials in MECs to increase the efficiency of anodic and cathodic reactions indicates the strategic potential of this application in bioreactors. Their approaches validate and suggest the feasibility of integrating both methods discussed in bioreactor projects with enzymes in magnetic nanoparticles.515
Although at an early stage of development, both studies emphasize the urgency of further research to ensure the improvement and effectiveness of bioreactors considering kinetic and engineering aspects, another emerging challenge is the aggregate use of AI and computer simulations.509,516,517
6. Study of countries, journals, and institutions
Bibliometric data analysis provides a quantitative and objective view of scientific publications, allowing the identification of the most influential countries in the production of knowledge in a given research area. Brazil, China, and Spain emerged as the most important countries regarding citations and a number of publications on the research topic. As shown in Fig. 8, Brazil recorded 2450 citations associated with 80 publications, followed by China with 1794 citations and 58 publications, and Spain with 1683 citations and 60 publications during the study period. These data highlight the significant impact of research on the topic in the international academic and scientific sphere. The high production of articles and the substantial number of citations attributed to these countries indicate an active interest and a significant contribution to the advancement of knowledge in this specific field.
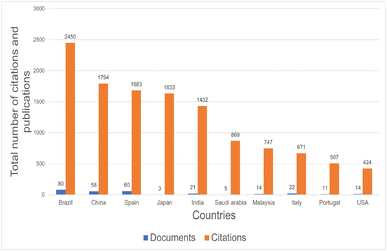 |
| Fig. 8 Prominent countries have published the most and were most cited in magnetic nanomaterials for enzyme immobilization in the last five years. | |
Fig. 9A illustrates the scientific collaboration between countries, with Brazil and China emerging as central players in this network. Brazil (58% of total citations) and China (42% of citations) are the most cited countries and have strong connections, as indicated by their size, the number of connections with other countries, and the thickness of the connecting lines. This suggests that both countries are major contributors to global research and have extensive international connections. Strengthening these collaborations could further promote the exchange of knowledge and resources worldwide. Fig. 9B visualises the temporal landscape of cross-country collaborations from 2016 to 2023. The map shows the strength of connections between countries and their clusters, with purple shades representing older years and light green shades representing more recent publications. This progression suggests a continued consolidation of research over time. In particular, clusters such as Brazil, China, Spain, Italy, and the United States maintain their relevance and significant contribution to knowledge in the field, laying a solid foundation for future scientific progress.
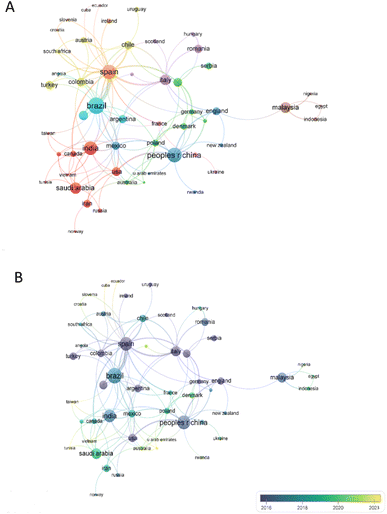 |
| Fig. 9 Bibliometric maps of country co-authorship. (A) Network of clusters of most cited countries. (B) Overlay visualization of country link power. | |
In Fig. 10A, we observe the importance of leading journals in different groupings (represented by clusters in yellow, red, and purple), with a focus on the journal with the highest impact, the Journal of Molecular Catalysis, which has 2441 citations across 63 publications, averaging 38.74 citations per article. This journal stands out as a central hub within its respective cluster (yellow) and explores the molecular and atomic aspects of catalytic activation and reaction mechanisms. We also highlight the Process Biochemistry cluster (purple), with 32 publications and 422 citations, focusing on processes related to bioactive molecules and living organisms. The Biochemical Engineering Journal (red), with 354 citations and 11 publications in the research topic clusters, is instrumental in developing biological processes, from preparing raw materials to recovering relevant products for industry.
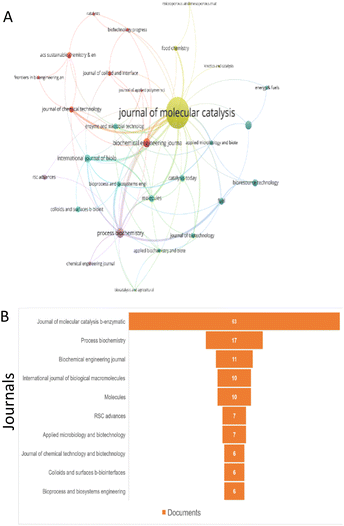 |
| Fig. 10 Bibliometric analysis map of country networks. (A) The most influential journals. (B) Number of significant documents per journal. | |
The presence of these journals in the same thematic area underscores the concentration of efforts and interests in specific areas of science as well as the active networking and collaboration among researchers working in this field. This interconnectedness is fundamental to the exchange of knowledge. Furthermore, when analyzing Fig. 10B, which shows the number of publications over five years, the Journal of Molecular Catalysis accounts for 60% of the published documents, followed by Process Biochemistry with 30% of the publications and Biochemical Engineering Journal with 10%. This visual representation highlights the interactions between countries regarding citations and mutual collaborations. It also highlights the importance of the links between different research centres and academic institutions, underscoring the crucial role of international cooperation in driving scientific and technological progress on a global scale.
In Fig. 11A, the top institutions are ranked by the number of published documents, most citations, and total link strength, highlighting those that have contributed the most to the research and their collaborative interactions. Aligarh Muslim University stands out, leading with 997 citations and 18 publications, reaffirming its dominance in research on magnetic nanomaterials for enzyme immobilization. The Superior Council for Scientific Investigations (CSIC) appears in second place with 841 citations and a higher number of 27 published documents, indicating increasing progress in research on this topic.
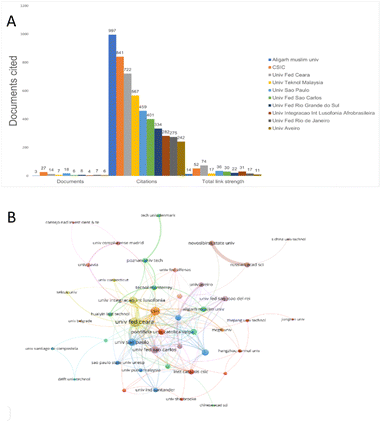 |
| Fig. 11 Bibliometric analysis of institutions. (A) Main institutions that published and cited. (B) Map of collaboration networks between institutions. | |
In third place is the Federal University of Ceará, in Brazil, with 722 citations and 14 published documents, highlighting Brazil's role in researching new technologies and advances. On the other hand, Fig. 11B provides a visualization map of the most important universities, identifying the clusters with the highest number of connections between universities. The Federal University of Ceará cluster (yellow) leads with 74 connections, followed by the CSIC cluster (orange) with 52 connections, and the University of São Paulo cluster (blue) with 36 connections. These results show that the total number of connections reinforces the strength of the overall connection of each institution. Of the 10 universities analyzed by the VOSviewer software, six are of Brazilian origin, highlighting Brazil as an important research center in this area.
7. Future trends (current challenges and prospects)
Magnetic nanoparticles are carriers with high potential for enzyme immobilization due to their easy separation and recovery from the reaction medium, large surface area, and high mass transfer capacity.518–521 However, the specific interactions between the carrier and the enzyme require optimization protocols to enhance the enzymes' catalytic activity, stability, and recyclability.522–524 Furthermore, the engineering and design of new magnetic nanomaterials with tailored structural properties and functionalities for specific applications while ensuring properties such as minimal toxicity, high biocompatibility, low environmental impact, and the selection of the appropriate immobilization method are critical considerations for industrial use.524–527
An example of a recently developed nanomaterial is cellulose nanocrystals, a versatile natural-based nanocarrier that has gained more attention in recent years due to its renewability, low cost, feasible synthesis and modification, high mechanical strength, and high stability against temperature and chemical compounds.528–534 Incorporating magnetic nanoparticles into the support matrix can facilitate the recovery and reuse of biocatalysts in practical applications.528–535 Another important aspect of using magnetic cellulose is that it allows for a single-step purification and immobilization of recombinant enzymes.
Enzymes fused with cellulose-binding domains.524–532 On the other hand, the diversity of biocatalysts available and the difficulty in scaling up the production process of this biocatalyst indicate the need for new strategies to incorporate these biotechnological units in the industrial sector.527,530,532 Dopamine is a functionalization reagent that gives excellent results for this type of nanomaterials.525,533–535 Dopamine is gaining attention for its versatility in anchoring various biologically active macromolecules such as antibodies, enzymes, DNA, and growth factors.525,534,536–539 The presence of catechol and amine in dopamine provides efficient conjugation of enzymes to various nanocarriers and does not require additional coupling reagents/complex linkers or organic solvents.525,533–535
In addition to the aspects already discussed, for the use of magnetic nanoparticles on an industrial scale, it is also necessary to have a complete understanding of the functionalization effects of the support, the surface density of enzymes, the binding sites between nanoparticles and enzymes, how immobilization chemistry can affect the activity and stability of the biocatalyst, the involvement of conformational changes in the immobilization process, and the design and development of immobilized enzyme-based bioreactors.522,540,541 From the topics discussed, it can be concluded that magnetic nanoparticles have a broad perspective in biocatalysis and several other fields.522,541–546
8. Conclusions
In summary, this review presents an overview of the development and construction of nanomaterial supports for possible applications in immobilising and stabilising enzymes. It is worth mentioning that enzymes immobilized on magnetic supports present advantages for commercial application due to their ease of separation and reuse, enabling greater scalability in the industrial sector. Furthermore, magnetic biocatalysts grouped by lipases have shown diverse applications and growing interest in the pharmaceutical and biofuel industries. It should also be noted that the bibliometric analysis explained that cooperation between countries and researchers is increasing on a large scale. Citations between journals and institutions generate high-impact articles and increase citations. The relevance of this topic and the high impact generated by the production of magnetic catalytic derivatives with biological content are increasingly apparent. However, magnetic biocatalysts still present some challenges that need to be overcome. Even though the advantages provided by enzyme immobilization add value, such as the possibility of recycling and improving their catalytic properties, the complexity of the synthesis and the different particularities of the lipase immobilization process still require more significant investments. Therefore, more research is needed to address these issues for long-term industrial applications of enzymes in addition to increased investment in this sector. To conclude, prospects are promising and have high industrial potential, allowing them to enhance existing processes further and produce new reaction routes that favour the sustainability and scalability of processes.
Author contributions
Writing—original draft preparation, A. L. G. C., R. B. R. V., G. F. M., J. E. S. S., T. G. R., F. T. T. C., K. S. M., A. L. B. O., J. Y. N. H. A., J. C. S. S.; writing—review and editing, A. L. G. C. and J. C. S. S. All authors have read and agreed to the published version of the manuscript.
Conflicts of interest
The authors declare no conflict of interest.
Acknowledgements
We gratefully acknowledge the following Brazilian Agencies for Scientific and Technological Development: Fundação Cearense de Apoio ao Desenvolvimento Científico e Tecnológico (FUNCAP), (PS1-0186-00216.01.00/21), (UNI-0210-00537.01.00/23), Conselho Nacional de Desenvolvimento Científico e Tecnológico (CNPq), (307454/2022-3), (440891/2020-5), and Coordenação de Aperfeiçoamento de Ensino Superior (CAPES) (finance code 001).
References
- T. Prozorov, D. A. Bazylinski, S. K. Mallapragada and R. Prozorov, Novel Magnetic Nanomaterials Inspired by Magnetotactic Bacteria: Topical Review, Mater. Sci. Eng., R, 2013, 74, 133–172, DOI:10.1016/j.mser.2013.04.002.
- J. Wang, Q. Zhang, X. Shao, J. Ma and G. Tian, Properties of Magnetic Carbon Nanomaterials and Application in Removal Organic Dyes, Chemosphere, 2018, 207, 377–384, DOI:10.1016/j.chemosphere.2018.05.109.
- R. F. L. Evans, W. J. Fan, P. Chureemart, T. A. Ostler, M. O. A. Ellis and R. W. Chantrell, Atomistic Spin Model Simulations of Magnetic Nanomaterials, J. Phys.: Condens.Matter, 2014, 26, 1–24, DOI:10.1088/0953-8984/26/10/103202.
- K. Zhu, Y. Ju, J. Xu, Z. Yang, S. Gao and Y. Hou, Magnetic Nanomaterials: Chemical Design, Synthesis, and Potential Applications, Acc. Chem. Res., 2018, 51, 404–413, DOI:10.1021/acs.accounts.7b00407.
- S. Liu, B. Yu, S. Wang, Y. Shen and H. Cong, Preparation, Surface Functionalization and Application of Fe3O4 Magnetic Nanoparticles, Adv. Colloid Interface Sci., 2020, 281, 102165, DOI:10.1016/j.cis.2020.102165.
- H. Nosrati, M. Salehiabar, S. Davaran, A. Ramazani, H. K. Manjili and H. Danafar, New Advances Strategies for Surface Functionalization of Iron Oxide Magnetic Nano Particles (IONPs), Res. Chem. Intermed., 2017, 43, 7423–7442, DOI:10.1007/s11164-017-3084-3.
- D. Fan, Q. Wang, T. Zhu, H. Wang, B. Liu, Y. Wang, Z. Liu, X. Liu, D. Fan and X. Wang, Recent Advances of Magnetic Nanomaterials in Bone Tissue Repair, Front. Chem., 2020, 8, 1–15, DOI:10.3389/fchem.2020.00745.
- X. Chen and L. Zhang, A Review on Micromixers Actuated with Magnetic Nanomaterials, Microchim. Acta, 2017, 184, 3639–3649, DOI:10.1007/s00604-017-2462-2.
- R. A. Bini, R. F. C. Marques, F. J. Santos, J. A. Chaker and M. Jafelicci, Synthesis and Functionalization of Magnetite Nanoparticles with Different Amino-Functional Alkoxysilanes, J. Magn. Magn. Mater., 2012, 324, 534–539, DOI:10.1016/j.jmmm.2011.08.035.
- W. Wu, Q. He and C. Jiang, Magnetic Iron Oxide Nanoparticles: Synthesis and Surface Functionalization Strategies, Nanoscale Res. Lett., 2008, 3, 397–415, DOI:10.1007/s11671-008-9174-9.
- K. Zhu, Y. Ju, J. Xu, Z. Yang, S. Gao and Y. Hou, Magnetic Nanomaterials: Chemical Design, Synthesis, and Potential Applications, Acc. Chem. Res., 2018, 51, 404–413, DOI:10.1021/acs.accounts.7b00407.
- G. Giakisikli and A. N. Anthemidis, Magnetic Materials as Sorbents for Metal/Metalloid Preconcentration and/or Separation. A Review, Anal. Chim. Acta, 2013, 789, 1–16, DOI:10.1016/j.aca.2013.04.021.
- C. F. G. C. Geraldes and S. Laurent, Classification and Basic Properties of Contrast Agents for Magnetic Resonance Imaging, Contrast Media Mol. Imaging, 2009, 4, 1–23, DOI:10.1002/cmmi.265.
- Y. Yang, Z. Fang, X. Chen, W. Zhang, Y. Xie, Y. Chen, Z. Liu and W. Yuan, An Overview of Pickering Emulsions: Solid-Particle Materials, Classification, Morphology, and Applications, Front. Pharmacol., 2017, 8, 1–20, DOI:10.3389/fphar.2017.00287.
- J. Kudr, Y. Haddad, L. Richtera, Z. Heger, M. Cernak, V. Adam and O. Zitka, Magnetic Nanoparticles: From Design and Synthesis to Real World Applications, Nanomaterials, 2017, 7, 1–29, DOI:10.3390/nano7090243.
- T. Prozorov, D. A. Bazylinski, S. K. Mallapragada and R. Prozorov, Novel Magnetic Nanomaterials
Inspired by Magnetotactic Bacteria: Topical Review, Mater. Sci. Eng., R, 2013, 74, 133–172, DOI:10.1016/j.mser.2013.04.002.
- D. Fan, Q. Wang, T. Zhu, H. Wang, B. Liu, Y. Wang, Z. Liu, X. Liu, D. Fan and X. Wang, Recent Advances of Magnetic Nanomaterials in Bone Tissue Repair, Front. Chem., 2020, 8, 1–15, DOI:10.3389/fchem.2020.00745.
- E. A. Périgo, J. Jacimovic, F. García Ferré and L. M. Scherf, Additive Manufacturing of Magnetic Materials, Addit. Manuf., 2019, 30, 100870, DOI:10.1016/j.addma.2019.100870.
- T. Plachy, O. Kratina and M. Sedlacik, Porous Magnetic Materials Based on EPDM Rubber Filled with Carbonyl Iron Particles, Compos. Struct., 2018, 192, 126–130, DOI:10.1016/j.compstruct.2018.02.095.
- Q. Jiang and Z. Zhong, Research and Development of Ce-Containing Nd2Fe14B-Type Alloys and Permanent Magnetic Materials, J. Mater. Sci. Technol., 2017, 33, 1087–1096, DOI:10.1016/j.jmst.2017.06.019.
- A. H. Lu, E. L. Salabas and F. Schüth, Magnetic Nanoparticles: Synthesis, Protection, Functionalization, and Application, Angew. Chem., Int. Ed., 2007, 46, 1222–1244, DOI:10.1002/anie.200602866.
- J. H. Lin, Z. H. Wu and W. L. Tseng, Extraction of Environmental Pollutants Using Magnetic Nanomaterials, Anal. Methods, 2010, 2, 1874–1879, 10.1039/c0ay00575d.
- J. Kudr, Y. Haddad, L. Richtera, Z. Heger, M. Cernak, V. Adam and O. Zitka, Magnetic Nanoparticles: From Design and Synthesis to Real World Applications, Nanomaterials, 2017, 7, 1–29, DOI:10.3390/nano7090243.
- G. Giakisikli and A. N. Anthemidis, Magnetic Materials as Sorbents for Metal/Metalloid Preconcentration and/or Separation. A Review, Anal. Chim. Acta, 2013, 789, 1–16, DOI:10.1016/j.aca.2013.04.021.
- W. Wu, Q. He and C. Jiang, Magnetic Iron Oxide Nanoparticles: Synthesis and Surface Functionalization Strategies, Nanoscale Res. Lett., 2008, 3, 397–415, DOI:10.1007/s11671-008-9174-9.
- S. Liu, B. Yu, S. Wang, Y. Shen and H. Cong, Preparation, Surface Functionalization and Application of Fe3O4 Magnetic Nanoparticles, Adv. Colloid Interface Sci., 2020, 281, 102165, DOI:10.1016/j.cis.2020.102165.
- H. Nosrati, M. Salehiabar, S. Davaran, A. Ramazani, H. K. Manjili and H. Danafar, New Advances Strategies for Surface Functionalization of Iron Oxide Magnetic Nano Particles (IONPs), Res. Chem. Intermed., 2017, 43, 7423–7442, DOI:10.1007/s11164-017-3084-3.
- R. A. Bini, R. F. C. Marques, F. J. Santos, J. A. Chaker and M. Jafelicci, Synthesis and Functionalization of Magnetite Nanoparticles with Different Amino-Functional Alkoxysilanes, J. Magn. Magn. Mater., 2012, 324, 534–539, DOI:10.1016/j.jmmm.2011.08.035.
- J. Wang, Q. Zhang, X. Shao, J. Ma and G. Tian, Properties of Magnetic Carbon Nanomaterials and Application in Removal Organic Dyes, Chemosphere, 2018, 207, 377–384, DOI:10.1016/j.chemosphere.2018.05.109.
- S. B. Somvanshi, P. B. Kharat, M. V. Khedkar and K. M. Jadhav, Hydrophobic to Hydrophilic Surface Transformation of Nano-Scale Zinc Ferrite via Oleic Acid Coating: Magnetic Hyperthermia Study towards Biomedical Applications, Ceram. Int., 2020, 46, 7642–7653, DOI:10.1016/j.ceramint.2019.11.265.
- X. Li, P. Yu, X. Niu, H. Yamaguchi and D. Li, Non-Contact Manipulation of Nonmagnetic Materials by Using a Uniform Magnetic Field: Experiment and Simulation, J. Magn. Magn. Mater., 2020, 497, 165957, DOI:10.1016/j.jmmm.2019.165957.
- B. Sai Ram, A. P. S. Baghel, S. V. Kulkarni, K. Chwastek and L. Daniel, A Hybrid Product-Multi-Scale Model for Magneto-Elastic Behavior of Soft Magnetic Materials, Phys. B, 2019, 571, 301–306, DOI:10.1016/j.physb.2019.06.069.
- H. Li, S. Ruan and Y. J. Zeng, Intrinsic Van Der Waals Magnetic Materials from Bulk to the 2D Limit: New Frontiers of Spintronics, Adv. Mater., 2019, 31, 1–34, DOI:10.1002/adma.201900065.
- R. F. L. Evans, W. J. Fan, P. Chureemart, T. A. Ostler, M. O. A. Ellis and R. W. Chantrell, Atomistic Spin Model Simulations of Magnetic Nanomaterials, J. Phys.: Condens.Matter, 2014, 26, 1–24, DOI:10.1088/0953-8984/26/10/103202.
- E. Yammine, L. Adumeau, M. Abboud, S. Mornet, M. Nakhl and E. Duguet, Towards Polymeric Nanoparticles with Multiple Magnetic Patches, Nanomaterials, 2021, 11, 1–15, DOI:10.3390/nano11010147.
- A. H. Lu, E. L. Salabas and F. Schüth, Magnetic Nanoparticles: Synthesis, Protection, Functionalization, and Application, Angew. Chem., Int. Ed., 2007, 46, 1222–1244, DOI:10.1002/anie.200602866.
- Y. Yang, Z. Fang, X. Chen, W. Zhang, Y. Xie, Y. Chen, Z. Liu and W. Yuan, An Overview of Pickering Emulsions: Solid-Particle Materials, Classification, Morphology, and Applications, Front. Pharmacol., 2017, 8, 1–20, DOI:10.3389/fphar.2017.00287.
- S. Čampelj, D. Makovec and M. Drofenik, Functionalization of Magnetic Nanoparticles with 3-Aminopropyl Silane, J. Magn. Magn. Mater., 2009, 321, 1346–1350, DOI:10.1016/j.jmmm.2009.02.036.
- Á. Ríos and M. Zougagh, Recent Advances in Magnetic Nanomaterials for Improving Analytical Processes, TrAC, Trends Anal. Chem., 2016, 84, 72–83, DOI:10.1016/j.trac.2016.03.001.
- W. Cai, X. Weng, W. Zhang and Z. Chen, Green Magnetic Nanomaterial as Antibiotic Release Vehicle: The Release of Pefloxacin and Ofloxacin, Mater. Sci. Eng. C, 2021, 118, 111439, DOI:10.1016/j.msec.2020.111439.
- R. Jurado and N. Gálvez, Apoferritin Amyloid-Fibril Directed the in Situ Assembly and/or Synthesis of Optical and Magnetic Nanoparticles, Nanomaterials, 2021, 11, 1–12, DOI:10.3390/nano11010146.
- E. Yammine, L. Adumeau, M. Abboud, S. Mornet, M. Nakhl and E. Duguet, Towards Polymeric Nanoparticles with Multiple Magnetic Patches, Nanomaterials, 2021, 11, 1–15, DOI:10.3390/nano11010147.
- M. S. A. Darwish, H. Kim, H. Lee, C. Ryu, J. Y. Lee and J. Yoon, Synthesis of Magnetic Ferrite Nanoparticles with High Hyperthermia Performance via a Controlled Co-Precipitation Method, Nanomaterials, 2019, 9, 1–21, DOI:10.3390/nano9081176.
- D. D. Andhare, S. R. Patade, J. S. Kounsalye and K. M. Jadhav, Effect of Zn Doping on Structural, Magnetic and Optical Properties of Cobalt Ferrite Nanoparticles Synthesized via. Co-Precipitation Method, Phys. B, 2020, 583, 412051, DOI:10.1016/j.physb.2020.412051.
- G. Vaidyanathan, S. Sendhilnathan and R. Arulmurugan, Structural and Magnetic Properties of Co1-XZnxFe2O4 Nanoparticles by Co-Precipitation Method, J. Magn. Magn. Mater., 2007, 313, 293–299, DOI:10.1016/j.jmmm.2007.01.010.
- N. Zhu, H. Ji, P. Yu, J. Niu, M. U. Farooq, M. W. Akram, I. O. Udego, H. Li and X. Niu, Surface Modification of Magnetic Iron Oxide Nanoparticles, Nanomaterials, 2018, 8, 1–27, DOI:10.3390/nano8100810.
- A. Sobczak-Kupiec, J. Venkatesan, A. Alhathal AlAnezi, D. Walczyk, A. Farooqi, D. Malina, S. H. Hosseini and B. Tyliszczak, Magnetic Nanomaterials and Sensors for Biological Detection, Nanomedicine, 2016, 12, 2459–2473, DOI:10.1016/j.nano.2016.07.003.
- Á. Ríos and M. Zougagh, Recent Advances in Magnetic Nanomaterials for Improving Analytical Processes, TrAC, Trends Anal. Chem., 2016, 84, 72–83, DOI:10.1016/j.trac.2016.03.001.
- A. Lassoued, M. S. Lassoued, B. Dkhil, S. Ammar and A. Gadri, Synthesis, Photoluminescence and Magnetic Properties of Iron Oxide (α-Fe2O3) Nanoparticles through Precipitation or Hydrothermal Methods, Phys. E, 2018, 101, 212–219, DOI:10.1016/j.physe.2018.04.009.
- A. Lassoued, M. S. Lassoued, B. Dkhil, S. Ammar and A. Gadri, Synthesis, Structural, Morphological, Optical and Magnetic Characterization of Iron Oxide (α-Fe2O3) Nanoparticles by Precipitation Method: Effect of Varying the Nature of Precursor, Phys. E, 2018, 97, 328–334, DOI:10.1016/j.physe.2017.12.004.
- Q. Zhang, X. Yang and J. Guan, Applications of Magnetic Nanomaterials in Heterogeneous Catalysis, ACS Appl. Nano Mater., 2019, 2, 4681–4697, DOI:10.1021/acsanm.9b00976.
- A. Lassoued, M. S. Lassoued, B. Dkhil, S. Ammar and A. Gadri, Synthesis, Photoluminescence and Magnetic Properties of Iron Oxide (α-Fe2O3) Nanoparticles through Precipitation or Hydrothermal Methods, Phys. E, 2018, 101, 212–219, DOI:10.1016/j.physe.2018.04.009.
- A. Lassoued, M. S. Lassoued, B. Dkhil, S. Ammar and A. Gadri, Synthesis, Structural, Morphological, Optical and Magnetic Characterization of Iron Oxide (α-Fe2O3) Nanoparticles by Precipitation Method: Effect of Varying the Nature of Precursor, Phys. E, 2018, 97, 328–334, DOI:10.1016/j.physe.2017.12.004.
- F. Maya, C. Palomino Cabello, R. M. Frizzarin, J. M. Estela, G. Turnes Palomino and V. Cerdà, Magnetic Solid-Phase Extraction Using Metal-Organic Frameworks (MOFs) and Their Derived Carbons, TrAC, Trends Anal. Chem., 2017, 90, 142–152, DOI:10.1016/j.trac.2017.03.004.
- I. Khmara, O. Strbak, V. Zavisova, M. Koneracka, M. Kubovcikova, I. Antal, V. Kavecansky, D. Lucanska, D. Dobrota and P. Kopcansky, Chitosan-Stabilized Iron Oxide Nanoparticles for Magnetic Resonance Imaging, J. Magn. Magn. Mater., 2019, 474, 319–325, DOI:10.1016/j.jmmm.2018.11.026.
- M. A. Almessiere, Y. Slimani, A. D. Korkmaz, N. Taskhandi, M. Sertkol, A. Baykal, S. E. Shirsath, İ. Ercan and B. Ozçelik, Sonochemical Synthesis of Eu3+ Substituted CoFe2O4 Nanoparticles and Their Structural, Optical and Magnetic Properties, Ultrason. Sonochem., 2019, 58, 104621, DOI:10.1016/j.ultsonch.2019.104621.
- J. Das, V. S. Moholkar and S. Chakma, Structural, Magnetic and Optical Properties of Sonochemically Synthesized Zr-Ferrite Nanoparticles, Powder Technol., 2018, 328, 1–6, DOI:10.1016/j.powtec.2017.11.057.
- J. Saffari, N. Mir, D. Ghanbari, K. Khandan-Barani, A. Hassanabadi and M. R. Hosseini-Tabatabaei, Sonochemical Synthesis of Fe3O4/ZnO Magnetic Nanocomposites and Their Application in Photo-Catalytic Degradation of Various Organic Dyes, J. Mater. Sci.: Mater. Electron., 2015, 26, 9591–9599, DOI:10.1007/s10854-015-3622-y.
- S. Majidi, F. Z. Sehrig, S. M. Farkhani, M. S. Goloujeh and A. Akbarzadeh, Current Methods for Synthesis of Magnetic Nanoparticles, Artif. Cells, Nanomed., Biotechnol., 2016, 44, 722–734, DOI:10.3109/21691401.2014.982802.
- X. Wang, P. Zhang, J. Gao, X. Chen and H. Yang, Facile Synthesis and Magnetic Properties of Fe3C/C Nanoparticles via a Sol-Gel Process, Dyes Pigm., 2015, 112, 305–310, DOI:10.1016/j.dyepig.2014.07.021.
- M. Manzoor, A. Rafiq, M. Ikram, M. Nafees and S. Ali, Structural, Optical, and Magnetic Study of Ni-Doped TiO2 Nanoparticles Synthesized by Sol–Gel Method, Int. Nano Lett., 2018, 8, 1–8, DOI:10.1007/s40089-018-0225-7.
- S. Aliramaji, A. Zamanian and Z. Sohrabijam, Characterization and Synthesis of Magnetite Nanoparticles by Innovative Sonochemical Method, Procedia Mater. Sci., 2015, 11, 265–269, DOI:10.1016/j.mspro.2015.11.022.
- A. K. Johnson, A. M. Zawadzka, L. A. Deobald, R. L. Crawford and A. J. Paszczynski, Novel Method for Immobilization of Enzymes to Magnetic Nanoparticles, J. Nanopart. Res., 2008, 10, 1009–1025, DOI:10.1007/s11051-007-9332-5.
- M. N. Gupta, M. Kaloti, M. Kapoor and K. Solanki, Nanomaterials as Matrices for Enzyme Immobilization, Artif. Cells, Blood Substitutes, Biotechnol., 2011, 39, 98–109, DOI:10.3109/10731199.2010.516259.
- M. Bilal and H. M. N. Iqbal, Chemical, Physical, and Biological Coordination: An Interplay between Materials and Enzymes as Potential Platforms for Immobilization, Coord. Chem. Rev., 2019, 388, 1–23, DOI:10.1016/j.ccr.2019.02.024.
- Q. Zhang, X. Yang and J. Guan, Applications of Magnetic Nanomaterials in Heterogeneous Catalysis, ACS Appl. Nano Mater., 2019, 2, 4681–4697, DOI:10.1021/acsanm.9b00976.
- L. Zhong, Y. Feng, G. Wang, Z. Wang, M. Bilal, H. Lv, S. Jia and J. Cui, Production and Use of Immobilized Lipases in/on Nanomaterials: A Review from the Waste to Biodiesel Production, Int. J. Biol. Macromol., 2020, 152, 207–222, DOI:10.1016/j.ijbiomac.2020.02.258.
- J. Xu, J. Sun, Y. Wang, J. Sheng, F. Wang and M. Sun, Application of Iron Magnetic Nanoparticles in Protein Immobilization, Molecules, 2014, 19, 11465–11486, DOI:10.3390/molecules190811465.
- A. K. Johnson, A. M. Zawadzka, L. A. Deobald, R. L. Crawford and A. J. Paszczynski, Novel Method for Immobilization of Enzymes to Magnetic Nanoparticles, J. Nanopart. Res., 2008, 10, 1009–1025, DOI:10.1007/s11051-007-9332-5.
- M. N. Gupta, M. Kaloti, M. Kapoor and K. Solanki, Nanomaterials as Matrices for Enzyme Immobilization, Artif. Cells, Blood Substitutes, Biotechnol., 2011, 39, 98–109, DOI:10.3109/10731199.2010.516259.
- S. K. S. Patel, R. K. Gupta, S. Y. Kim, I. W. Kim, V. C. Kalia and J. K. Lee, Rhus Vernicifera Laccase Immobilization on Magnetic Nanoparticles to Improve Stability and Its Potential Application in Bisphenol A Degradation, Indian J. Microbiol., 2021, 61, 45–54, DOI:10.1007/s12088-020-00912-4.
- J. Xu, J. Sun, Y. Wang, J. Sheng, F. Wang and M. Sun, Application of Iron Magnetic Nanoparticles in Protein Immobilization, Molecules, 2014, 19, 11465–11486, DOI:10.3390/molecules190811465.
- M. Bilal and H. M. N. Iqbal, Chemical, Physical, and Biological Coordination: An Interplay between Materials and Enzymes as Potential Platforms for Immobilization, Coord. Chem. Rev., 2019, 388, 1–23, DOI:10.1016/j.ccr.2019.02.024.
- L. Zhong, Y. Feng, G. Wang, Z. Wang, M. Bilal, H. Lv, S. Jia and J. Cui, Production and Use of Immobilized Lipases in/on Nanomaterials: A Review from the Waste to Biodiesel Production, Int. J. Biol. Macromol., 2020, 152, 207–222, DOI:10.1016/j.ijbiomac.2020.02.258.
- M. Bilal, Y. Zhao, T. Rasheed and H. M. N. Iqbal, Magnetic Nanoparticles as Versatile Carriers for Enzymes Immobilization: A Review, Int. J. Biol. Macromol., 2018, 120, 2530–2544, DOI:10.1016/j.ijbiomac.2018.09.025.
- S. S. Nadar and V. K. Rathod, Magnetic-Metal Organic Framework (Magnetic-MOF): A Novel Platform for Enzyme Immobilization and Nanozyme Applications, Int. J. Biol. Macromol., 2018, 120, 2293–2302, DOI:10.1016/j.ijbiomac.2018.08.126.
- G. Orfanakis, M. Patila, A. V. Catzikonstantinou, K. M. Lyra, A. Kouloumpis, K. Spyrou, P. Katapodis, A. Paipetis, P. Rudolf and D. Gournis, et al., Hybrid Nanomaterials of Magnetic Iron Nanoparticles and Graphene Oxide as Matrices for the Immobilization of β-Glucosidase: Synthesis, Characterization, and Biocatalytic Properties, Front. Mater., 2018, 5, 1–11, DOI:10.3389/fmats.2018.00025.
- F. Esmi, T. Nematian, Z. Salehi, A.
A. Khodadadi and A. K. Dalai, Amine and Aldehyde Functionalized Mesoporous Silica on Magnetic Nanoparticles for Enhanced Lipase Immobilization, Biodiesel Production, and Facile Separation, Fuel, 2021, 291, 120126, DOI:10.1016/j.fuel.2021.120126.
- J. Long, X. Li, X. Liu, Z. Jin, Z. Xie, X. Xu and C. Lu, Preparation of streptavidin-coated magnetic nanoparticles for specific immobilization of enzymes with high activity and enhanced stability, Ind. Eng. Chem. Res., 2021, 60, 1542–1552, DOI:10.1021/acs.iecr.0c03281.
- K. Khoshnevisan, E. Poorakbar, H. Baharifar and M. Barkhi, Recent Advances of Cellulase Immobilization onto Magnetic Nanoparticles: An Update Review, Magnetochemistry, 2019, 5, 36, DOI:10.3390/magnetochemistry5020036.
- S. K. S. Patel, R. K. Gupta, S. Y. Kim, I. W. Kim, V. C. Kalia and J. K. Lee, Rhus Vernicifera Laccase Immobilization on Magnetic Nanoparticles to Improve Stability and Its Potential Application in Bisphenol A Degradation, Indian J. Microbiol., 2021, 61, 45–54, DOI:10.1007/s12088-020-00912-4.
- M. S. Jabir, U. M. Nayef and W. K. A. Kadhim, Polyethylene Glycol-Functionalized Magnetic (Fe3O4) Nanoparticles: A Novel DNA-Mediated Antibacterial Agent, Nano Biomed. Eng., 2019, 11, 18–27, DOI:10.5101/nbe.v11i1.p18-27.
- M. Jouyandeh, M. R. Ganjali, J. A. Ali, V. Akbari, Z. Karami, M. Aghazadeh, P. Zarrintaj and M. R. Saeb, Curing Epoxy with Polyethylene Glycol (PEG) Surface-Functionalized GdxFe3-XO4 Magnetic Nanoparticles, Prog. Org. Coat., 2019, 137, 105283, DOI:10.1016/j.porgcoat.2019.105283.
- S. O. Aisida, I. Ahmad and F. I. Ezema, Effect of Calcination on the Microstructural and Magnetic Properties of PVA, PVP and PEG Assisted Zinc Ferrite Nanoparticles, Phys. B, 2020, 579, 411907, DOI:10.1016/j.physb.2019.411907.
- M. Jouyandeh, M. R. Ganjali, J. A. Ali, M. Aghazadeh, S. M. R. Paran, G. Naderi, M. R. Saeb and S. Thomas, Curing Epoxy with Polyvinylpyrrolidone (PVP) Surface-Functionalized ZnxFe3-XO4 Magnetic Nanoparticles, Prog. Org. Coat., 2019, 136, 105227, DOI:10.1016/j.porgcoat.2019.105227.
- M. Jouyandeh, M. R. Ganjali, J. A. Ali, M. Aghazadeh, M. R. Saeb and S. S. Ray, Curing Epoxy with Polyvinylpyrrolidone (PVP) Surface-Functionalized NixFe3-XO4 Magnetic Nanoparticles, Prog. Org. Coat., 2019, 136, 105259, DOI:10.1016/j.porgcoat.2019.105259.
- S. O. Aisida, I. Ahmad and F. I. Ezema, Effect of Calcination on the Microstructural and Magnetic Properties of PVA, PVP and PEG Assisted Zinc Ferrite Nanoparticles, Phys. B, 2020, 579, 411907, DOI:10.1016/j.physb.2019.411907.
- M. Jouyandeh, M. R. Ganjali, J. A. Ali, M. Aghazadeh, S. M. R. Paran, G. Naderi, M. R. Saeb and S. Thomas, Curing Epoxy with Polyvinylpyrrolidone (PVP) Surface-Functionalized ZnxFe3-XO4 Magnetic Nanoparticles, Prog. Org. Coat., 2019, 136, 105227, DOI:10.1016/j.porgcoat.2019.105227.
- M. Jouyandeh, M. R. Ganjali, J. A. Ali, M. Aghazadeh, M. R. Saeb and S. S. Ray, Curing Epoxy with Polyvinylpyrrolidone (PVP) Surface-Functionalized NixFe3-XO4 Magnetic Nanoparticles, Prog. Org. Coat., 2019, 136, 105259, DOI:10.1016/j.porgcoat.2019.105259.
- H. P. Cong, J. J. He, Y. Lu and S. H. Yu, Water-Soluble Magnetic-Functionalized Reduced Graphene Oxide Sheets: In Situ Synthesis and Magnetic Resonance Imaging Applications, Small, 2010, 6, 169–173, DOI:10.1002/smll.200901360.
- A. Walcarius, S. D. Minteer, J. Wang, Y. Lin and A. Merkoçi, Nanomaterials for Bio-Functionalized Electrodes: Recent Trends, J. Mater. Chem. B, 2013, 1, 4878–4908, 10.1039/c3tb20881h.
- S. Bagheri and N. M. Julkapli, Modified Iron Oxide Nanomaterials: Functionalization and Application, J. Magn. Magn. Mater., 2016, 416, 117–133, DOI:10.1016/j.jmmm.2016.05.042.
- Q. Tang, Z. Zhou and Z. Chen, Graphene-Related Nanomaterials: Tuning Properties by Functionalization, Nanoscale, 2013, 5, 4541–4583, 10.1039/c3nr33218g.
- B. Hu, J. Pan, H. L. Yu, J. W. Liu and J. H. Xu, Immobilization of Serratia Marcescens Lipase onto Amino-Functionalized Magnetic Nanoparticles for Repeated Use in Enzymatic Synthesis of Diltiazem Intermediate, Process Biochem., 2009, 44, 1019–1024, DOI:10.1016/j.procbio.2009.05.001.
- S. Leitner, C. Solans, M. J. García-Celma, G. Morral-Ruíz, P. Melgar-Lesmes and G. Calderó, Ethylcellulose Nanoparticles Prepared from Nano-Emulsion Templates as New Folate Binding Haemocompatible Platforms, Mater. Sci. Eng. C, 2020, 120, 1–45, DOI:10.1016/j.msec.2020.111682.
- S. O. Aisida, P. A. Akpa, I. Ahmad, T. kai Zhao, M. Maaza and F. I. Ezema, Bio-Inspired Encapsulation and Functionalization of Iron Oxide Nanoparticles for Biomedical Applications, Eur. Polym. J., 2020, 122, 109371, DOI:10.1016/j.eurpolymj.2019.109371.
- B. Thangaraj and P. R. Solomon, Immobilization of Lipases – A Review. Part II: Carrier Materials, ChemBioEng Rev., 2019, 6, 167–194, DOI:10.1002/cben.201900017.
- F. Alnadari, Y. Xue, L. Zhou, Y. S. Hamed, M. Taha and M. F. Foda, Immobilization of β-Glucosidase from Thermatoga Maritima on Chitin-Functionalized Magnetic Nanoparticle via a Novel Thermostable Chitin-Binding Domain, Sci. Rep., 2020, 10, 1–12, DOI:10.1038/s41598-019-57165-5.
- X. Qiu, S. Wang, S. Miao, H. Suo, H. Xu and Y. Hu, Co-Immobilization of Laccase and ABTS onto Amino-Functionalized Ionic Liquid-Modified Magnetic Chitosan Nanoparticles for Pollutants Removal, J. Hazard. Mater., 2021, 401, 1–35, DOI:10.1016/j.jhazmat.2020.123353.
- A. Soozanipour, A. Taheri-Kafrani, M. Barkhori and M. Nasrollahzadeh, Preparation of a Stable and Robust Nanobiocatalyst by Efficiently Immobilizing of Pectinase onto Cyanuric Chloride-Functionalized Chitosan Grafted Magnetic Nanoparticles, J. Colloid Interface Sci., 2019, 536, 261–270, DOI:10.1016/j.jcis.2018.10.053.
- G. Y. Li, Z. De Zhou, Y. J. Li, K. L. Huang and M. Zhong, Surface Functionalization of Chitosan-Coated Magnetic Nanoparticles for Covalent Immobilization of Yeast Alcohol Dehydrogenase from Saccharomyces Cerevisiae, J. Magn. Magn. Mater., 2010, 322, 3862–3868, DOI:10.1016/j.jmmm.2010.08.008.
- G. Cheng, J. Xing, Z. Pi, S. Liu, Z. Liu and F. Song, α-Glucosidase Immobilization on Functionalized Fe 3 O 4 Magnetic Nanoparticles for Screening of Enzyme Inhibitors, Chin. Chem. Lett., 2019, 30, 656–659, DOI:10.1016/j.cclet.2018.12.003.
- M. L. Verma, S. Kumar, A. Das, J. S. Randhawa and M. Chamundeeswari, Chitin and Chitosan-Based Support Materials for Enzyme Immobilization and Biotechnological Applications, Environ. Chem. Lett., 2020, 18, 315–323, DOI:10.1007/s10311-019-00942-5.
- M. I. A. Abdel Maksoud, A. M. Elgarahy, C. Farrell, A. H. Al-Muhtaseb, D. W. Rooney and A. I. Osman, Insight on Water Remediation Application Using Magnetic Nanomaterials and Biosorbents, Coord. Chem. Rev., 2020, 403, 213096, DOI:10.1016/j.ccr.2019.213096.
- Z. Cai, Y. Sun, W. Liu, F. Pan, P. Sun and J. Fu, An Overview of Nanomaterials Applied for Removing Dyes from Wastewater, Environ. Sci. Pollut. Res., 2017, 24, 15882–15904, DOI:10.1007/s11356-017-9003-8.
- B. Thangaraj and P. R. Solomon, Immobilization of Lipases – A Review. Part II: Carrier Materials, ChemBioEng Rev., 2019, 6, 167–194, DOI:10.1002/cben.201900017.
- W. Shuai, R. K. Das, M. Naghdi, S. K. Brar and M. Verma, A Review on the Important Aspects of Lipase Immobilization on Nanomaterials, Biotechnol. Appl. Biochem., 2017, 64, 496–508, DOI:10.1002/bab.1515.
- E. P. Cipolatti, A. Valério, R. O. Henriques, D. E. Moritz, J. L. Ninow, D. M. G. Freire, E. A. Manoel, R. Fernandez-Lafuente and D. De Oliveira, Nanomaterials for Biocatalyst Immobilization-State of the Art and Future Trends, RSC Adv., 2016, 6, 104675–104692, 10.1039/c6ra22047a.
- W. Shuai, R. K. Das, M. Naghdi, S. K. Brar and M. Verma, A Review on the Important Aspects of Lipase Immobilization on Nanomaterials, Biotechnol. Appl. Biochem., 2017, 64, 496–508, DOI:10.1002/bab.1515.
- M. Cao, Z. Li, J. Wang, W. Ge, T. Yue, R. Li, V. L. Colvin and W. W. Yu, Food Related Applications of Magnetic Iron Oxide Nanoparticles: Enzyme Immobilization, Protein Purification, and Food Analysis, Trends Food Sci. Technol., 2012, 27, 47–56, DOI:10.1016/j.tifs.2012.04.003.
- K. Khoshnevisan, A. K. Bordbar, D. Zare, D. Davoodi, M. Noruzi, M. Barkhi and M. Tabatabaei, Immobilization of Cellulase Enzyme on Superparamagnetic Nanoparticles and Determination of Its Activity and Stability, Chem. Eng. J., 2011, 171, 669–673, DOI:10.1016/j.cej.2011.04.039.
- M. Cao, Z. Li, J. Wang, W. Ge, T. Yue, R. Li, V. L. Colvin and W. W. Yu, Food Related Applications of Magnetic Iron Oxide Nanoparticles: Enzyme Immobilization, Protein Purification, and Food Analysis, Trends Food Sci. Technol., 2012, 27, 47–56, DOI:10.1016/j.tifs.2012.04.003.
- K. Khoshnevisan, A. K. Bordbar, D. Zare, D. Davoodi, M. Noruzi, M. Barkhi and M. Tabatabaei, Immobilization of Cellulase Enzyme on Superparamagnetic Nanoparticles and Determination of Its Activity and Stability, Chem. Eng. J., 2011, 171, 669–673, DOI:10.1016/j.cej.2011.04.039.
- A. Soozanipour, A. Taheri-Kafrani and A. Landarani Isfahani, Covalent Attachment of Xylanase on Functionalized Magnetic Nanoparticles and Determination of Its Activity and Stability, Chem. Eng. J., 2015, 270, 235–243, DOI:10.1016/j.cej.2015.02.032.
- K. Abdollahi, F. Yazdani and R. Panahi, Covalent Immobilization of Tyrosinase onto Cyanuric Chloride Crosslinked Amine-Functionalized Superparamagnetic Nanoparticles: Synthesis and Characterization of the Recyclable Nanobiocatalyst, Int. J. Biol. Macromol., 2017, 94, 396–405, DOI:10.1016/j.ijbiomac.2016.10.058.
- H. Torabizadeh and A. Mahmoudi, Inulin Hydrolysis by Inulinase Immobilized Covalently on Magnetic Nanoparticles Prepared with Wheat Gluten Hydrolysates, Biotechnol. Rep., 2018, 17, 97–103, DOI:10.1016/j.btre.2018.02.004.
- W. Sheng, Y. Xi, L. Zhang, T. Ye and X. Zhao, Enhanced Activity and Stability of Papain by Covalent Immobilization on Porous Magnetic Nanoparticles, Int. J. Biol. Macromol., 2018, 114, 143–148, DOI:10.1016/j.ijbiomac.2018.03.088.
- M. R. Mehrasbi, J. Mohammadi, M. Peyda and M. Mohammadi, Covalent Immobilization of Candida Antarctica Lipase on Core-Shell Magnetic Nanoparticles for Production of Biodiesel from Waste Cooking Oil, Renewable Energy, 2017, 101, 593–602, DOI:10.1016/j.renene.2016.09.022.
- M. Ziegler-Borowska, D. Chelminiak-Dudkiewicz, T. Siódmiak, A. Sikora, K. Wegrzynowska-Drzymalska, J. Skopinska-Wisniewska, H. Kaczmarek and M. P. Marszałł, Chitosan–Collagen Coated Magnetic Nanoparticles for Lipase Immobilization—New Type of “Enzyme Friendly” Polymer Shell Crosslinking with Squaric Acid, Catalysts, 2017, 7, 26, DOI:10.3390/CATAL7010026.
- D. M. Liu, J. Chen and Y. P. Shi, Tyrosinase Immobilization on Aminated Magnetic Nanoparticles by Physical Adsorption Combined with Covalent Crosslinking with Improved Catalytic Activity, Reusability and Storage Stability, Anal. Chim. Acta, 2018, 1006, 90–98, DOI:10.1016/J.ACA.2017.12.022.
- T. Iype, J. Thomas, S. Mohan, K. K. Johnson, L. E. George, L. A. Ambattu, A. Bhati, K. Ailsworth, B. Menon and S. M. Rayabandla, et al., A Novel Method for Immobilization of Proteins via Entrapment of
Magnetic Nanoparticles through Epoxy Cross-Linking, Anal. Biochem., 2017, 519, 42–50, DOI:10.1016/J.AB.2016.12.007.
- H. Torabizadeh and A. Mahmoudi, Inulin Hydrolysis by Inulinase Immobilized Covalently on Magnetic Nanoparticles Prepared with Wheat Gluten Hydrolysates, Biotechnol. Rep., 2018, 17, 97–103, DOI:10.1016/J.BTRE.2018.02.004.
- M. R. Mehrasbi, J. Mohammadi, M. Peyda and M. Mohammadi, Covalent Immobilization of Candida Antarctica Lipase on Core-Shell Magnetic Nanoparticles for Production of Biodiesel from Waste Cooking Oil, Renewable Energy, 2017, 101, 593–602, DOI:10.1016/J.RENENE.2016.09.022.
- W. Sheng, Y. Xi, L. Zhang, T. Ye and X. Zhao, Enhanced Activity and Stability of Papain by Covalent Immobilization on Porous Magnetic Nanoparticles, Int. J. Biol. Macromol., 2018, 114, 143–148, DOI:10.1016/J.IJBIOMAC.2018.03.088.
- K. Abdollahi, F. Yazdani and R. Panahi, Covalent Immobilization of Tyrosinase onto Cyanuric Chloride Crosslinked Amine-Functionalized Superparamagnetic Nanoparticles: Synthesis and Characterization of the Recyclable Nanobiocatalyst, Int. J. Biol. Macromol., 2017, 94, 396–405, DOI:10.1016/J.IJBIOMAC.2016.10.058.
- J. Long, X. Li, X. Zhan, X. Xu, Y. Tian, Z. Xie and Z. Jin, Sol–Gel Encapsulation of Pullulanase in the Presence of Hybrid Magnetic (Fe3O4–Chitosan) Nanoparticles Improves Thermal and Operational Stability, Bioprocess Biosyst. Eng., 2017, 40, 821–831, DOI:10.1007/S00449-017-1747-5/FIGURES/7.
- J. S. Lima, P. H. H. Araújo, C. Sayer, A. A. U. Souza, A. C. Viegas and D. de Oliveira, Cellulase Immobilization on Magnetic Nanoparticles Encapsulated in Polymer Nanospheres, Bioprocess Biosyst. Eng., 2017, 40, 511–518, DOI:10.1007/S00449-016-1716-4/FIGURES/10.
- B. B. Tikhonov, E. M. Sulman, P. Y. Stadol'nikova, A. M. Sulman, E. P. Golikova, A. I. Sidorov and V. G. Matveeva, Immobilized Enzymes from the Class of Oxidoreductases in Technological Processes: A Review, Catal. Ind., 2019, 11, 251–263, DOI:10.1134/S2070050419030115.
- A. Sulman, V. G. Matveeva, E. P. Golikova, O. V. Grebennikova, N. V. Lakina, V. Y. Doluda, A. Y. Karpenkov and E. M. Sulman, Oxidoreductase Immobilization on Magnetic Nanoparticles, Chem. Eng. Trans., 2019, 74, 487–492, DOI:10.3303/CET1974082.
- J. Zdarta, A. S. Meyer, T. Jesionowski and M. Pinelo, A General Overview of Support Materials for Enzyme Immobilization: Characteristics, Properties, Practical Utility, Catalysts, 2018, 8, 1–27, DOI:10.3390/catal8020092.
- C. G. C. M. Netto, H. E. Toma and L. H. Andrade, Superparamagnetic Nanoparticles as Versatile Carriers and Supporting Materials for Enzymes, J. Mol. Catal. B: Enzym., 2013, 85–86, 71–92, DOI:10.1016/j.molcatb.2012.08.010.
- A. Kumari, P. Kaila, P. Tiwari, V. Singh, S. Kaul, N. Singhal and P. Guptasarma, Multiple Thermostable Enzyme Hydrolases on Magnetic Nanoparticles: An Immobilized Enzyme-Mediated Approach to Saccharification through Simultaneous Xylanase, Cellulase and Amylolytic Glucanotransferase Action, Int. J. Biol. Macromol., 2018, 120, 1650–1658, DOI:10.1016/j.ijbiomac.2018.09.106.
- R. Khaksarinejad, A. Mohsenifar, T. Rahmani-Cherati, R. Karami and M. Tabatabaei, An Organophosphorus Hydrolase-Based Biosensor for Direct Detection of Paraoxon Using Silica-Coated Magnetic Nanoparticles, Appl. Biochem. Biotechnol., 2015, 176, 359–371, DOI:10.1007/s12010-015-1579-1.
- O. A. Journal, Biointerface Res. Appl. Chem., 2020, 10, 5556–5563 Search PubMed.
- M. de Sousa, B. Silva Gurgel, B. C. Pessela and L. R. B. Gonçalves, Preparation of CLEAs and Magnetic CLEAs of a Recombinant L-Arabinose Isomerase for D-Tagatose Synthesis, Enzyme Microb. Technol., 2020, 138, 109566, DOI:10.1016/j.enzmictec.2020.109566.
- S. Chakraborty, T. Jagan Mohan Rao and A. Goyal, Immobilization of Recombinant Pectate Lyase from Clostridium Thermocellum ATCC-27405 on Magnetic Nanoparticles for Bioscouring of Cotton Fabric, Biotechnol. Prog., 2017, 33, 236–244, DOI:10.1002/btpr.2379.
- B. Tural, S. Tural, E. Ertaş, I. Yalinkiliç and A. S. Demir, Purification and Covalent Immobilization of Benzaldehyde Lyase with Heterofunctional Chelate-Epoxy Modified Magnetic Nanoparticles and Its Carboligation Reactivity, J. Mol. Catal. B: Enzym., 2013, 95, 41–47, DOI:10.1016/j.molcatb.2013.05.023.
- M. de Sousa, B. Silva Gurgel, B. C. Pessela and L. R. B. Gonçalves, Preparation of CLEAs and Magnetic CLEAs of a Recombinant L-Arabinose Isomerase for D-Tagatose Synthesis, Enzyme Microb. Technol., 2020, 138, 109566, DOI:10.1016/j.enzmictec.2020.109566.
- J. R. Guimarães, R. de Lima Camargo Giordano, R. Fernandez-Lafuente and P. W. Tardioli, Evaluation of Strategies to Produce Highly Porous Cross-Linked Aggregates of Porcine Pancreas Lipase with Magnetic Properties, Molecules, 2018, 23, 13–16, DOI:10.3390/molecules23112993.
- L. P. Miranda, J. R. Guimarães, R. C. Giordano, R. Fernandez-Lafuente and P. W. Tardioli, Composites of Crosslinked Aggregates of Eversa® Transform and Magnetic Nanoparticles. Performance in the Ethanolysis of Soybean Oil, Catalysts, 2020, 10, 9–11, DOI:10.3390/catal10080817.
- X. Yang, Y. Chen, S. Yao, J. Qian, H. Guo and X. Cai, Preparation of Immobilized Lipase on Magnetic Nanoparticles Dialdehyde Starch, Carbohydr. Polym., 2019, 218, 324–332, DOI:10.1016/j.carbpol.2019.05.012.
- R. R. C. Monteiro, D. M. A. Neto, P. B. A. Fechine, A. A. S. Lopes, L. R. B. Gonçalves, J. C. S. Dos Santos, M. C. M. de Souza and R. Fernandez-Lafuente, Ethyl Butyrate Synthesis Catalyzed by Lipases a and b from Candida Antarctica Immobilized onto Magnetic Nanoparticles. Improvement of Biocatalysts’ Performance under Ultrasonic Irradiation, Int. J. Mol. Sci., 2019, 20, 1–21, DOI:10.3390/ijms20225807.
- R. R. C. Monteiro, P. J. M. Lima, B. B. Pinheiro, T. M. Freire, L. M. U. Dutra, P. B. A. Fechine, L. R. B. Gonçalves, M. C. M. de Souza, J. C. S. Dos Santos and R. Fernandez-Lafuente, Immobilization of Lipase a from Candida Antarctica onto Chitosan-Coated Magnetic Nanoparticles, Int. J. Mol. Sci., 2019, 20, 4018, DOI:10.3390/ijms20164018.
- H. Vaghari, H. Jafarizadeh-Malmiri, M. Mohammadlou, A. Berenjian, N. Anarjan, N. Jafari and S. Nasiri, Application of Magnetic Nanoparticles in Smart Enzyme Immobilization, Biotechnol. Lett., 2016, 38, 223–233, DOI:10.1007/s10529-015-1977-z.
- S. H. Huang and R. S. Juang, Biochemical and Biomedical Applications of Multifunctional Magnetic Nanoparticles: A Review, J. Nanopart. Res., 2011, 13, 4411–4430, DOI:10.1007/s11051-011-0551-4.
- H. H. P. Yiu and M. A. Keane, Enzyme-Magnetic Nanoparticle Hybrids: New Effective Catalysts for the Production of High Value Chemicals, J. Chem. Technol. Biotechnol., 2012, 87, 583–594, DOI:10.1002/jctb.3735.
- C. C. S. Fortes, A. L. Daniel-da-Silva, A. M. R. B. Xavier and A. P. M. Tavares, Optimization of Enzyme Immobilization on Functionalized Magnetic Nanoparticles for Laccase Biocatalytic Reactions, Chem. Eng. Process., 2017, 117, 1–8, DOI:10.1016/j.cep.2017.03.009.
- R. Fernandez-Lafuente, Editorial for Special Issue: Enzyme Immobilization and Its Applications, Molecules, 2019, 24, 6–9, DOI:10.3390/molecules24244619.
- M. Fuentes, C. Mateo, A. Rodriguez, M. Casqueiro, J. C. Tercero, H. H. Riese, R. Fernández-Lafuente and J. M. Guisán, Detecting Minimal Traces of DNA Using DNA Covalently Attached to Superparamagnetic Nanoparticles and Direct PCR-ELISA, Biosens. Bioelectron., 2006, 21, 1574–1580, DOI:10.1016/j.bios.2005.07.017.
- L. Betancor, M. Fuentes, G. Dellamora-Ortiz, F. López-Gallego, A. Hidalgo, N. Alonso-Morales, C. Mateo, J. M. Guisán and R. Fernández-Lafuente, Dextran Aldehyde Coating of Glucose Oxidase Immobilized on Magnetic Nanoparticles Prevents Its Inactivation by Gas Bubbles, J.
Mol. Catal. B: Enzym., 2005, 32, 97–101, DOI:10.1016/j.molcatb.2004.11.003.
- F. Kazenwadel, H. Wagner, B. E. Rapp and M. Franzreb, Optimization of Enzyme Immobilization on Magnetic Microparticles Using 1-Ethyl-3-(3-Dimethylaminopropyl)Carbodiimide (EDC) as a Crosslinking Agent, Anal. Methods, 2015, 7, 10291–10298, 10.1039/c5ay02670a.
- G. Khoobbakht, K. Kheiralipour, W. Yuan, M. R. Seifi and M. Karimi, Desirability Function Approach for Optimization of Enzymatic Transesterification Catalyzed by Lipase Immobilized on Mesoporous Magnetic Nanoparticles, Renewable Energy, 2020, 158, 253–262, DOI:10.1016/j.renene.2020.05.087.
- X. Zhao, F. Qi, C. Yuan, W. Du and D. Liu, Lipase-Catalyzed Process for Biodiesel Production: Enzyme Immobilization, Process Simulation and Optimization, Renewable Sustainable Energy Rev., 2015, 44, 182–197, DOI:10.1016/j.rser.2014.12.021.
- C. C. S. Fortes, A. L. Daniel-da-Silva, A. M. R. B. Xavier and A. P. M. Tavares, Optimization of Enzyme Immobilization on Functionalized Magnetic Nanoparticles for Laccase Biocatalytic Reactions, Chem. Eng. Process., 2017, 117, 1–8, DOI:10.1016/j.cep.2017.03.009.
- H. Zhang, T. Liu, Y. Zhu, L. Hong, T. Li, X. Wang and Y. Fu, Lipases Immobilized on the Modified Polyporous Magnetic Cellulose Support as an Efficient and Recyclable Catalyst for Biodiesel Production from Yellow Horn Seed Oil, Renewable Energy, 2020, 145, 1246–1254, DOI:10.1016/j.renene.2019.06.031.
- S. Shanmugam, S. Krishnaswamy, R. Chandrababu, U. Veerabagu, A. Pugazhendhi and T. Mathimani, Optimal Immobilization of Trichoderma Asperellum Laccase on Polymer Coated Fe3O4@SiO2 Nanoparticles for Enhanced Biohydrogen Production from Delignified Lignocellulosic Biomass, Fuel, 2020, 273, 117777, DOI:10.1016/j.fuel.2020.117777.
- K. da S. Moreira, A. L. B. de Oliveira, L. S. de M. Júnior, R. R. C. Monteiro, T. N. da Rocha, F. L. Menezes, L. M. U. D. Fechine, J. C. Denardin, S. Michea and R. M. Freire, et al., Lipase From Rhizomucor Miehei Immobilized on Magnetic Nanoparticles: Performance in Fatty Acid Ethyl Ester (FAEE) Optimized Production by the Taguchi Method, Front. Bioeng. Biotechnol., 2020, 8, 1–17, DOI:10.3389/fbioe.2020.00693.
- M. A. A. Abdella, G. M. El-Sherbiny, A. R. El-Shamy, S. M. M. Atalla and S. A. Ahmed, Statistical Optimization of Chemical Modification of Chitosan-Magnetic Nano-Particles Beads to Promote Bacillus Subtilis MK1 α-Amylase Immobilization and Its Application, Bull. Natl. Res. Cent., 2020, 44, 1–13, DOI:10.1186/s42269-020-00301-3.
- F. Kazenwadel, H. Wagner, B. E. Rapp and M. Franzreb, Optimization of Enzyme Immobilization on Magnetic Microparticles Using 1-Ethyl-3-(3-Dimethylaminopropyl)Carbodiimide (EDC) as a Crosslinking Agent, Anal. Methods, 2015, 7, 10291–10298, 10.1039/c5ay02670a.
- H. Zhang, T. Liu, Y. Zhu, L. Hong, T. Li, X. Wang and Y. Fu, Lipases Immobilized on the Modified Polyporous Magnetic Cellulose Support as an Efficient and Recyclable Catalyst for Biodiesel Production from Yellow Horn Seed Oil, Renewable Energy, 2020, 145, 1246–1254, DOI:10.1016/j.renene.2019.06.031.
- G. Khoobbakht, K. Kheiralipour, W. Yuan, M. R. Seifi and M. Karimi, Desirability Function Approach for Optimization of Enzymatic Transesterification Catalyzed by Lipase Immobilized on Mesoporous Magnetic Nanoparticles, Renewable Energy, 2020, 158, 253–262, DOI:10.1016/j.renene.2020.05.087.
- S. Shanmugam, S. Krishnaswamy, R. Chandrababu, U. Veerabagu, A. Pugazhendhi and T. Mathimani, Optimal Immobilization of Trichoderma Asperellum Laccase on Polymer Coated Fe3O4@SiO2 Nanoparticles for Enhanced Biohydrogen Production from Delignified Lignocellulosic Biomass, Fuel, 2020, 273, 117777, DOI:10.1016/j.fuel.2020.117777.
- E. Quayson, J. Amoah, S. Hama, A. Kondo and C. Ogino, Immobilized Lipases for Biodiesel Production: Current and Future Greening Opportunities, Renewable Sustainable Energy Rev., 2020, 134, 110355, DOI:10.1016/j.rser.2020.110355.
- A. R. Ismail and K. H. Baek, Lipase Immobilization with Support Materials, Preparation Techniques, and Applications: Present and Future Aspects, Int. J. Biol. Macromol., 2020, 163, 1624–1639, DOI:10.1016/j.ijbiomac.2020.09.021.
- J. Liu, R. T. Ma and Y. P. Shi, “Recent Advances on Support Materials for Lipase Immobilization and Applicability as Biocatalysts in Inhibitors Screening Methods”-A Review, Anal. Chim. Acta, 2020, 1101, 9–22, DOI:10.1016/j.aca.2019.11.073.
- A. R. Ismail and K. H. Baek, Lipase Immobilization with Support Materials, Preparation Techniques, and Applications: Present and Future Aspects, Int. J. Biol. Macromol., 2020, 163, 1624–1639, DOI:10.1016/j.ijbiomac.2020.09.021.
- E. Quayson, J. Amoah, S. Hama, A. Kondo and C. Ogino, Immobilized Lipases for Biodiesel Production: Current and Future Greening Opportunities, Renewable Sustainable Energy Rev., 2020, 134, 110355, DOI:10.1016/j.rser.2020.110355.
- P. Sirajudheen, P. Karthikeyan, K. Ramkumar, P. Nisheetha and S. Meenakshi, Magnetic Carbon-Biomass from the Seeds of Moringa Oleifera@MnFe2O4 Composite as an Effective and Recyclable Adsorbent for the Removal of Organic Pollutants from Water, J. Mol. Liq., 2020, 114829, DOI:10.1016/j.molliq.2020.114829.
- G. L. Lima, R. W. L. Oliveira, R. M. de Jesus Neto, A. M. de S. Gomes, R. A. Fiuza Junior, H. M. C. Andrade and A. J. S. Mascarenhas, Single Step Synthesis of Magnetic Materials Derived from Biomass Residues, Waste Biomass Valorization, 2020, 12, 1039–1050, DOI:10.1007/s12649-020-01003-7.
- A. Wang, P. Sudarsanam, Y. Xu, H. Zhang, H. Li and S. Yang, Functionalized Magnetic Nanosized Materials for Efficient Biodiesel Synthesis: Via Acid-Base/Enzyme Catalysis, Green Chem., 2020, 22, 2977–3012, 10.1039/d0gc00924e.
- R. Nithya, A. Thirunavukkarasu, A. Bose and S. Raja, Crystal, C. V Magnetic Materials and Magnetic Separation of Dyes from Aqueous Solutions : A Review, Environ. Chem. Lett., 2021, 19, 1275–1294, DOI:10.1007/s10311-020-01149-9.
- J. Liu, R. T. Ma and Y. P. Shi, “Recent Advances on Support Materials for Lipase Immobilization and Applicability as Biocatalysts in Inhibitors Screening Methods”-A Review, Anal. Chim. Acta, 2020, 1101, 9–22, DOI:10.1016/j.aca.2019.11.073.
- S. Liang, X. L. Wu, J. Xiong, M. H. Zong and W. Y. Lou, Metal-Organic Frameworks as Novel Matrices for Efficient Enzyme Immobilization: An Update Review, Coord. Chem. Rev., 2020, 406, 213149, DOI:10.1016/j.ccr.2019.213149.
- L. H. Nguyen, N. H. Nam, L. T. Tam, D. Van Tuan, N. X. Truong, N. Van Quynh, P. Thi Hong Tuyet, H. P. Thu, D. H. Manh and P. T. Phong, et al., Effect of Gd Substitution on Structure, Optical and Magnetic Properties, and Heating Efficiency of Fe3O4 Nanoparticles for Magnetic Hyperthermia Applications, J. Alloys Compd., 2023, 968, 172205, DOI:10.1016/j.jallcom.2023.172205.
- T. Dippong, Innovative Nanomaterial Properties and Applications in Chemistry , Physics , Medicine , or Environment, Nanomaterials, 2024, 14, 145 CrossRef CAS PubMed.
- O. Awogbemi and D. V. Kallon, Von Recent Advances in the Application of Nanomaterials for Improved Biodiesel, Biogas, Biohydrogen, and Bioethanol Production, Fuel, 2024, 358, 130261, DOI:10.1016/j.fuel.2023.130261.
- M. Ahmadi, A. Ghoorchian, K. Dashtian, M. Kamalabadi, T. Madrakian and A. Afkhami, Application of Magnetic Nanomaterials in Electroanalytical Methods: A Review, Talanta, 2021, 225, 121974, DOI:10.1016/j.talanta.2020.121974.
- N. Mosleh, M. Najmi, E. Parandi, H. Rashidi Nodeh, Y. Vasseghian and S. Rezania, Magnetic Sporopollenin Supported Polyaniline Developed for Removal of Lead Ions from Wastewater: Kinetic, Isotherm and Thermodynamic Studies, Chemosphere, 2022, 300, 134461, DOI:10.1016/j.chemosphere.2022.134461.
- H. Vaghari, H. Jafarizadeh-Malmiri, M. Mohammadlou, A. Berenjian, N. Anarjan, N. Jafari and S. Nasiri, Application of Magnetic Nanoparticles in Smart Enzyme Immobilization, Biotechnol. Lett., 2016, 38, 223–233, DOI:10.1007/s10529-015-1977-z.
- S. Behzad and R. Chegel, Tailoring Thermoelectric Properties through Carbon Doping and Magnetic Field Variation: A Comparative Study in 2D h-BN and h-SiC, Chin. J. Phys., 2024, 87, 398–414, DOI:10.1016/j.cjph.2023.12.003.
- A. Pusta, M. Tertis, I. Crăciunescu, R. Turcu, S. Mirel and C. Cristea, Recent Advances in the Development of Drug Delivery Applications of Magnetic Nanomaterials, Pharmaceutics, 2023, 15, 1–37, DOI:10.3390/pharmaceutics15071872.
- L. Parra-Arroyo, R. B. González-González, R. A. Chavez-Santoscoy, E. A. Flores-Contreras, R. Parra-Saldívar, E. M. M. Martínez and H. M. N. Iqbal, Magnetic Nanomaterials Assisted Nanobiocatalysis to Abate Groundwater Pollution, MethodsX, 2023, 10, 102161, DOI:10.1016/j.mex.2023.102161.
- R. L. F. Melo, M. B. Sales, V. de Castro Bizerra, P. G. de Sousa Junior, A. L. G. Cavalcante, T. M. Freire, F. S. Neto, M. Bilal, T. Jesionowski and J. M. Soares, et al., Recent Applications and Future Prospects of Magnetic Biocatalysts, Int. J. Biol. Macromol., 2023, 253, 1–26, DOI:10.1016/j.ijbiomac.2023.126709.
- K. Zhu, Y. Ju, J. Xu, Z. Yang, S. Gao and Y. Hou, Magnetic Nanomaterials: Chemical Design, Synthesis, and Potential Applications, Acc. Chem. Res., 2018, 51, 404–413, DOI:10.1021/acs.accounts.7b00407.
- E. F. Bôa Morte, D. S. Marum, E. B. Saitovitch, M. Alzamora, S. N. Monteiro and R. J. Sanchez Rodriguez, Modified Magnetite Nanoparticle as Biocatalytic Support for Magnetically Stabilized Fluidized Bed Reactors, J. Mater. Res. Technol., 2021, 14, 1112–1125, DOI:10.1016/j.jmrt.2021.06.105.
- P. D. Patil, R. K. Kelkar, N. P. Patil, P. V. Pise, S. P. Patil, A. S. Patil, N. S. Kulkarni, M. S. Tiwari, A. N. Phirke and S. S. Nadar, Magnetic Nanoflowers: A Hybrid Platform for Enzyme Immobilization, Crit. Rev. Biotechnol., 2023, 1–22, DOI:10.1080/07388551.2023.2230518.
- M. A. Mariño, S. Fulaz and L. Tasic, Magnetic Nanomaterials as Biocatalyst Carriers for Biomass Processing: Immobilization Strategies, Reusability, and Applications, Magnetochemistry, 2021, 7, 1–22, DOI:10.3390/magnetochemistry7100133.
- H. Maftoon, A. Taravati and F. Tohidi, Immobilization of Laccase on Carboxyl-Functionalized Chitosan-Coated Magnetic Nanoparticles with Improved Stability and Reusability, Monatsh. Chem., 2023, 154, 279–291, DOI:10.1007/s00706-022-03029-0.
- M. Henrique da Silva Cavalcanti, L. Bueno Alves, A. Duarte, A. Aguiar Mendes, J. Maurício Schneedorf Ferreira da Silva, N. José Freitas da Silveira, M. Tsuyama Escote and L. Sindra Virtuoso, Immobilization of Thermomyces Lanuginosus Lipase via Ionic Adsorption on Superparamagnetic Iron Oxide Nanoparticles: Facile Synthesis and Improved Catalytic Performance, Chem. Eng. J., 2022, 431, 1–13, DOI:10.1016/j.cej.2021.134128.
- I. Armenia, M. V. Grazú Bonavia, L. De Matteis, P. Ivanchenko, G. Martra, R. Gornati, J. M. de la Fuente and G. Bernardini, Enzyme Activation by Alternating Magnetic Field: Importance of the Bioconjugation Methodology, J. Colloid Interface Sci., 2019, 537, 615–628, DOI:10.1016/j.jcis.2018.11.058.
- N. Y. Elamin, A. Modwi, W. Abd El-Fattah and A. Rajeh, Synthesis and Structural of Fe3O4 Magnetic Nanoparticles and Its Effect on the Structural Optical, and Magnetic Properties of Novel Poly(Methyl Methacrylate)/Polyaniline Composite for Electromagnetic and Optical Applications, Opt. Mater., 2023, 135, 113323, DOI:10.1016/j.optmat.2022.113323.
- S. S. Abouelkheir, H. A. H. Ibrahim and E. A. Beltagy, Functionalized Maghemite Superparamagnetic Iron Oxide Nanoparticles (γ-Fe2O3-SPIONs)-Amylase Enzyme Hybrid in Biofuel Production, Sci. Rep., 2023, 13, 1–14, DOI:10.1038/s41598-023-37826-2.
- E. Parandi, M. Safaripour, N. Mosleh, M. Saidi, H. Rashidi Nodeh, B. Oryani and S. Rezania, Lipase Enzyme Immobilized over Magnetic Titanium Graphene Oxide as Catalyst for Biodiesel Synthesis from Waste Cooking Oil, Biomass Bioenergy, 2023, 173, 106794, DOI:10.1016/j.biombioe.2023.106794.
- D. E. Navarro-López, A. R. Bautista-Ayala, M. F. Rosales-De la Cruz, S. Martínez-Beltrán, D. E. Rojas-Torres, A. Sanchez-Martinez, O. Ceballos-Sanchez, J. A. Jáuregui-Jáuregui, L. M. Lozano and M. Sepúlveda-Villegas, et al., Nanocatalytic Performance of Pectinase Immobilized over in Situ Prepared Magnetic Nanoparticles, Heliyon, 2023, 9, 1–11, DOI:10.1016/j.heliyon.2023.e19021.
- D. Yu, Z. Li, X. Zhou, W. Wang, L. Wang, T. Liu and J. Du, Study on the Modification of Magnetic Graphene Oxide and the Effect of Immobilized Lipase, Int. J. Biol. Macromol., 2022, 216, 498–509, DOI:10.1016/j.ijbiomac.2022.06.203.
- M. Sadeghi, Z. Moghimifar and H. Javadian, Fe3O4@SiO2 Nanocomposite Immobilized with Cellulase Enzyme: Stability Determination and Biological Activity, Chem. Phys. Lett., 2023, 811, 140161, DOI:10.1016/j.cplett.2022.140161.
- J. A. A. Abdullah, Á. Díaz-García, J. Y. Law, A. Romero, V. Franco and A. Guerrero, Sustainable Nanomagnetism: Investigating the Influence of Green Synthesis and PH on Iron Oxide Nanoparticles for Enhanced Biomedical Applications, Polymers, 2023, 15, 1–25, DOI:10.3390/polym15183850.
- D. Egea-Benavente, C. Díaz-Ufano, Á. Gallo-Cordova, F. J. Palomares, J. L. Cuya Huaman, D. F. Barber, M. Morales, P. del and J. Balachandran, Cubic Mesocrystal Magnetic Iron Oxide Nanoparticle Formation by Oriented Aggregation of Cubes in Organic Media: A Rational Design to Enhance the Magnetic Hyperthermia Efficiency, ACS Appl. Mater. Interfaces, 2023, 15, 32162–32176, DOI:10.1021/acsami.3c03254.
- P. P. Falciglia, E. Gagliano, P. Scandura, C. Bianco, T. Tosco, R. Sethi, G. Varvaro, E. Agostinelli, C. Bongiorno and A. Russo, et al., Physico-Magnetic Properties and Dynamics of Magnetite (Fe3O4) Nanoparticles (MNPs) under the Effect of Permanent Magnetic Fields in Contaminated Water Treatment Applications, Sep. Purif. Technol., 2022, 296, 121342, DOI:10.1016/j.seppur.2022.121342.
- M. Bilal, E. U. Rashid, J. Munawar, H. M. N. Iqbal, J. Cui, J. Zdarta, S. S. Ashraf and T. Jesionowski, Magnetic Metal-Organic Frameworks Immobilized Enzyme-Based Nano-Biocatalytic Systems for Sustainable Biotechnology, Int. J. Biol. Macromol., 2023, 237, 123968, DOI:10.1016/j.ijbiomac.2023.123968.
- M. Bilal, Y. Zhao, T. Rasheed and H. M. N. Iqbal, Magnetic Nanoparticles as Versatile Carriers for Enzymes Immobilization: A Review, Int. J. Biol. Macromol., 2018, 120, 2530–2544, DOI:10.1016/j.ijbiomac.2018.09.025.
- S. Bedanta, O. Petracic and W. Kleemann, in Supermagnetism, 2015, pp. 1–83 Search PubMed.
- T. Neuberger, B. Schöpf, H. Hofmann, M. Hofmann and B. von Rechenberg, Superparamagnetic Nanoparticles for Biomedical Applications: Possibilities and Limitations of a New Drug Delivery System, J. Magn. Magn. Mater., 2005, 293, 483–496, DOI:10.1016/j.jmmm.2005.01.064.
- G. C. Papaefthymiou, Nanoparticle Magnetism, Nano Today, 2009, 4, 438–447, DOI:10.1016/j.nantod.2009.08.006.
- D. Peddis, P. E. Jönsson, S. Laureti and G. Varvaro, in Magnetic Interactions, 2014, pp. 129–188 Search PubMed.
- O. Petracic, Superparamagnetic Nanoparticle Ensembles, Superlattices Microstruct., 2010, 47, 569–578, DOI:10.1016/j.spmi.2010.01.009.
- A. Ali, H. Zafar, M. Zia, I. ul Haq, A. R. Phull, J. S. Ali and A. Hussain, Synthesis, Characterization, Applications, and Challenges of Iron Oxide Nanoparticles, Nanotechnol., Sci. Appl., 2016, 9, 49–67, DOI:10.2147/NSA.S99986.
- V. Raviraj, B. T. T. Pham, B. J. Kim, N. T. H. Pham, L. F. Kok, N. Painter, N. C. Delic, S. K. Jones, B. S. Hawkett and J. G. Lyons, Non-Invasive Transdermal Delivery of Chemotherapeutic Molecules in Vivo Using Superparamagnetic Iron Oxide Nanoparticles, Cancer Nanotechnol., 2021, 12, 6, DOI:10.1186/s12645-021-00079-7.
- P. Carter, B. Narasimhan and Q. Wang, Biocompatible Nanoparticles and Vesicular Systems in Transdermal Drug Delivery for Various Skin Diseases, Int. J. Pharm., 2019, 555, 49–62, DOI:10.1016/j.ijpharm.2018.11.032.
- F. S. Hasany, I. Ahmed, J. Rajan and A. Rehman, Systematic Review of the Preparation Techniques of Iron Oxide Magnetic Nanoparticles, Nanosci. Nanotechnol., 2013, 2(26), 148–158, DOI:10.5923/j.nn.20120206.01.
- B. Palmer and L. DeLouise, Nanoparticle-Enabled Transdermal Drug Delivery Systems for Enhanced Dose Control and Tissue Targeting, Molecules, 2016, 21, 1719, DOI:10.3390/molecules21121719.
- M. Wang, S. K. Marepally, P. K. Vemula and C. Xu, Inorganic nanoparticles for transdermal drug delivery and topical application, in Nanoscience in Dermatology, Elsevier, 2016, pp. 57–72 Search PubMed.
- T. Dippong, Innovative Nanomaterial Properties and Applications in Chemistry, Physics, Medicine, or Environment, Nanomaterials, 2024, 14, 145, DOI:10.3390/nano14020145.
- P. F. Rezaei, S. Ghanbari and G. Mahdavinia, Purification and Immobilization of Exo-Polygalacturonase Using Nanomagnetic Beads, Process Biochem., 2023, 132, 180–190, DOI:10.1016/j.procbio.2023.06.018.
- A. G. Niculescu, C. Chircov and A. M. Grumezescu, Magnetite Nanoparticles: Synthesis Methods – A Comparative Review, Methods, 2022, 199, 16–27, DOI:10.1016/j.ymeth.2021.04.018.
- X. Batlle, C. Moya, M. Escoda-Torroella, Ò. Iglesias, A. Fraile Rodríguez and A. Labarta, Magnetic Nanoparticles: From the Nanostructure to the Physical Properties, J. Magn. Magn. Mater., 2022, 543, 1–60, DOI:10.1016/j.jmmm.2021.168594.
- S. wei Ge, X. yu Wang, T. Chang, B. Chen, P. Hu, F. F. Yang, Q. gao Cao, F. Yang, L. Kang and K. S. Wang, Fe3O4 Nanoparticles Synthesized by One-Step Reduction with Nanoscale Size-Dependent Magnetic Properties, J. Sol-Gel Sci. Technol., 2023, 105, 98–105, DOI:10.1007/s10971-022-05962-2.
- A. Al-Anazi, Iron-Based Magnetic Nanomaterials in Environmental and Energy Applications: A Short Review, Curr. Opin. Chem. Eng., 2022, 36, 100794, DOI:10.1016/j.coche.2022.100794.
- H. Al-Madhagi, V. Yazbik, W. Abdelwahed and L. Alchab, Magnetite Nanoparticle Co-Precipitation Synthesis, Characterization, and Applications: Mini Review, Bionanoscience, 2023, 13, 853–859, DOI:10.1007/s12668-023-01113-1.
- S. Dai, M. Li, X. Wang, H. Zhu, Y. Zhao and Z. Wu, Fabrication and Magnetic Property of Novel (Co,Zn,Fe,Mn,Ni)3O4 High-Entropy Spinel Oxide, J. Magn. Magn. Mater., 2021, 536, 168123, DOI:10.1016/j.jmmm.2021.168123.
- R. Abedini‐nassab, M. Pouryosef Miandoab and M. Şaşmaz, Microfluidic Synthesis, Control, and Sensing of Magnetic Nanoparticles: A Review, Micromachines, 2021, 12, 1–34, DOI:10.3390/mi12070768.
- A. Setia, A. K. Mehata, S. Vikas, A. K. Malik, M. K. Viswanadh and M. S. Muthu, Theranostic Magnetic Nanoparticles: Synthesis, Properties, Toxicity, and Emerging Trends for Biomedical Applications, J. Drug Delivery Sci. Technol., 2023, 81, 104295, DOI:10.1016/j.jddst.2023.104295.
- A. Ali, T. Shah, R. Ullah, P. Zhou, M. Guo, M. Ovais, Z. Tan and Y. K. Rui, Review on Recent Progress in Magnetic Nanoparticles: Synthesis, Characterization, and Diverse Applications, Front. Chem., 2021, 9, 1–25, DOI:10.3389/fchem.2021.629054.
- S. I. Hamdallah, R. Zoqlam, P. Erfle, M. Blyth, A. M. Alkilany, A. Dietzel and S. Qi, Microfluidics for Pharmaceutical Nanoparticle Fabrication: The Truth and the Myth, Int. J. Pharm., 2020, 584, 1–16, DOI:10.1016/j.ijpharm.2020.119408.
- J. Wang, Q. Han, K. Wang, S. Li, W. Luo, Q. Liang, J. Zhong and M. Ding, Recent Advances in Development of Functional Magnetic Adsorbents for Selective Separation of Proteins/Peptides, Talanta, 2023, 253, 123919, DOI:10.1016/j.talanta.2022.123919.
- J. M. M. Silva, P. E. Feuser, R. Cercená, M. Peterson and A. G. Dal-Bó, Obtention of Magnetite Nanoparticles via the Hydrothermal Method and Effect of Synthesis Parameters, J. Magn. Magn. Mater., 2023, 580(27), 170925, DOI:10.1016/j.jmmm.2023.170925.
- W. Li, F. Xiao, X. Bai and H. Xu, Magnetic Nanoparticles for Food Hazard Factors Sensing: Synthesis, Modification and Application, Chem. Eng. J., 2023, 465, 142816, DOI:10.1016/j.cej.2023.142816.
- D. Morán, G. Gutiérrez, R. Mendoza, M. Rayner, C. Blanco-López and M. Matos, Synthesis of Controlled-Size Starch Nanoparticles and Superparamagnetic Starch Nanocomposites by Microemulsion Method, Carbohydr. Polym., 2023, 299, 120–223, DOI:10.1016/j.carbpol.2022.120223.
- B. Rezaei, P. Yari, S. M. Sanders, H. Wang, V. K. Chugh, S. Liang, S. Mostufa, K. Xu, J. P. Wang and J. Gómez-Pastora, et al., Magnetic Nanoparticles: A Review on Synthesis, Characterization, Functionalization, and Biomedical Applications, Small, 2023, 2304848, 1–34, DOI:10.1002/smll.202304848.
- W. M. Alamier, N. Hasan, M. S. Nawaz, K. S. Ismail, M. Shkir, M. A. Malik and M. D. Y. Oteef, Biosynthesis of NiFe2O4nanoparticles Using Murayya Koenigii for Photocatalytic Dye Degradation and Antibacterial Application, J. Mater. Res. Technol., 2023, 22, 1331–1348, DOI:10.1016/j.jmrt.2022.11.181.
- D. D. Suppiah, N. M. Julkapli, S. Sagadevan and M. R. Johan, Eco-Friendly Green Synthesis Approach and Evaluation of Environmental and Biological Applications of Iron Oxide Nanoparticles, Inorg. Chem. Commun., 2023, 152, 110700, DOI:10.1016/j.inoche.2023.110700.
- M. J. Jacinto, V. C. Silva, D. M. S. Valladão and R. S. Souto, Biosynthesis of Magnetic Iron Oxide Nanoparticles: A Review, Biotechnol. Lett., 2021, 43, 1–12, DOI:10.1007/s10529-020-03047-0.
- P. R. Yaashikaa and P. S. Kumar, Fabrication and Characterization of Magnetic Nanomaterials for the Removal of Toxic Pollutants from Water Environment: A Review, Chemosphere, 2022, 303, 135067, DOI:10.1016/j.chemosphere.2022.135067.
- N. Karami, A. Mohammadpour, M. R. Samaei, A. M. Amani, M. Dehghani, R. S. Varma and J. N. Sahu, Green Synthesis of Sustainable Magnetic Nanoparticles Fe3O4 and Fe3O4-Chitosan Derived from Prosopis Farcta Biomass Extract and Their Performance in the Sorption of Lead(II), Int. J. Biol. Macromol., 2024, 254, 127663, DOI:10.1016/j.ijbiomac.2023.127663.
- T. Tarhan, G. Dik, A. Ulu, B. Tural, S. Tural and B. Ateş, Newly Synthesized Multifunctional Biopolymer Coated Magnetic Core/Shell Fe3O4@Au Nanoparticles for Evaluation of L-Asparaginase Immobilization, Top. Catal., 2023, 66, 577–591, DOI:10.1007/s11244-022-01742-y.
- R. Maurya, U. Ali, S. Kaul, R. Bhaiyya, R. P. Singh and K. Mazumder, Immobilization of α-Transglucosidase on Silica-Coated Magnetic Nanoparticles and Its Application for Production of Isomaltooligosaccharide from the Potato Peel, Sci. Rep., 2023, 13, 1–16, DOI:10.1038/s41598-023-38266-8.
- M. D. Nguyen, H. V. Tran, S. Xu and T. R. Lee, Fe3O4 Nanoparticles: Structures, Synthesis, Magnetic Properties, Surface Functionalization, and Emerging Applications, Appl. Sci., 2021, 11, 1–23, DOI:10.3390/app112311301.
- B. Wareppam, E. Kuzmann, V. K. Garg and L. H. Singh, Mössbauer Spectroscopic Investigations on Iron Oxides and Modified Nanostructures: A Review, J. Mater. Res., 2023, 38, 937–957, DOI:10.1557/s43578-022-00665-4.
- T. Girardet, S. Diliberto, C. Carteret, F. Cleymand and S. Fleutot, Determination of the Percentage of Magnetite in Iron Oxide Nanoparticles: A Comparison between Mössbauer Spectroscopy and Raman Spectroscopy, Solid State Sci., 2023, 143, 107–116, DOI:10.1016/j.solidstatesciences.2023.107258.
- G. Sarojini, P. Kannan, N. Rajamohan and M. Rajasimman, Bio-Fabrication of Porous Magnetic Chitosan/Fe3O4 Nanocomposite Using Azolla Pinnata for Removal of Chromium – Parametric Effects, Surface Characterization and Kinetics, Environ. Res., 2023, 218, 114822, DOI:10.1016/j.envres.2022.114822.
- S. Kharazmi and A. Taheri-Kafrani, Bi-Enzymatic Nanobiocatalyst Based on Immobilization of Xylanase and Pectinase onto Functionalized Magnetic Nanoparticles for Efficient Fruit Juice Clarification, Lwt, 2023, 183, 114914, DOI:10.1016/j.lwt.2023.114914.
- P. Agnihotri and V. N. Lad, Controlling the Interface in Microfluidic Flow by Varying Orientations of Microchannels with Reference to the Magnetic Field, J. Braz. Soc. Mech. Sci. Eng., 2023, 45, 1–11, DOI:10.1007/s40430-023-04167-0.
- R. Wohlgemuth, J. Littlechild, D. Monti, K. Schnorr, T. van Rossum, B. Siebers, P. Menzel, I. v. Kublanov, A. G. Rike and G. Skretas, et al., Discovering Novel Hydrolases from Hot Environments, Biotechnol. Adv., 2018, 36, 2077–2100, DOI:10.1016/J.BIOTECHADV.2018.09.004.
- M. Sharifi, M. J. Sohrabi, S. H. Hosseinali, A. Hasan, P. H. Kani, A. J. Talaei, A. Y. Karim, N. M. Q. Nanakali, A. Salihi and F. M. Aziz, et al., Enzyme Immobilization onto the Nanomaterials: Application in Enzyme Stability and Prodrug-Activated Cancer Therapy, Int. J. Biol. Macromol., 2020, 143, 665–676, DOI:10.1016/J.IJBIOMAC.2019.12.064.
- Z. Ashkan, R. Hemmati, A. Homaei, A. Dinari, M. Jamlidoost and A. Tashakor, Immobilization of Enzymes on Nanoinorganic Support Materials: An Update, Int. J. Biol. Macromol., 2021, 168, 708–721, DOI:10.1016/J.IJBIOMAC.2020.11.127.
- E. Zanuso, H. A. Ruiz, L. Domingues and J. A. Teixeira, Magnetic Nanoparticles as Support for Cellulase Immobilization Strategy for Enzymatic Hydrolysis Using Hydrothermally Pretreated Corn Cob Biomass, BioEnergy Res., 2022, 1, 1–12, DOI:10.1007/S12155-021-10384-Z/TABLES/2.
- M. Bilal, Y. Zhao, T. Rasheed and H. M. N. Iqbal, Magnetic Nanoparticles as Versatile Carriers for Enzymes Immobilization: A Review, Int. J. Biol. Macromol., 2018, 120, 2530–2544, DOI:10.1016/J.IJBIOMAC.2018.09.025.
- Z. Ashkan, R. Hemmati, A. Homaei, A. Dinari, M. Jamlidoost and A. Tashakor, Immobilization of Enzymes on Nanoinorganic Support Materials: An Update, Int. J. Biol. Macromol., 2021, 168, 708–721, DOI:10.1016/j.ijbiomac.2020.11.127.
- Y. A. Rodríguez-Restrepo and C. E. Orrego, Immobilization of Enzymes and Cells on Lignocellulosic Materials, Environ. Chem. Lett., 2020, 18, 787–806, DOI:10.1007/s10311-020-00988-w.
- J. Boudrant, J. M. Woodley and R. Fernandez-Lafuente, Parameters Necessary to Define an Immobilized Enzyme Preparation, Process Biochem., 2020, 90, 66–80, DOI:10.1016/j.procbio.2019.11.026.
- R. A. Wahab, N. Elias, F. Abdullah and S. K. Ghoshal, On the Taught New Tricks of Enzymes Immobilization: An All-Inclusive Overview, React. Funct. Polym., 2020, 152, 104613, DOI:10.1016/j.reactfunctpolym.2020.104613.
- F. Shakerian, J. Zhao and S.-P. Li, Recent Development in the Application of Immobilized Oxidative Enzymes for Bioremediation of Hazardous Micropollutants – A Review, Chemosphere, 2020, 239, 124716, DOI:10.1016/j.chemosphere.2019.124716.
- J. M. Guisan, F. López-Gallego, J. M. Bolívar, M. J. Ravier and L. G. Fernandéz, The science of enzyme immobilization, in Immobilization of Enzymes and Cells: Methods and Protocols, Springer US, New York, NY, 2020, pp. 1–26 Search PubMed.
- F. Shakerian, J. Zhao and S.-P. Li, Recent Development in the Application of Immobilized Oxidative Enzymes for Bioremediation of Hazardous Micropollutants – A Review, Chemosphere, 2020, 239, 124716, DOI:10.1016/j.chemosphere.2019.124716.
- J. M. Guisan, F. López-Gallego, J. M. Bolívar, M. J. Ravier and L. G. Fernandéz, The science of enzyme immobilization, in Immobilization of Enzymes and Cells: Methods and Protocols, Springer US, New York, NY, 2020, pp. 1–26 Search PubMed.
- Z. Ashkan, R. Hemmati, A. Homaei, A. Dinari, M. Jamlidoost and A. Tashakor, Immobilization of Enzymes on Nanoinorganic Support Materials: An Update, Int. J. Biol. Macromol., 2021, 168, 708–721, DOI:10.1016/j.ijbiomac.2020.11.127.
- Y. A. Rodríguez-Restrepo and C. E. Orrego, Immobilization of Enzymes and Cells on Lignocellulosic Materials, Environ. Chem. Lett., 2020, 18, 787–806, DOI:10.1007/s10311-020-00988-w.
- T. Heinks, N. Montua, M. Teune, J. Liedtke, M. Höhne, U. T. Bornscheuer and G. von Mollard, Comparison of Four Immobilization Methods for Different Transaminases, Catalysts, 2023, 13, 300–307, DOI:10.3390/catal13020300.
- J. Ahlawat, M. Sharma and C. S. Pundir, An Amperometric Acetylcholine Biosensor Based on Co-Immobilization of Enzyme Nanoparticles onto Nanocomposite, Biosensors, 2023, 13, 386, DOI:10.3390/bios13030386.
- J. Feng, Q.-Y. Huang, C. Zhang, S. Ramakrishna and Y.-B. Dong, Review of Covalent Organic Frameworks for Enzyme Immobilization: Strategies, Applications, and Prospects, Int. J. Biol. Macromol., 2023, 248, 125729, DOI:10.1016/j.ijbiomac.2023.125729.
- Z. B. Mohammadi, F. Zhang, M. S. Kharazmi and S. M. Jafari, Nano-Biocatalysts for Food Applications; Immobilized Enzymes within Different Nanostructures, Crit. Rev. Food Sci. Nutr., 2023, 63, 11351–11369, DOI:10.1080/10408398.2022.2092719.
- T. Heinks, N. Montua, M. Teune, J. Liedtke, M. Höhne, U. T. Bornscheuer and G. von Mollard, Comparison of Four Immobilization Methods for Different Transaminases, Catalysts, 2023, 13, 330, DOI:10.3390/catal13020300.
- J. Ahlawat, M. Sharma and C. S. Pundir, An Amperometric Acetylcholine Biosensor Based on Co-Immobilization of Enzyme Nanoparticles onto Nanocomposite, Biosensors, 2023, 13, 386, DOI:10.3390/bios13030386.
- A. M. Girelli and V. Chiappini, Renewable, Sustainable, and Natural Lignocellulosic Carriers for Lipase Immobilization: A Review, J. Biotechnol., 2023, 365, 29–47, DOI:10.1016/j.jbiotec.2023.02.003.
- B. H. Kim, J. Hwang and C. C. Akoh, Liquid Microbial Lipase — Recent Applications and Expanded Use through Immobilization, Curr. Opin. Food Sci., 2023, 50, 100987, DOI:10.1016/j.cofs.2023.100987.
- J. Mulinari, A. Ambrosi, Y. Feng, Z. He, X. Huang, Q. Li, M. Di Luccio, D. Hotza and J. V. Oliveira, Polydopamine-Assisted One-Step Immobilization of Lipase on α-Alumina Membrane for Fouling Control in the Treatment of Oily Wastewater, Chem. Eng. J., 2023, 459, 141516, DOI:10.1016/j.cej.2023.141516.
- F. Aghabeigi, H. Nikkhah, H. Zilouei and M. Bazarganipour, Immobilization of Lipase on the Graphene Oxides Magnetized with NiFe2O4 Nanoparticles for Biodiesel Production from Microalgae Lipids, Process Biochem., 2023, 126, 171–185, DOI:10.1016/j.procbio.2023.01.012.
- Y. Peng, M. Wang, X. Huang, F. Yang, Y. Shi, C. Liao and D. Yu, Investigation into the Magnetic Immobilization of Lipase and Its Application in the Synthesis of Structured Triacylglycerols, LWT, 2023, 174, 114466, DOI:10.1016/j.lwt.2023.114466.
- R. L. F. Melo, M. B. Sales, V. de Castro Bizerra, P. G. de Sousa Junior, A. L. G. Cavalcante, T. M. Freire, F. S. Neto, M. Bilal, T. Jesionowski and J. M. Soares, et al., Recent Applications and Future Prospects of Magnetic Biocatalysts, Int. J. Biol. Macromol., 2023, 253, 126709, DOI:10.1016/j.ijbiomac.2023.126709.
- D. Yang, X. Wang, J. Shi, X. Wang, S. Zhang, P. Han and Z. Jiang, In Situ Synthesized RGO–Fe3O4 Nanocomposites as Enzyme Immobilization Support for Achieving High Activity Recovery and Easy Recycling, Biochem. Eng. J., 2016, 105, 273–280, DOI:10.1016/j.bej.2015.10.003.
- J. Lin, Q. Wen, S. Chen, X. Le, X. Zhou and L. Huang, Synthesis of Amine-Functionalized Fe3O4@C Nanoparticles for Laccase Immobilization, Int. J. Biol. Macromol., 2017, 96, 377–383, DOI:10.1016/j.ijbiomac.2016.12.051.
- V. Mehnati-Najafabadi, A. Taheri-Kafrani and A.-K. Bordbar, Xylanase Immobilization on Modified Superparamagnetic Graphene Oxide Nanocomposite: Effect of PEGylation on Activity and Stability, Int. J. Biol. Macromol., 2018, 107, 418–425, DOI:10.1016/j.ijbiomac.2017.09.013.
- F. Xue, Q. Chen, Y. Li, E. Liu and D. Li, Immobilized Lysozyme onto 1,2,3,4-Butanetetracarboxylic (BTCA)-Modified Magnetic Cellulose Microsphere for Improving Bio-Catalytic Stability and Activities, Enzyme Microb. Technol., 2019, 131, 109425, DOI:10.1016/j.enzmictec.2019.109425.
- R. M. Bezerra, R. R. C. Monteiro, D. M. A. Neto, F. F. M. da Silva, R. C. M. de Paula, T. L. G. de Lemos, P. B. A. Fechine, M. A. Correa, F. Bohn and L. R. B. Gonçalves, et al., A New Heterofunctional Support for Enzyme Immobilization: PEI Functionalized Fe3O4 MNPs Activated with Divinyl Sulfone. Application in the Immobilization of Lipase from Thermomyces Lanuginosus, Enzyme Microb. Technol., 2020, 138, 109560, DOI:10.1016/j.enzmictec.2020.109560.
- T. C. Coutinho, J. O. D. Malafatti, E. C. Paris, P. W. Tardioli and C. S. Farinas, Hydroxyapatite-CoFe2O4 Magnetic Nanoparticle Composites for Industrial Enzyme Immobilization, Use, and Recovery, ACS Appl. Nano Mater., 2020, 3, 12334–12345, DOI:10.1021/acsanm.0c02811.
- T. Carvalho, S. da, A. Pereira, R. C. F. Bonomo, M. Franco, P. V. Finotelli and P. F. F. Amaral, Simple Physical Adsorption Technique to Immobilize Yarrowia Lipolytica Lipase Purified by Different Methods on Magnetic Nanoparticles: Adsorption Isotherms and Thermodynamic Approach, Int. J. Biol. Macromol., 2020, 160, 889–902, DOI:10.1016/j.ijbiomac.2020.05.174.
- G. Coşkun, Z. Çıplak, N. Yıldız and Ü. Mehmetoğlu, Immobilization of Candida Antarctica Lipase on Nanomaterials and Investigation of the Enzyme Activity and Enantioselectivity, Appl. Biochem. Biotechnol., 2021, 193, 430–445, DOI:10.1007/s12010-020-03443-2.
- R. Perveen, A. Nasar, Inamuddin, S. Kanchi and H. A. Kashmery, Development of a Ternerry Condunting Composite (PPy/Au/CNT@Fe3O4) Immobilized FRT/GOD Bioanode for Glucose/Oxygen Biofuel Cell Applications, Int. J. Hydrogen Energy, 2021, 46, 3259–3269, DOI:10.1016/j.ijhydene.2020.02.175.
- F. R. Paz-Cedeno, J. M. Carceller, S. Iborra, R. K. Donato, A. P. Godoy, A. Veloso de Paula, R. Monti, A. Corma and F. Masarin, Magnetic Graphene Oxide as a Platform for the Immobilization of Cellulases and Xylanases: Ultrastructural Characterization and Assessment of Lignocellulosic Biomass Hydrolysis, Renewable Energy, 2021, 164, 491–501, DOI:10.1016/j.renene.2020.09.059.
- Y. Osuna, J. Sandoval, H. Saade, R. G. López, J. L. Martinez, E. M. Colunga, G. de la Cruz, E. P. Segura, F. J. Arévalo and M. A. Zon, et al., Immobilization of Aspergillus Niger Lipase on Chitosan-Coated Magnetic Nanoparticles Using Two Covalent-Binding Methods, Bioprocess Biosyst. Eng., 2015, 38, 1437–1445, DOI:10.1007/s00449-015-1385-8.
- W. Xie and X. Zang, Immobilized Lipase on Core-Shell Structured Fe3O4-MCM-41 Nanocomposites as a Magnetically Recyclable Biocatalyst for Interesterification of Soybean Oil and Lard, Food Chem., 2016, 194, 1283–1292, DOI:10.1016/j.foodchem.2015.09.009.
- K. Atacan, B. Çakiroǧlu and M. Özacar, Improvement of the Stability and Activity of Immobilized Trypsin on Modified Fe3O4 Magnetic Nanoparticles for Hydrolysis of Bovine Serum Albumin and Its Application in the Bovine Milk, Food Chem., 2016, 212, 460–468, DOI:10.1016/j.foodchem.2016.06.011.
- C. C. S. Fortes, A. L. Daniel-da-Silva, A. M. R. B. Xavier and A. P. M. Tavares, Optimization of Enzyme Immobilization on Functionalized Magnetic Nanoparticles for Laccase Biocatalytic Reactions, Chem. Eng. Process., 2017, 117, 1–8, DOI:10.1016/j.cep.2017.03.009.
- M. Heidarizadeh, E. Doustkhah, S. Rostamnia, P. F. Rezaei, F. D. Harzevili and B. Zeynizadeh, Dithiocarbamate to Modify Magnetic Graphene Oxide Nanocomposite (Fe3O4-GO): A New Strategy for Covalent Enzyme (Lipase) Immobilization to Fabrication a New Nanobiocatalyst for Enzymatic Hydrolysis of PNPD, Int. J. Biol. Macromol., 2017, 101, 696–702, DOI:10.1016/j.ijbiomac.2017.03.152.
- W. S. Galvão, B. B. Pinheiro, L. R. B. Golçalves, M. C. de Mattos, T. S. Fonseca, T. Regis, D. Zampieri, J. C. S. dos Santos, L. S. Costa and M. A. Correa, et al., Novel Nanohybrid Biocatalyst: Application in the Kinetic Resolution of Secondary Alcohols, J. Mater. Sci., 2018, 53, 14121–14137, DOI:10.1007/s10853-018-2641-5.
- W. Xie and M. Huang, Immobilization of Candida Rugosa Lipase onto Graphene Oxide Fe3O4 Nanocomposite: Characterization and Application for Biodiesel Production, Energy Convers. Manage., 2018, 159, 42–53, DOI:10.1016/j.enconman.2018.01.021.
- F. Xue, Q. Chen, Y. Li, E. Liu and D. Li, Immobilized Lysozyme onto 1,2,3,4-Butanetetracarboxylic (BTCA)-Modified Magnetic Cellulose Microsphere for Improving Bio-Catalytic Stability and Activities, Enzyme Microb. Technol., 2019, 131, 109425, DOI:10.1016/j.enzmictec.2019.109425.
- R. M. Bezerra, R. R. C. Monteiro, D. M. A. Neto, F. F. M. da Silva, R. C. M. de Paula, T. L. G. de Lemos, P. B. A. Fechine, M. A. Correa, F. Bohn and L. R. B. Gonçalves, et al., A New Heterofunctional Support for Enzyme Immobilization: PEI Functionalized Fe3O4 MNPs Activated with Divinyl Sulfone. Application in the Immobilization of Lipase from Thermomyces Lanuginosus, Enzyme Microb. Technol., 2020, 138, 109560, DOI:10.1016/j.enzmictec.2020.109560.
- T. C. Coutinho, J. O. D. Malafatti, E. C. Paris, P. W. Tardioli and C. S. Farinas, Hydroxyapatite-CoFe2O4Magnetic Nanoparticle Composites for Industrial Enzyme Immobilization, Use, and Recovery, ACS Appl. Nano Mater., 2020, 3, 12334–12345, DOI:10.1021/acsanm.0c02811.
- S. Shanmugam, S. Krishnaswamy, R. Chandrababu, U. Veerabagu, A. Pugazhendhi and T. Mathimani, Optimal Immobilization of Trichoderma Asperellum Laccase on Polymer Coated Fe3O4@SiO2 Nanoparticles for Enhanced Biohydrogen Production from Delignified Lignocellulosic Biomass, Fuel, 2020, 273, 117777, DOI:10.1016/j.fuel.2020.117777.
- G. Coşkun, Z. Çıplak, N. Yıldız and Ü. Mehmetoğlu, Immobilization of Candida Antarctica Lipase on Nanomaterials and Investigation of the Enzyme Activity and Enantioselectivity, Appl. Biochem. Biotechnol., 2021, 193, 430–445, DOI:10.1007/s12010-020-03443-2.
- U. Kilimci, S. Evli, B. Öndeş, M. Uygun and D. A. Uygun, Inulinase Immobilized Lectin Affinity Magnetic Nanoparticles for Inulin Hydrolysis, Appl. Biochem. Biotechnol., 2021, 1415–1426, DOI:10.1007/s12010-020-03476-7.
- F. R. Paz-Cedeno, J. M. Carceller, S. Iborra, R. K. Donato, A. P. Godoy, A. Veloso de Paula, R. Monti, A. Corma and F. Masarin, Magnetic Graphene Oxide
as a Platform for the Immobilization of Cellulases and Xylanases: Ultrastructural Characterization and Assessment of Lignocellulosic Biomass Hydrolysis, Renewable Energy, 2021, 164, 491–501, DOI:10.1016/j.renene.2020.09.059.
- J. Ayub, M. U. Saeed, N. Hussain, I. Zulfiqar, T. Mehmood, H. M. N. Iqbal and M. Bilal, Designing Robust Nano-Biocatalysts Using Nanomaterials as Multifunctional Carriers - Expanding the Application Scope of Bio-Enzymes, Top. Catal., 2023, 66, 625–648, DOI:10.1007/s11244-022-01657-8.
- H. Yamaguchi and M. Miyazaki, Enzyme-Immobilized Microfluidic Devices for Biomolecule Detection, TrAC, Trends Anal. Chem., 2023, 159, 116908, DOI:10.1016/j.trac.2022.116908.
- Y. R. Maghraby, R. M. El-Shabasy, A. H. Ibrahim and H. M. E. S. Azzazy, Enzyme Immobilization Technologies and Industrial Applications, ACS Omega, 2023, 8, 5184–5196, DOI:10.1021/acsomega.2c07560.
- E. S. Ribeiro, B. S. de Farias, T. R. Sant'Anna Cadaval Junior, L. A. de Almeida Pinto and P. S. Diaz, Chitosan–Based Nanofibers for Enzyme Immobilization, Int. J. Biol. Macromol., 2021, 183, 1959–1970, DOI:10.1016/j.ijbiomac.2021.05.214.
- M. Salehipour, S. Rezaei, M. Yazdani and M. Mogharabi-Manzari, Recent advances in preparation of polymer hydrogel composites and their applications in enzyme immobilization, Polym. Bull., 2023, 80 DOI:10.1007/s00289-022-04370-4.
- Y. Bai, Z. Jing, R. Ma, X. Wan, J. Liu and W. Huang, A Critical Review of Enzymes Immobilized on Chitosan Composites: Characterization and Applications, Bioprocess Biosyst. Eng., 2023, 46, 1539–1567, DOI:10.1007/s00449-023-02914-0.
- H. T. Imam, P. C. Marr and A. C. Marr, Enzyme Entrapment, Biocatalyst Immobilization without Covalent Attachment, Green Chem., 2021, 23, 4980–5005, 10.1039/d1gc01852c.
- J. R. Fernandez Caresani, A. Dallegrave and J. H. Z. dos Santos, Amylases Immobilization by Sol–Gel Entrapment: Application for Starch Hydrolysis, J. Sol-Gel Sci. Technol., 2020, 94, 229–240, DOI:10.1007/s10971-019-05136-7.
- S. Datta, L. R. Christena and Y. R. S. Rajaram, Enzyme Immobilization: An Overview on Techniques and Support Materials, 3 Biotech, 2013, 3, 1–9, DOI:10.1007/s13205-012-0071-7.
- J. Shen, S. Zhang, X. Fang and S. Salmon, Advances in 3D Gel Printing for Enzyme Immobilization, Gels, 2022, 8, 460, DOI:10.3390/gels8080460.
- Y. V. Samoylova, K. N. Sorokina, A. V. Piligaev and V. N. Parmon, Preparation of Stable Cross-Linked Enzyme Aggregates (CLEAs) of a Ureibacillus Thermosphaericus Esterase for Application in Malathion Removal from Wastewater, Catalysts, 2018, 8, 154, DOI:10.3390/catal8040154.
- S. S. Nadar, A. B. Muley, M. R. Ladole and P. U. Joshi, Macromolecular Cross-Linked Enzyme Aggregates (M-CLEAs) of α-Amylase, Int. J. Biol. Macromol., 2016, 84, 69–78, DOI:10.1016/j.ijbiomac.2015.11.082.
- H. H. Nguyen and M. Kim, An Overview of Techniques in Enzyme Immobilization, Appl. Sci. Converg. Technol., 2017, 26, 157–163, DOI:10.5757/asct.2017.26.6.157.
- L. Dal Magro, P. F. Hertz, R. Fernandez-Lafuente, M. P. Klein and R. C. Rodrigues, Preparation and Characterization of a Combi-CLEAs from Pectinases and Cellulases: A Potential Biocatalyst for Grape Juice Clarification, RSC Adv., 2016, 6, 27242–27251, 10.1039/c6ra03940e.
- A. Badoei-dalfard, S. Malekabadi, Z. Karami and G. Sargazi, Magnetic Cross-Linked Enzyme Aggregates of Km12 Lipase: A Stable Nanobiocatalyst for Biodiesel Synthesis from Waste Cooking Oil, Renewable Energy, 2019, 141, 874–882, DOI:10.1016/j.renene.2019.04.061.
- R. Verma, A. Kumar and S. Kumar, Synthesis and Characterization of Cross-Linked Enzyme Aggregates (CLEAs) of Thermostable Xylanase from Geobacillus Thermodenitrificans X1, Process Biochem., 2019, 80, 72–79, DOI:10.1016/j.procbio.2019.01.019.
- M. J. Rojas, M. Amaral-Fonseca, G. M. Zanin, R. Fernandez-Lafuente, R. de L. C. Giordano and P. W. Tardioli, Preparation of Crosslinked Enzyme Aggregates of a Thermostable Cyclodextrin Glucosyltransferase from Thermoanaerobacter Sp. Critical Effect of the Crosslinking Agent, Catalysts, 2019, 9, 120, DOI:10.3390/catal9020120.
- H. H. Nguyen and M. Kim, An Overview of Techniques in Enzyme Immobilization, Appl. Sci. Converg. Technol., 2017, 26, 157–163, DOI:10.5757/asct.2017.26.6.157.
- L. Zhou, H. Mou, J. Gao, L. Ma, Y. He and Y. Jiang, Preparation of Cross-Linked Enzyme Aggregates of Nitrile Hydratase ES-NHT-118 from E. Coli by Macromolecular Cross-Linking Agent, Chin. J. Chem. Eng., 2017, 25, 487–492, DOI:10.1016/j.cjche.2016.08.027.
- B. S. Chang and R. R. Mahoney, Enzyme Thermostabilization by Bovine Serum Albumin and Other Proteins: Evidence for Hydrophobic Interactions, Biotechnol. Appl. Biochem., 1995, 203–214, DOI:10.1111/j.1470-8744.1995.tb00346.x.
- C. Wong, T. J. Lee, T. Y. Lee, T. H. Lu and C. S. Hung, Intermolecular Cross-Linking of a Protein Crystal - Acid Protease from Endothia Parasitica - in 2.7 M Ammonium Sulfate Solution, Biochem. Biophys. Res. Commun., 1978, 80, 886–890, DOI:10.1016/0006-291X(78)91327-X.
- G. M. Alter, D. L. Leussing, H. Neurath and B. L. Vallee, Kinetic Properties of Carboxypeptidase B in Solutions and Crystals, Biochemistry, 1977, 16, 3663–3668, DOI:10.1021/bi00635a024.
- T. Akkas, A. Zakharyuta, A. Taralp and C. W. Ow-Yang, Cross-Linked Enzyme Lyophilisates (CLELs) of Urease: A New Method to Immobilize Ureases, Enzyme Microb. Technol., 2020, 132, 109390, DOI:10.1016/j.enzmictec.2019.109390.
- S. Velasco-Lozano, F. López-Gallego, J. C. Mateos-Díaz and E. Favela-Torres, Cross-Linked Enzyme Aggregates (CLEA) in Enzyme Improvement – a Review, Biocatalysis, 2016, 1(47), 166–177, DOI:10.1515/boca-2015-0012.
- G. Alarcon-Angeles, G. A. Álvarez-Romero and A. Merkoçi, Electrochemical Biosensors: Enzyme Kinetics and Role of Nanomaterials, Encycl. Interfacial Chem., 2018, 140–155, DOI:10.1016/B978-0-12-409547-2.13477-8.
- C. X. You, P. H. Huang and S. C. Lin, Concomitant Selective Adsorption and Covalent Immobilization of a His-Tagged Protein Switch with Silica-Based Metal Chelate-Epoxy Bifunctional Adsorbents, Process Biochem., 2020, 99, 179–186, DOI:10.1016/j.procbio.2020.09.005.
- S. Z. Zhang, Department of Enzyme-Polymer-Materials and Enzyme Immobilization, Fraunhofer Institute for Applied Polymer Research (IAP), Geiselbergstraße 69, 14476 Potsdam, G.; Chair of Polymer Materials and Polymer Technologies, University of Potsdam, Institute of Chemistry, Karl-Liebknecht-Straße 24-25, 14476 Potsdam, G.; Zhang, M. by S.; , Julia Bramski, Murat Tutus, Jörg Pietruszka, Alexander Böker, and S.R. A Biocatalytically Active Membrane Obtained from Immobilization of 2-Deoxy-d-Ribose-5-Phosphate Aldolase on a Porous Support, ACS Appl. Mater. Interfaces, 2019, 11, 34441–34453 CrossRef CAS PubMed.
- X. Qiu, Y. Wang, Y. Xue, W. Li and Y. Hu, Laccase Immobilized on Magnetic Nanoparticles Modified by Amino-Functionalized Ionic Liquid via Dialdehyde Starch for Phenolic Compounds Biodegradation, Chem. Eng. J., 2020, 391 DOI:10.1016/j.cej.2019.123564.
- J. H. Yang, H. R. Kim, J. H. Lee, J. H. Jin, H. U. Lee and S. W. Kim, Electrochemical Properties of Enzyme Electrode Covalently Immobilized on a Graphite Oxide/Cobalt Hydroxide/Chitosan Composite Mediator for Biofuel Cells, Int. J. Hydrogen Energy, 2020, 4(46) DOI:10.1016/j.ijhydene.2020.03.084.
- M. Li, F. Cheng, H. Li, W. Jin, C. Chen, W. He, G. Cheng and Q. Wang, Site-Specific and Covalent Immobilization of His-Tagged Proteins via Surface Vinyl Sulfone-Imidazole Coupling, Langmuir, 2019, 35, 16466–16475, DOI:10.1021/acs.langmuir.9b02933.
- C. Ding, J. Liang, Z. Zhou, Y. Li, W. Peng, G. Zhang, F. Zhang and X. Fan, Photothermal Enhanced Enzymatic Activity of Lipase Covalently Immobilized on Functionalized Ti3C2TX Nanosheets, Chem. Eng. J., 2019, 378, 122205, DOI:10.1016/j.cej.2019.122205.
- J. Zdarta, A. S. Meyer, T. Jesionowski and M. Pinelo, A General Overview of Support Materials for Enzyme
Immobilization: Characteristics, Properties, Practical Utility, Catalysts, 2018, 8, 92, DOI:10.3390/catal8020092.
- M. Bilal and H. M. N. Iqbal, Naturally-Derived Biopolymers: Potential Platforms for Enzyme Immobilization, Int. J. Biol. Macromol., 2019, 130, 462–482, DOI:10.1016/j.ijbiomac.2019.02.152.
- T. Nematian, Z. Salehi and A. Shakeri, Conversion of Bio-Oil Extracted from Chlorella Vulgaris Micro Algae to Biodiesel via Modified Superparamagnetic Nano-Biocatalyst, Renewable Energy, 2020, 146, 1796–1804, DOI:10.1016/j.renene.2019.08.048.
- H. Suo, L. Xu, C. Xu, X. Qiu, H. Huang and Y. Hu, Enhanced Catalytic Performance of Lipase Covalently Bonded on Ionic Liquids Modified Magnetic Alginate Composites, J. Colloid Interface Sci., 2019, 553, 494–502, DOI:10.1016/j.jcis.2019.06.049.
- A. M. Girelli, M. L. Astolfi and F. R. Scuto, Agro-Industrial Wastes as Potential Carriers for Enzyme Immobilization: A Review, Chemosphere, 2020, 244, 125368, DOI:10.1016/j.chemosphere.2019.125368.
- C. J. Ugwuodo and T. N. Nwagu, Stabilizing Enzymes by Immobilization on Bacterial Spores: A Review of Literature, Int. J. Biol. Macromol., 2020 DOI:10.1016/j.ijbiomac.2020.10.171.
- S. Liang, X. L. Wu, J. Xiong, M. H. Zong and W. Y. Lou, Metal-Organic Frameworks as Novel Matrices for Efficient Enzyme Immobilization: An Update Review, Coord. Chem. Rev., 2020, 406, 213149, DOI:10.1016/j.ccr.2019.213149.
- S. Aggarwal, A. Chakravarty and S. Ikram, International Journal of Biological Macromolecules A Comprehensive Review on Incredible Renewable Carriers as Promising Platforms for Enzyme Immobilization & Thereof Strategies, Int. J. Biol. Macromol., 2020, 167, 962–986, DOI:10.1016/j.ijbiomac.2020.11.052.
- Y. Liu and J. Y. Chen, Enzyme Immobilization on Cellulose Matrixes, J. Bioact. Compat. Polym., 2016, 31, 553–567, DOI:10.1177/0883911516637377.
- J. M. Bolivar and B. Nidetzky, The Microenvironment in Immobilized Enzymes: Methods of Characterization and Its Role in Determining Enzyme Performance, Molecules, 2019, 24, 8–10, DOI:10.3390/molecules24193460.
- D. M. Liu, J. Chen and Y. P. Shi, Advances on Methods and Easy Separated Support Materials for Enzymes Immobilization, TrAC, Trends Anal. Chem., 2018, 102, 332–342, DOI:10.1016/j.trac.2018.03.011.
- M. Bilal, Y. Zhao, T. Rasheed and H. M. N. Iqbal, Magnetic Nanoparticles as Versatile Carriers for Enzymes Immobilization: A Review, Int. J. Biol. Macromol., 2018, 120, 2530–2544, DOI:10.1016/j.ijbiomac.2018.09.025.
- M. P. Van Der Helm, P. Bracco, H. Busch, K. Szymańska, A. B. Jarzȩbski and U. Hanefeld, Hydroxynitrile Lyases Covalently Immobilized in Continuous Flow Microreactors, Catal. Sci. Technol., 2019, 9, 1189–1200, 10.1039/c8cy02192a.
- Y. Cárdenas‐Moreno, J. González‐Bacerio, H. García Arellano and A. del Monte‐Martínez, Oxidoreductase Enzymes: Characteristics, Applications, and Challenges as a Biocatalyst, Biotechnol. Appl. Biochem., 2023, 70, 2108–2135, DOI:10.1002/bab.2513.
- L. Sellés Vidal, C. L. Kelly, P. M. Mordaka and J. T. Heap, Review of NAD(P)H-Dependent Oxidoreductases: Properties, Engineering and Application, Biochim. Biophys. Acta, Proteins Proteomics, 2018, 1866, 327–347, DOI:10.1016/j.bbapap.2017.11.005.
- M. Marcia, J. D. Langer, D. Parcej, V. Vogel, G. Peng and H. Michel, Characterizing a Monotopic Membrane Enzyme. Biochemical, Enzymatic and Crystallization Studies on Aquifex Aeolicus Sulfide:Quinone Oxidoreductase, Biochim. Biophys. Acta, Biomembr., 2010, 1798, 2114–2123, DOI:10.1016/j.bbamem.2010.07.033.
- S. W. May, Applications of Oxidoreductases, Curr. Opin. Biotechnol., 1999, 10, 370–375, DOI:10.1016/S0958-1669(99)80067-6.
- S. Datta, R. Veena, M. S. Samuel and E. Selvarajan, Immobilization of Laccases and Applications for the Detection and Remediation of Pollutants: A Review, Environ. Chem. Lett., 2021, 19, 521–538, DOI:10.1007/s10311-020-01081-y.
- P. Upadhyay, R. Shrivastava and P. K. Agrawal, Bioprospecting and Biotechnological Applications of Fungal Laccase, 3 Biotech, 2016, 6, 15, DOI:10.1007/s13205-015-0316-3.
- Biocatalysis Based on Heme Peroxidases, ed. E. Torres and M. Ayala, Springer Berlin Heidelberg, Berlin, Heidelberg, 2010 Search PubMed.
- L. Sellés Vidal, C. L. Kelly, P. M. Mordaka and J. T. Heap, Review of NAD(P)H-Dependent Oxidoreductases: Properties, Engineering and Application, Biochim. Biophys. Acta, Proteins Proteomics, 2018, 1866, 327–347, DOI:10.1016/j.bbapap.2017.11.005.
- O. Hayaishi, Oxygenases, in Encyclopedia of Biological Chemistry, Elsevier, 2004, pp. 178–182 Search PubMed.
- G. K. Kouassi, J. Irudayaraj and G. McCarty, Examination of Cholesterol Oxidase Attachment to Magnetic Nanoparticles, J. Nanobiotechnol., 2005, 3, 1, DOI:10.1186/1477-3155-3-1.
- G. K. Kouassi, J. Irudayaraj and G. McCarty, Examination of Cholesterol Oxidase Attachment to Magnetic Nanoparticles, J. Nanobiotechnol., 2005, 3, 1, DOI:10.1186/1477-3155-3-1.
- L. Pollegioni, G. Wels, M. S. Pilone and S. Ghisla, Kinetic Mechanisms of Cholesterol Oxidase from Streptomyces Hygroscopicus and Brevibacterium Sterolicum, Eur. J. Biochem., 1999, 264, 140–151, DOI:10.1046/j.1432-1327.1999.00586.x.
- S.-H. Huang, M.-H. Liao and D.-H. Chen, Direct Binding and Characterization of Lipase onto Magnetic Nanoparticles, Biotechnol. Prog., 2003, 19, 1095–1100, DOI:10.1021/bp025587v.
- J. Liu, W. Kong, J. Bai, Y. Li, L. Dong, L. Zhou, Y. Liu, J. Gao, R. T. Bradshaw Allen and N. J. Turner, et al., Amine Dehydrogenases: Current Status and Potential Value for Chiral Amine Synthesis, Chem Catal., 2022, 2, 1288–1314, DOI:10.1016/j.checat.2022.03.018.
- H. Ren, Y. Zhang, J. Su, P. Lin, B. Wang, B. Fang and S. Wang, Encapsulation of Amine Dehydrogenase in Hybrid Titania Nanoparticles by Polyethylenimine Coating and Templated Biomineralization, J. Biotechnol., 2017, 241, 33–41, DOI:10.1016/j.jbiotec.2016.11.006.
- J. Zdarta, A. S. Meyer, T. Jesionowski and M. Pinelo, Developments in Support Materials for Immobilization of Oxidoreductases: A Comprehensive Review, Adv. Colloid Interface Sci., 2018, 258, 1–20, DOI:10.1016/j.cis.2018.07.004.
- A. T. Martínez, F. J. Ruiz-Dueñas, S. Camarero, A. Serrano, D. Linde, H. Lund, J. Vind, M. Tovborg, O. M. Herold-Majumdar and M. Hofrichter, et al., Oxidoreductases on Their Way to Industrial Biotransformations, Biotechnol. Adv., 2017, 35, 815–831, DOI:10.1016/j.biotechadv.2017.06.003.
- C. Y. Zamora, N. S. Schocker, M. M. Chang and B. Imperiali, Chemoenzymatic synthesis and applications of prokaryote-specific UDP-sugars, Methods Enzymol., 2017, 145–186, DOI:10.1016/bs.mie.2017.06.003.
- M. Zhang, C. An, Y. Gao, R. K. Leak, J. Chen and F. Zhang, Emerging Roles of Nrf2 and Phase II Antioxidant Enzymes in Neuroprotection, Prog. Neurobiol., 2013, 100, 30–47, DOI:10.1016/j.pneurobio.2012.09.003.
- K. Nakata, Y. Tanaka, T. Nakano, T. Adachi, H. Tanaka, T. Kaminuma and T. Ishikawa, Nuclear Receptor-Mediated Transcriptional Regulation in Phase I, II, and III Xenobiotic Metabolizing Systems, Drug Metab. Pharmacokinet., 2006, 21, 437–457, DOI:10.2133/dmpk.21.437.
- D. Sheehan, G. Meade, V. M. Foley and C. A. Dowd, Structure, Function and Evolution of Glutathione Transferases: Implications for Classification of Non-Mammalian Members of an Ancient Enzyme Superfamily, Biochem. J., 2001, 360, 1, DOI:10.1042/0264-6021:3600001.
- C. Xu, C. Y.-T. Li and A.-N. T. Kong, Induction of Phase I, II and III Drug Metabolism/Transport by Xenobiotics, Arch. Pharmacal Res., 2005, 28, 249–268, DOI:10.1007/BF02977789.
- R. S. Obach and N. Isoherranen, Pathways of drug metabolism, in Atkinson's Principles of Clinical Pharmacology, Elsevier, 2022, pp. 151–168 Search PubMed.
- A. Shabbir, K. Haider, K. Rehman, M. S. H. Akash and S. Chen, Biochemical activation and functions of drug-metabolizing enzymes, in Biochemistry of Drug Metabolizing Enzymes, Elsevier, 2022, pp. 1–27 Search PubMed.
- E. Romeih and G. Walker, Recent Advances on Microbial Transglutaminase and Dairy Application, Trends Food Sci. Technol., 2017, 62, 133–140, DOI:10.1016/j.tifs.2017.02.015.
- M. Griffin, R. Casadio and C. M. Bergamini, Transglutaminases: Nature's Biological Glues, Biochem. J., 2002, 368, 377–396, DOI:10.1042/bj20021234.
- S. Taguchi, K. Arakawa, K. Yokoyama, S. Takehana, H. Takagi and H. Momose, Overexpression and Purification of Microbial Pro-Transglutaminase from Streptomyces Cinnamoneum and in Vitro Processing by Streptomyces Albogriseolus Proteases, J. Biosci. Bioeng., 2002, 94, 478–481, DOI:10.1016/S1389-1723(02)80228-6.
- M. Date, K. Yokoyama, Y. Umezawa, H. Matsui and Y. Kikuchi, Production of Native-Type Streptoverticillium Mobaraense Transglutaminase in Corynebacterium Glutamicum, Appl. Environ. Microbiol., 2003, 69, 3011–3014, DOI:10.1128/AEM.69.5.3011-3014.2003.
- M.-T. Yang, C.-H. Chang, J. M. Wang, T. K. Wu, Y.-K. Wang, C.-Y. Chang and T. T. Li, Crystal Structure and Inhibition Studies of Transglutaminase from Streptomyces Mobaraense, J. Biol. Chem., 2011, 286, 7301–7307, DOI:10.1074/jbc.M110.203315.
- M. Gajšek, U. Jančič, K. Vasić, Ž. Knez and M. Leitgeb, Enhanced Activity of Immobilized Transglutaminase for Cleaner Production Technologies, J. Cleaner Prod., 2019, 240, 118218, DOI:10.1016/j.jclepro.2019.118218.
- A. L. C. Gaspar and S. P. de Góes-Favoni, Action of Microbial Transglutaminase (MTGase) in the Modification of Food Proteins: A Review, Food Chem., 2015, 171, 315–322, DOI:10.1016/j.foodchem.2014.09.019.
- C.-M. Yu, H. Zhou, W.-F. Zhang, H.-M. Yang and J.-B. Tang, Site-Specific, Covalent Immobilization of BirA by Microbial Transglutaminase: A Reusable Biocatalyst for in Vitro Biotinylation, Anal. Biochem., 2016, 511, 10–12, DOI:10.1016/j.ab.2016.07.026.
- J. Cortez, P. L. R. Bonner and M. Griffin, Transglutaminase Treatment of Wool Fabrics Leads to Resistance to Detergent Damage, J. Biotechnol., 2005, 116, 379–386, DOI:10.1016/j.jbiotec.2004.12.007.
- C. E. Brubaker and P. B. Messersmith, Biological adhesion, in Polymer Science: A Comprehensive Reference, Elsevier, 2012, pp. 211–229 Search PubMed.
- G. Hojnik Podrepšek, Ž. Knez and M. Leitgeb, The Synthesis of (Magnetic) Crosslinked Enzyme Aggregates With Laccase, Cellulase, β-Galactosidase and Transglutaminase, Front. Bioeng. Biotechnol., 2022, 10(57), 3–10, DOI:10.3389/fbioe.2022.813919.
- M. Kieliszek and A. Misiewicz, Microbial Transglutaminase and Its Application in the Food Industry. A Review, Folia Microbiol., 2014, 59, 241–250, DOI:10.1007/s12223-013-0287-x.
- E. Romeih and G. Walker, Recent Advances on Microbial Transglutaminase and Dairy Application, Trends Food Sci. Technol., 2017, 62, 133–140, DOI:10.1016/j.tifs.2017.02.015.
- G. Bardini, F. Boukid, E. Carini, E. Curti, E. Pizzigalli and E. Vittadini, Enhancing Dough-Making Rheological Performance of Wheat Flour by Transglutaminase and Vital Gluten Supplementation, LWT, 2018, 91, 467–476, DOI:10.1016/j.lwt.2018.01.077.
- W. Wang, X. Han, H. Yi and L. Zhang, The Ultrafiltration Efficiency and Mechanism of Transglutaminase Enzymatic Membrane Reactor (EMR) for Protein Recovery from Cheese Whey, Int. Dairy J., 2018, 80, 52–61, DOI:10.1016/j.idairyj.2017.12.012.
- J. Q. Zhou, T. He and J. W. Wang, The Microbial Transglutaminase Immobilization on Carboxylated Poly(N-Isopropylacrylamide) for Thermo-Responsivity, Enzyme Microb. Technol., 2016, 87–88, 44–51, DOI:10.1016/j.enzmictec.2016.02.012.
- L. Stanca, O. I. Geicu, A. I. Serban and A. Dinischiotu, Interplay of Oxidative Stress, Inflammation, and Autophagy in RAW 264.7 Murine Macrophage Cell Line Challenged with Si/SiO2 Quantum Dots, Materials, 2023, 16, 5083, DOI:10.3390/ma16145083.
- M. Gao, Q. Liu, Y. Xue, B. Li, X. Liu, Z. Shi, N. Liu and X. Zou, Facile Synthesis of Peanut-like Sn-Doped Silica Nano-Adsorbent for Affinity Separation of Proteins, RSC Adv., 2022, 12, 4697–4702, 10.1039/D1RA08362G.
- O. Sacher, M. Reitz and J. Gasteiger, Classification of Enzyme-Catalyzed Reactions Based on Physicochemical Descriptors : Investigations on Hydrolases, Mol. Biol., 2008, 1–42 Search PubMed.
- S. F. Sousa, M. J. Ramos, C. Lim and P. A. Fernandes, Relationship between Enzyme/Substrate Properties and Enzyme Efficiency in Hydrolases, ACS Catal., 2015, 5, 5877–5887, DOI:10.1021/acscatal.5b00923.
- R. Wohlgemuth, J. Littlechild, D. Monti, K. Schnorr, T. van Rossum, B. Siebers, P. Menzel, I. V. Kublanov, A. G. Rike and G. Skretas, et al., Discovering Novel Hydrolases from Hot Environments, Biotechnol. Adv., 2018, 36, 2077–2100, DOI:10.1016/j.biotechadv.2018.09.004.
- R. Greiner, U. Konietzny, D. M. Blackburn and M. A. Jorquera, Production of Partially Phosphorylated Myo-Inositol Phosphates Using Phytases Immobilised on Magnetic Nanoparticles, Bioresour. Technol., 2013, 142, 375–383, DOI:10.1016/j.biortech.2013.05.056.
- A. Sahu, P. S. Badhe, R. Adivarekar, M. R. Ladole and A. B. Pandit, Synthesis of Glycinamides Using Protease Immobilized Magnetic Nanoparticles, Biotechnol. Rep., 2016, 12, 13–25, DOI:10.1016/j.btre.2016.07.002.
- R. Li, G. Fu, C. Liu, D. J. McClements, Y. Wan, S. Wang and T. Liu, Tannase Immobilisation by Amino-Functionalised Magnetic Fe3O4-Chitosan Nanoparticles and Its Application in Tea Infusion, Int. J. Biol. Macromol., 2018, 114, 1134–1143, DOI:10.1016/j.ijbiomac.2018.03.077.
- M. Defaei, A. Taheri-Kafrani, M. Miroliaei and P. Yaghmaei, Improvement of Stability and Reusability of α-Amylase Immobilized on Naringin Functionalized Magnetic Nanoparticles: A Robust Nanobiocatalyst, Int. J. Biol. Macromol., 2018, 113, 354–360, DOI:10.1016/j.ijbiomac.2018.02.147.
- L. Križnik, K. Vasić, Ž. Knez and M. Leitgeb, Hyper-Activation of ß-Galactosidase from Aspergillus Oryzae via Immobilization onto Amino-Silane and Chitosan Magnetic Maghemite Nanoparticles, J. Cleaner Prod., 2018, 179, 225–234, DOI:10.1016/j.jclepro.2018.01.117.
- X. Cao, H. Xu, F. Li, Y. Zou, Y. Ran, X. Ma, Y. Cao, Q. Xu, D. Qiao and Y. Cao, One-Step Direct Transesterification of Wet Yeast for Biodiesel Production Catalyzed by Magnetic Nanoparticle-Immobilized Lipase, Renewable Energy, 2021, 171, 11–21, DOI:10.1016/j.renene.2021.02.065.
- V. D. Nguyen, G. Styevkó, E. Madaras, G. Haktanirlar, A. T. M. Tran, E. Bujna, M. S. Dam and Q. D. Nguyen, Immobilization of β-Galactosidase on Chitosan-Coated Magnetic Nanoparticles and Its Application for Synthesis of Lactulose-Based Galactooligosaccharides, Process Biochem., 2019, 84, 30–38, DOI:10.1016/j.procbio.2019.05.021.
- M. Ashjari, M. Garmroodi, F. Amiri Asl, M. Emampour, M. Yousefi, M. Pourmohammadi Lish, Z. Habibi and M. Mohammadi, Application of Multi-Component Reaction for Covalent Immobilization of Two Lipases on Aldehyde-Functionalized
Magnetic Nanoparticles; Production of Biodiesel from Waste Cooking Oil, Process Biochem., 2020, 90, 156–167, DOI:10.1016/j.procbio.2019.11.002.
- M. F. Khan, D. Kundu, C. Hazra and S. Patra, A Strategic Approach of Enzyme Engineering by Attribute Ranking and Enzyme Immobilization on Zinc Oxide Nanoparticles to Attain Thermostability in Mesophilic Bacillus Subtilis Lipase for Detergent Formulation, Int. J. Biol. Macromol., 2019, 136, 66–82, DOI:10.1016/j.ijbiomac.2019.06.042.
- D. A. Mota, D. Rajan, G. C. Heinzl, N. M. Osório, J. Gominho, L. C. Krause, C. M. F. Soares, K. M. Nampoothiri, R. K. Sukumaran and S. Ferreira-Dias, Production of Low-Calorie Structured Lipids from Spent Coffee Grounds or Olive Pomace Crude Oils Catalyzed by Immobilized Lipase in Magnetic Nanoparticles, Bioresour. Technol., 2020, 307, 123223, DOI:10.1016/j.biortech.2020.123223.
- J. Wang, K. Li, Y. He, Y. Wang, X. Han and Y. Yan, Enhanced Performance of Lipase Immobilized onto Co2+-Chelated Magnetic Nanoparticles and Its Application in Biodiesel Production, Fuel, 2019, 255, 115794, DOI:10.1016/j.fuel.2019.115794.
- M. Khoobi, S. F. Motevalizadeh, Z. Asadgol, H. Forootanfar, A. Shafiee and M. A. Faramarzi, Polyethyleneimine-Modified Superparamagnetic Fe3O4 Nanoparticles for Lipase Immobilization: Characterization and Application, Mater. Chem. Phys., 2015, 149, 77–86, DOI:10.1016/j.matchemphys.2014.09.039.
- D. Alagöz, A. Toprak, D. Yildirim, S. S. Tükel and R. Fernandez-Lafuente, Modified Silicates and Carbon Nanotubes for Immobilization of Lipase from Rhizomucor Miehei: Effect of Support and Immobilization Technique on the Catalytic Performance of the Immobilized Biocatalysts, Enzyme Microb. Technol., 2021, 144, 271, DOI:10.1016/j.enzmictec.2020.109739.
- A. Ameri, M. Shakibaie, M. Khoobi, M. A. Faramarzi, E. Gholibegloo, A. Ameri and H. Forootanfar, Optimization of Immobilization Conditions of Bacillus Atrophaeus FSHM2 Lipase on Maleic Copolymer Coated Amine-Modified Graphene Oxide Nanosheets and Its Application for Valeric Acid Esterification, Int. J. Biol. Macromol., 2020, 162, 1790–1806, DOI:10.1016/j.ijbiomac.2020.08.101.
- N. A. Yazid, R. Barrena and A. Sánchez, The Immobilisation of Proteases Produced by SSF onto Functionalized Magnetic Nanoparticles: Application in the Hydrolysis of Different Protein Sources, J. Mol. Catal. B: Enzym., 2016, 133, S230–S242, DOI:10.1016/j.molcatb.2017.01.009.
- S. Ashkevarian, J. Badraghi, F. Mamashli, B. Delavari and A. Akbar Saboury, Covalent Immobilization and Characterization of Rhizopus Oryzae Lipase on Core-Shell Cobalt Ferrite Nanoparticles for Biodiesel Production, Chin. J. Chem. Eng., 2020, 37 DOI:10.1016/j.cjche.2020.11.003.
- S. Asmat, A. H. Anwer and Q. Husain, Immobilization of Lipase onto Novel Constructed Polydopamine Grafted Multiwalled Carbon Nanotube Impregnated with Magnetic Cobalt and Its Application in Synthesis of Fruit Flavours, Int. J. Biol. Macromol., 2019, 140, 484–495, DOI:10.1016/j.ijbiomac.2019.08.086.
- J. S. de Lima, M. P. Cabrera, C. M. de Souza Motta, A. Converti and L. B. Carvalho, Hydrolysis of Tannins by Tannase Immobilized onto Magnetic Diatomaceous Earth Nanoparticles Coated with Polyaniline, Food Res. Int., 2018, 107, 470–476, DOI:10.1016/j.foodres.2018.02.066.
- J. Lyu, Z. Li, J. Men, R. Jiang, G. Tang, Y. Zhou and R. Gao, Covalent Immobilization of Bacillus Subtilis Lipase A on Fe3O4 Nanoparticles by Aldehyde Tag: An Ideal Immobilization with Minimal Chemical Modification, Process Biochem., 2019, 81, 63–69, DOI:10.1016/j.procbio.2019.03.017.
- S. M. Hosseini, S. M. Kim, M. Sayed, H. Younesi, N. Bahramifar, J. H. Park and S. H. Pyo, Lipase-Immobilized Chitosan-Crosslinked Magnetic Nanoparticle as a Biocatalyst for Ring Opening Esterification of Itaconic Anhydride, Biochem. Eng. J., 2019, 143, 141–150, DOI:10.1016/j.bej.2018.12.022.
- K. Li, J. Wang, Y. He, G. Cui, M. A. Abdulrazaq and Y. Yan, Enhancing Enzyme Activity and Enantioselectivity of Burkholderia Cepacia Lipase via Immobilization on Melamine-Glutaraldehyde Dendrimer Modified Magnetic Nanoparticles, Chem. Eng. J., 2018, 351, 258–268, DOI:10.1016/j.cej.2018.06.086.
- J. L. Norris, T. Patel, A. K. R. Dasari, T. A. Cope, K. H. Lim and R. M. Hughes, Covalent and Non-Covalent Strategies for the Immobilization of Tobacco Etch Virus Protease (TEVp) on Superparamagnetic Nanoparticles, J. Biotechnol., 2020, 322, 1–9, DOI:10.1016/j.jbiotec.2020.06.021.
- M. S. Sadjadi, N. Farhadyar and K. Zare, Improvement of the Alkaline Protease Properties via Immobilization on the TiO2 Nanoparticles Supported by Mesoporous MCM-41, Superlattices Microstruct., 2009, 46, 77–83, DOI:10.1016/j.spmi.2008.10.022.
- G. Murugappan and K. J. Sreeram, Nano-Biocatalyst: Bi-Functionalization of Protease and Amylase on Copper Oxide Nanoparticles, Colloids Surf., B, 2021, 197, 111386, DOI:10.1016/j.colsurfb.2020.111386.
- A. S. S. Ibrahim, Y. B. Elbadawi, A. M. El-Toni, K. S. Almaary, M. A. El-Tayeb, A. A. Elagib and D. A. F. Maany, Stabilization and Improved Properties of Salipaludibacillus Agaradhaerens Alkaline Protease by Immobilization onto Double Mesoporous Core-Shell Nanospheres, Int. J. Biol. Macromol., 2021, 166, 557–566, DOI:10.1016/j.ijbiomac.2020.10.213.
- M. Razzaghi, A. Homaei and E. Mosaddegh, Penaeus Vannamei Protease Stabilizing Process of ZnS Nanoparticles, Int. J. Biol. Macromol., 2018, 112, 509–515, DOI:10.1016/j.ijbiomac.2018.01.173.
- W. Xie and X. Zang, Covalent Immobilization of Lipase onto Aminopropyl-Functionalized Hydroxyapatite-Encapsulated-γ-Fe2O3 Nanoparticles: A Magnetic Biocatalyst for Interesterification of Soybean Oil, Food Chem., 2017, 227, 397–403, DOI:10.1016/j.foodchem.2017.01.082.
- K. Pashangeh, M. Akhond, H. R. Karbalaei-Heidari and G. Absalan, Biochemical Characterization and Stability Assessment of Rhizopus Oryzae Lipase Covalently Immobilized on Amino-Functionalized Magnetic Nanoparticles, Int. J. Biol. Macromol., 2017, 105, 300–307, DOI:10.1016/j.ijbiomac.2017.07.035.
- H. Suo, Z. Gao, L. Xu, C. Xu, D. Yu, X. Xiang, H. Huang and Y. Hu, Synthesis of Functional Ionic Liquid Modified Magnetic Chitosan Nanoparticles for Porcine Pancreatic Lipase Immobilization, Mater. Sci. Eng. C, 2019, 96, 356–364, DOI:10.1016/j.msec.2018.11.041.
- M. Sarno, M. Iuliano, M. Polichetti and P. Ciambelli, High Activity and Selectivity Immobilized Lipase on Fe3O4 Nanoparticles for Banana Flavour Synthesis, Process Biochem., 2017, 56, 98–108, DOI:10.1016/j.procbio.2017.02.004.
- R. Joshi, R. Sharma and A. Kuila, Lipase Production from Fusarium Incarnatum KU377454 and Its Immobilization Using Fe3O4 NPs for Application in Waste Cooking Oil Degradation, Bioresour. Technol. Rep., 2019, 5, 134–140, DOI:10.1016/j.biteb.2019.01.005.
- B. Thangaraj, Z. Jia, L. Dai, D. Liu and W. Du, Effect of Silica Coating on Fe3O4 Magnetic Nanoparticles for Lipase Immobilization and Their Application for Biodiesel Production, Arabian J. Chem., 2019, 12, 4694–4706, DOI:10.1016/j.arabjc.2016.09.004.
- G. Khoobbakht, K. Kheiralipour, W. Yuan, M. R. Seifi and M. Karimi, Desirability Function Approach for Optimization of Enzymatic Transesterification Catalyzed by Lipase Immobilized on Mesoporous Magnetic Nanoparticles, Renewable Energy, 2020, 158, 253–262, DOI:10.1016/j.renene.2020.05.087.
- C. Miao, L. Yang, Z. Wang, W. Luo, H. Li, P. Lv and Z. Yuan, Lipase Immobilization on Amino-Silane Modified Superparamagnetic Fe3O4 Nanoparticles as Biocatalyst for Biodiesel Production, Fuel, 2018, 224, 774–782, DOI:10.1016/j.fuel.2018.02.149.
- M. Kalantari, M. Kazemeini and A. Arpanaei, Evaluation of Biodiesel Production Using Lipase Immobilized on Magnetic Silica Nanocomposite Particles of Various Structures, Biochem. Eng. J., 2013, 79, 267–273, DOI:10.1016/j.bej.2013.09.001.
- F. Esmi, T. Nematian, Z. Salehi, A. A. Khodadadi and A. K. Dalai, Amine and Aldehyde Functionalized Mesoporous Silica on Magnetic Nanoparticles for Enhanced Lipase Immobilization, Biodiesel Production, and Facile Separation, Fuel, 2021, 291, 120126, DOI:10.1016/j.fuel.2021.120126.
- A. Zulfiqar, M. W. Mumtaz, H. Mukhtar, J. Najeeb, A. Irfan, S. Akram, T. Touqeer and G. Nabi, Lipase-PDA-TiO2 NPs: An Emphatic Nano-Biocatalyst for Optimized Biodiesel Production from Jatropha Curcas Oil, Renewable Energy, 2021, 169, 1026–1037, DOI:10.1016/j.renene.2020.12.135.
- S. Martínez Cuesta, S. A. Rahman, N. Furnham and J. M. Thornton, The Classification and Evolution of Enzyme Function, Biophys. J., 2015, 109, 1082–1086, DOI:10.1016/j.bpj.2015.04.020.
- Z. Tao, B. Dong, Z. Teng and Y. Zhao, The Classification of Enzymes by Deep Learning, IEEE Access, 2020, 8, 89802–89811, DOI:10.1109/ACCESS.2020.2992468.
- P. E. V. Paul, V. Sangeetha and R. G. Deepika, Emerging trends in the industrial production of chemical products by microorganisms, in Recent Developments in Applied Microbiology and Biochemistry, ed. V. Buddolla, MA: Academic Press, Cambridge, 2019, pp. 107–125, DOI:10.1007/10_2016_10.
- Q. Li, F. Hu, M. Wang, B. Zhu, F. Ni and Z. Yao, Elucidation of Degradation Pattern and Immobilization of a Novel Alginate Lyase for Preparation of Alginate Oligosaccharides, Int. J. Biol. Macromol., 2020, 146, 579–587, DOI:10.1016/j.ijbiomac.2019.12.238.
- I. Alkorta, C. Garbisu, J. L. María and J. L. Serra, Immobilization of Pectin Lyase from Penicillium Italicum by Covalent Binding to Nylon, Enzyme Microb. Technol., 1996, 18, 141–146, DOI:10.1016/0141-0229(95)00094-1.
- M. M. Heberling, B. Wu, S. Bartsch and D. B. Janssen, Priming Ammonia Lyases and Aminomutases for Industrial and Therapeutic Applications, Curr. Opin. Chem. Biol., 2013, 17, 250–260, DOI:10.1016/j.cbpa.2013.02.013.
- G. Song, J. Sun, M. Zhao, Z. Wang, Q. Gong and W. Yu, Cloning and Characterization of Two Chondroitin Sulfate ABC Lyases from Edwardsiella Tarda LMG2793, Enzyme Microb. Technol., 2021, 143, 109701, DOI:10.1016/j.enzmictec.2020.109701.
- Z. Jiang, X. Zhang, L. Wu, H. Li, Y. Chen, L. Li, H. Ni, Q. Li and Y. Zhu, Exolytic Products of Alginate by the Immobilized Alginate Lyase Confer Antioxidant and Antiapoptotic Bioactivities in Human Umbilical Vein Endothelial Cells, Carbohydr. Polym., 2021, 251, 116976, DOI:10.1016/j.carbpol.2020.116976.
- S. Chakraborty, T. Jagan Mohan Rao and A. Goyal, Immobilization of Recombinant Pectate Lyase from Clostridium Thermocellum ATCC-27405 on Magnetic Nanoparticles for Bioscouring of Cotton Fabric, Biotechnol. Prog., 2017, 33, 236–244, DOI:10.1002/btpr.2379.
- B. Tural, S. Tural, E. Ertaş, I. Yalinkiliç and A. S. Demir, Purification and Covalent Immobilization of Benzaldehyde Lyase with Heterofunctional Chelate-Epoxy Modified Magnetic Nanoparticles and Its Carboligation Reactivity, J. Mol. Catal. B: Enzym., 2013, 95, 41–47, DOI:10.1016/j.molcatb.2013.05.023.
- A. Mukhopadhyay, T. Bhattacharyya, A. K. Dasgupta and K. Chakrabarti, Nanotechnology Based Activation-Immobilization of Psychrophilic Pectate Lyase: A Novel Approach towards Enzyme Stabilization and Enhanced Activity, J. Mol. Catal. B: Enzym., 2015, 119, 54–63, DOI:10.1016/j.molcatb.2015.05.017.
- B. Sun, Z. Wang, X. Wang, M. Qiu, Z. Zhang, Z. Wang, J. Cui and S. Jia, Paper-Based Biosensor Based on Phenylalnine Ammonia Lyase Hybrid Nanoflowers for Urinary Phenylalanine Measurement, Int. J. Biol. Macromol., 2021, 166, 601–610, DOI:10.1016/j.ijbiomac.2020.10.218.
- N. K. Ozdemir, J. P. Cline, C. J. Kiely, S. McIntosh and M. A. Snyder, Scalable Biomineralization of CdS Quantum Dots by Immobilized Cystathionine γ-Lyase, ACS Sustain. Chem. Eng., 2020, 8, 15189–15198, DOI:10.1021/acssuschemeng.0c04600.
- E. Tasgin, A. Babagil and H. Nadaroglu, Immobilization
of Purified Pectin Lyase from Acinetobacter Calcoaceticus onto Magnetic Carboxymethyl Cellulose Nanoparticles and Its Usability in Food Industry, J. Chem., 2020, 2020(61), 12, DOI:10.1155/2020/4791408.
- F. Xu, X. L. Chen, X. H. Sun, F. Dong, C. Y. Li, P. Y. Li, H. Ding, Y. Chen, Y. Z. Zhang and P. Wang, Structural and Molecular Basis for the Substrate Positioning Mechanism of a New PL7 Subfamily Alginate Lyase from the Arctic, J. Biol. Chem., 2020, 295, 16380–16392, DOI:10.1074/jbc.RA120.015106.
- B. Zhu and H. Yin, Alginate Lyase: Review of Major Sources and Classification, Properties, Structure-Function Analysis and Applications, Bioengineered, 2015, 6, 125–131, DOI:10.1080/21655979.2015.1030543.
- Y. Wang, X. Chen, X. Bi, Y. Ren, Q. Han, Y. Zhou, Y. Han, R. Yao and S. Li, Characterization of an Alkaline Alginate Lyase with PH-Stable and Thermo-Tolerance Property, Mar. Drugs, 2019, 17, 1–14, DOI:10.3390/md17050308.
- J. W. Shin, S. H. Choi, D. E. Kim, H. S. Kim, J. H. Lee, I. S. Lee and E. Y. Lee, Heterologous Expression of an Alginate Lyase from Streptomyces Sp. ALG-5 in Escherichia Coli and Its Use for Preparation of the Magnetic Nanoparticle-Immobilized Enzymes, Bioprocess Biosyst. Eng., 2011, 34, 113–119, DOI:10.1007/s00449-010-0452-4.
- Z. ling Tian, H. li Shi, M. Maria and J. wu Zheng, Pectate Lyase Is a Factor in the Adaptability for Heterodera Glycines Infecting Tobacco, J. Integr. Agric., 2019, 18, 618–626, DOI:10.1016/S2095-3119(18)62090-8.
- N. Hugouvieux-Cotte-Pattat, G. Condemine and V. E. Shevchik, Bacterial Pectate Lyases, Structural and Functional Diversity, Environ. Microbiol. Rep., 2014, 6, 427–440, DOI:10.1111/1758-2229.12166.
- S. Uluisik and G. B. Seymour, Pectate Lyases: Their Role in Plants and Importance in Fruit Ripening, Food Chem., 2020, 309, 125559, DOI:10.1016/j.foodchem.2019.125559.
- J. Kamijo, K. Sakai, H. Suzuki, K. Suzuki, E. Kunitake, M. Shimizu and M. Kato, Identification and Characterization of a Thermostable Pectate Lyase from Aspergillus Luchuensis Var. Saitoi, Food Chem., 2019, 276, 503–510, DOI:10.1016/j.foodchem.2018.10.059.
- H. J. Roh, P. Kim, Y. C. Park and J. H. Choi, Bioconversion of D-Galactose into D-Tagatose by Expression of L-Arabinose Isomerase, Biotechnol. Appl. Biochem., 2000, 31, 1–4, DOI:10.1042/BA19990065.
- S. O. Serna-Saldivar, Maize: foods from maize, Reference Module in Food Science, 2016, DOI:10.1016/B978-0-08-100596-5.00126-8.
- A. I. Soares Moretti and F. R. Martins Laurindo, Protein Disulfide Isomerases: Redox Connections in and out of the Endoplasmic Reticulum, Arch. Biochem. Biophys., 2017, 617, 106–119, DOI:10.1016/J.ABB.2016.11.007.
- A. J. Furth, J. D. Milman, J. D. Priddle and R. E. Offord, Studies on the Subunit Structure and Amino Acid Sequence of Triose Phosphate Isomerase from Chicken Breast Muscle, Biochem. J., 1974, 139, 11–22, DOI:10.1042/BJ1390011.
- H. J. Roh, P. Kim, Y. C. Park and J. H. Choi, Bioconversion of D-Galactose into D-Tagatose by Expression of L-Arabinose Isomerase, Biotechnol. Appl. Biochem., 2000, 31, 1–4, DOI:10.1042/BA19990065.
- M. L. Cacicedo, R. M. Manzo, S. Municoy, H. L. Bonazza, G. A. Islan, M. Desimone, M. Bellino, E. J. Mammarella and G. R. Castro, Chapter 7 – Immobilized enzymes and their applications, in Advances in Enzyme Technology, ed. R. S. Singh, R. R. Singhania, A. Pandey and C. Larroche, Elsevier, 2019, Biomass, Biofuels, Biochemicals, pp. 169–200 Search PubMed.
- M. L. Verma, S. Kumar, A. Das, J. S. Randhawa and M. Chamundeeswari, Chitin and Chitosan-Based Support Materials for Enzyme Immobilization and Biotechnological Applications, Environ. Chem. Lett., 2020, 18, 315–323, DOI:10.1007/s10311-019-00942-5.
- M. L. Verma, S. Kumar, D. Anamika, J. S. Randhawa and M. Chamundeeswari, Enzyme immobilization on chitin and chitosan-based supports for biotechnological applications, in Sustainable Agriculture Reviews 35: Chitin and Chitosan: History, Fundamentals and Innovations, ed. G. Crini and E. Lichtfouse, Springer International Publishing, Cham, 2019, pp. 147–173 Search PubMed.
- M. G. Gänzle, G. Haase and P. Jelen, Lactose: Crystallization, Hydrolysis and Value-Added Derivatives, Int. Dairy J., 2008, 18, 685–694, DOI:10.1016/j.idairyj.2008.03.003.
- K. Li, M. W. Woo, H. Patel, L. Metzger and C. Selomulya, Improvement of Rheological and Functional Properties of Milk Protein Concentrate by Hydrodynamic Cavitation, J. Food Eng., 2018, 221, 106–113, DOI:10.1016/j.jfoodeng.2017.10.005.
- T. Fujimaru, J.-H. Park and J. Lim, Sensory Characteristics and Relative Sweetness of Tagatose and Other Sweeteners, J. Food Sci., 2012, 77, S323–S328, DOI:10.1111/j.1750-3841.2012.02844.x.
- S. Köpper and S. Freimund, The Composition of Keto Aldoses in Aqueous Solution as Determined by NMR Spectroscopy, Helv. Chim. Acta, 2003, 86, 827–843, DOI:10.1002/hlca.200390083.
- C. Liu, J. M. Carraher, J. L. Swedberg, C. R. Herndon, C. N. Fleitman and J.-P. Tessonnier, Selective Base-Catalyzed Isomerization of Glucose to Fructose, ACS Catal., 2014, 4, 4295–4298, DOI:10.1021/cs501197w.
- J. Dijkmans, D. Gabriëls, M. Dusselier, F. de Clippel, P. Vanelderen, K. Houthoofd, A. Malfliet, Y. Pontikes and B. F. Sels, Productive Sugar Isomerization with Highly Active Sn in Dealuminated β Zeolites, Green Chem., 2013, 15, 2777–2785, 10.1039/C3GC41239C.
- J. Hajek, D. Yu. Murzin, T. Salmi and J.-P. Mikkola, Interconversion of Lactose to Lactulose in Alkaline Environment: Comparison of Different Catalysis Concepts, Top. Catal., 2013, 56, 839–845, DOI:10.1007/s11244-013-0044-z.
- S. Cheng, L. E. Metzger and S. I. Martínez-Monteagudo, One-Pot Synthesis of Sweetening Syrup from Lactose, Sci. Rep., 2020, 10, 2730, DOI:10.1038/s41598-020-59704-x.
- D. Vasanti and M. Rao, Glucose isomerase, in Enzyme Technology, ed. A. Pandey, C. Webb, C. R. Soccol and C. Larroche, Springer, New York, New York, NY, 2006, pp. 239–252 Search PubMed.
- C. Liu, J. M. Carraher, J. L. Swedberg, C. R. Herndon, C. N. Fleitman and J.-P. Tessonnier, Selective Base-Catalyzed Isomerization of Glucose to Fructose, ACS Catal., 2014, 4, 4295–4298, DOI:10.1021/cs501197w.
- S. O. Serna-Saldivar, Maize: foods from maize, in Reference Module in Food Science, Elsevier, 2016 Search PubMed.
- S. H. Bhosale, M. B. Rao and V. V. Deshpande, Molecular and Industrial Aspects of Glucose Isomerase, Microbiol. Rev., 1996, 60, 280–300, DOI:10.1128/mr.60.2.280-300.1996.
- D. Agyei, B. K. Shanbhag and L. He, 11 – Enzyme engineering (immobilization) for food applications, in Improving and Tailoring Enzymes for Food Quality and Functionality, ed. R. Y. Yada, Woodhead Publishing Series in Food Science, Technology and Nutrition, Woodhead Publishing, 2015, pp. 213–235 Search PubMed.
- M. Amaral-Fonseca, R. Morellon-Sterling, R. Fernández-Lafuente and P. W. Tardioli, Optimization of Simultaneous Saccharification and Isomerization of Dextrin to High Fructose Syrup Using a Mixture of Immobilized Amyloglucosidase and Glucose Isomerase, Catal. Today, 2021, 362, 175–183, DOI:10.1016/j.cattod.2020.03.021.
- F. R. M. Laurindo, L. A. Pescatore and D. de Castro Fernandes, Protein Disulfide Isomerase in Redox Cell Signaling and Homeostasis, Free Radical Biol. Med., 2012, 52, 1954–1969, DOI:10.1016/j.freeradbiomed.2012.02.037.
- A. I. Soares Moretti and F. R. Martins Laurindo, Protein Disulfide Isomerases: Redox Connections in and out of the Endoplasmic Reticulum, Arch. Biochem. Biophys., 2017, 617, 106–119, DOI:10.1016/j.abb.2016.11.007.
- R. Flaumenhaft and B. Furie, Vascular Thiol Isomerases, Blood, 2016, 128, 893–901, DOI:10.1182/blood-2016-04-636456.
- R. H. Bekendam and R. Flaumenhaft, Inhibition of Protein Disulfide Isomerase in Thrombosis, Basic Clin. Pharmacol. Toxicol., 2016, 119, 42–48, DOI:10.1111/bcpt.12573.
- H. Zhao, Q. Cui, V. Shah, J. Xu and T. Wang, Enhancement of Glucose Isomerase Activity by Immobilizing on Silica/Chitosan Hybrid Microspheres, J. Mol. Catal. B: Enzym., 2016, 126, 18–23, DOI:10.1016/j.molcatb.2016.01.013.
- A. J. Furth, J. D. Milman, J. D. Priddle and R. E. Offord, Studies on the Subunit Structure and Amino Acid Sequence of Trisoe Phosphate Isomerase from Chicken Breast Muscle, Biochem. J., 1974, 139, 11–22, DOI:10.1042/bj1390011.
- M. P. Esnouf, R. P. Harris and J. D. McVittie, [98] Triosephosphate isomerase from chicken and rabbit muscle, in Carbohydrate Metabolism – Part D; Methods in Enzymology, Academic Press, 1982, vol. 89, pp. 579–583 Search PubMed.
- C. A. Browne, I. D. Campbell, P. A. Kiener, D. C. Phillips, S. G. Waley and I. A. Wilson, Studies of the Histidine Residues of Triose Phosphate Isomerase by Proton Magnetic Resonance and X-Ray Crystallography, J. Mol. Biol., 1976, 100, 319–343, DOI:10.1016/S0022-2836(76)80066-6.
- H. Oshima, I. Kimura and K. Izumori, Psicose Contents in Various Food Products and Its Origin, Food Sci. Technol. Res., 2006, 12, 137–143, DOI:10.3136/fstr.12.137.
- T. Matsuo, Y. Baba, M. Hashiguchi, K. Takeshita, K. Izumori and H. Suzuki, Dietary D-Psicose, a C-3 Epimer of D-Fructose, Suppresses the Activity of Hepatic Lipogenic Enzymes in Rats, Asia Pac. J. Clin. Nutr., 2001, 10, 233–237, DOI:10.1046/j.1440-6047.2001.00246.x.
- H. Itoh, H. Okaya, A. R. Khan, S. Tajima, S. Hayakawa and K. Izumori, Purification and Characterization of D-Tagatose 3-Epimerase from Pseudomonas Sp. ST-24, Biosci., Biotechnol., Biochem., 1994, 58, 2168–2171, DOI:10.1271/bbb.58.2168.
- S. R. Dedania, M. J. Patel, D. M. Patel, R. C. Akhani and D. H. Patel, Immobilization on Graphene Oxide Improves the Thermal Stability and Bioconversion Efficiency of D-Psicose 3-Epimerase for Rare Sugar Production, Enzyme Microb. Technol., 2017, 107, 49–56, DOI:10.1016/j.enzmictec.2017.08.003.
- G. Devanand Venkatasubbu, S. Ramasamy, V. Ramakrishnan and J. Kumar, Folate Targeted PEGylated Titanium Dioxide Nanoparticles as a Nanocarrier for Targeted Paclitaxel Drug Delivery, Adv. Powder Technol., 2013, 24, 947–954, DOI:10.1016/j.apt.2013.01.008.
- X. Wang, Z. Chen, K. Li, X. Wei, Z. Chen, J. M. Ruso, Z. Tang and Z. Liu, The Study of Titanium Dioxide Modification by Glutaraldehyde and Its Application of Immobilized Penicillin Acylase, Colloids Surf., A, 2019, 560, 298–305, DOI:10.1016/j.colsurfa.2018.10.001.
- S. R. Dedania, V. K. Patel, S. S. Soni and D. H. Patel, Immobilization of Agrobacterium Tumefaciens D-Psicose 3-Epimerase onto Titanium Dioxide for Bioconversion of Rare Sugar, Enzyme Microb. Technol., 2020, 140, 109605, DOI:10.1016/j.enzmictec.2020.109605.
- J. H. Crowe, L. M. Crowe and D. Chapman, Preservation of Membranes in Anhydrobiotic Organisms: The Role of Trehalose, Science, 1984, 223, 701–703, DOI:10.1126/science.223.4637.701.
- E. P. Feofilova, A. I. Usov, I. S. Mysyakina and G. A. Kochkina, Trehalose: Chemical Structure, Biological Functions, and Practical Application, Microbiology, 2014, 83, 184–194, DOI:10.1134/S0026261714020064.
- S. Leekumjorn and A. K. Sum, Molecular Investigation of the Interactions of Trehalose with Lipid Bilayers of DPPC, DPPE and Their Mixture, Mol. Simul., 2006, 32, 219–230, DOI:10.1080/08927020600586565.
- L. Zhu, B. Shen, Z. Song and L. Jiang, Permeabilized TreS-Expressing Bacillus Subtilis Cells Decorated with Glucose Isomerase and a Shell of ZIF-8 as a Reusable Biocatalyst for the Coproduction of Trehalose and Fructose, J. Agric. Food Chem., 2020, 68, 4464–4472, DOI:10.1021/acs.jafc.0c00971.
- B. Thangaraj, P. R. Solomon, B. Muniyandi, S. Ranganathan and L. Lin, Catalysis in Biodiesel Production—a Review, Clean Energy, 2019, 3, 2–23, DOI:10.1093/ce/zky020.
- B. Changmai, C. Vanlalveni, A. P. Ingle, R. Bhagat and S. L. Rokhum, Widely Used Catalysts in Biodiesel Production: A Review, RSC Adv., 2020, 10, 41625–41679, 10.1039/D0RA07931F.
- C. L. B. Reis, E. Y. A. de Sousa, J. de França Serpa, R. C. Oliveira and J. C. S. Dos Santos, Design of Immobilized Enzyme Biocatalysts: Drawbacks and Opportunities, Quim. Nova, 2019, 42, 768–783 CAS.
- T. Carvalho, S. da, A. Pereira, R. C. F. Bonomo, M. Franco, P. V. Finotelli and P. F. F. Amaral, Simple Physical Adsorption Technique to Immobilize Yarrowia Lipolytica Lipase Purified by Different Methods on Magnetic Nanoparticles: Adsorption Isotherms and Thermodynamic Approach, Int. J. Biol. Macromol., 2020, 160, 889–902, DOI:10.1016/j.ijbiomac.2020.05.174.
- N. S. Mohammadi, M. S. Khiabani, B. Ghanbarzadeh and R. R. Mokarram, Improvement of Lipase Biochemical Properties via a Two-Step Immobilization Method: Adsorption onto Silicon Dioxide Nanoparticles and Entrapment in a Polyvinyl Alcohol/Alginate Hydrogel, J. Biotechnol., 2020, 323, 189–202, DOI:10.1016/j.jbiotec.2020.07.002.
- Y. Liu, T. Liu, X. Wang, L. Xu and Y. Yan, Biodiesel Synthesis Catalyzed by Burkholderia Cenocepacia Lipase Supported on Macroporous Resin NKA in Solvent-Free and Isooctane Systems, Energy Fuels, 2011, 25, 1206–1212, DOI:10.1021/ef200066x.
- X. Li, L. Xu, G. Wang, H. Zhang and Y. Yan, Conformation Studies on Burkholderia Cenocepacia Lipase via Resolution of Racemic 1-Phenylethanol in Non-Aqueous Medium and Its Process Optimization, Process Biochem., 2013, 48, 1905–1913, DOI:10.1016/j.procbio.2013.09.001.
- K. Li, J. Wang, Y. He, G. Cui, M. A. Abdulrazaq and Y. Yan, Enhancing Enzyme Activity and Enantioselectivity of Burkholderia Cepacia Lipase via Immobilization on Melamine-Glutaraldehyde Dendrimer Modified Magnetic Nanoparticles, Chem. Eng. J., 2018, 351, 258–268, DOI:10.1016/j.cej.2018.06.086.
- H. Zaak, M. Sassi and R. Fernandez-Lafuente, A New Heterofunctional Amino-Vinyl Sulfone Support to Immobilize Enzymes: Application to the Stabilization of β-Galactosidase from Aspergillus Oryzae, Process Biochem., 2018, 64, 200–205, DOI:10.1016/j.procbio.2017.09.020.
- J. J. Virgen-Ortíz, J. C. S. dos Santos, Á. Berenguer-Murcia, O. Barbosa, R. C. Rodrigues and R. Fernandez-Lafuente, Polyethylenimine: A Very Useful Ionic Polymer in the Design of Immobilized Enzyme Biocatalysts, J. Mater. Chem. B, 2017, 5, 7461–7490, 10.1039/C7TB01639E.
- H. Vaghari, H. Jafarizadeh-Malmiri, M. Mohammadlou, A. Berenjian, N. Anarjan, N. Jafari and S. Nasiri, Application of Magnetic Nanoparticles in Smart Enzyme Immobilization, Biotechnol. Lett., 2016, 38, 223–233, DOI:10.1007/s10529-015-1977-z.
- T. L. de Albuquerque, N. Rueda, J. C. S. dos Santos, O. Barbosa, C. Ortiz, B. Binay, E. Özdemir, L. R. B. Gonçalves and R. Fernandez-Lafuente, Easy Stabilization of Interfacially Activated Lipases Using Heterofunctional Divinyl Sulfone Activated-Octyl Agarose Beads. Modulation of the Immobilized Enzymes by Altering Their Nanoenvironment, Process Biochem., 2016, 51, 865–874, DOI:10.1016/j.procbio.2016.04.002.
- J. J. Virgen-Ortíz, J. C. S. dos Santos, Á. Berenguer-Murcia, O. Barbosa, R. C. Rodrigues and R. Fernandez-Lafuente, Polyethylenimine: A Very Useful Ionic Polymer in the Design of Immobilized Enzyme Biocatalysts, J. Mater. Chem. B, 2017, 5, 7461–7490, 10.1039/C7TB01639E.
- Z. Huang, K. He, Z. Song, G. Zeng, A. Chen, L. Yuan, H. Li, L. Hu, Z. Guo and G. Chen, Antioxidative Response of Phanerochaete Chrysosporium against Silver Nanoparticle-Induced Toxicity and Its Potential Mechanism, Chemosphere, 2018, 211, 573–583, DOI:10.1016/j.chemosphere.2018.07.192.
- R. M. Bezerra, R. R. C. Monteiro, D. M. A. Neto, F. F. M. da Silva, R. C. M. de Paula, T. L. G. de Lemos, P. B. A. Fechine, M. A. Corrêa, F. Bohn and L. R. B. Gonçalves, et al., A New Heterofunctional Support for Enzyme Immobilization: PEI Functionalized Fe3O4 MNPs Activated with Divinyl Sulfone. Application in the Immobilization of Lipase from Thermomyces Lanuginosus, Enzyme Microb. Technol., 2020, 138, 109560 CrossRef CAS PubMed.
- J. Yang, K. Ni, D. Wei and Y. Ren, One-Step Purification and Immobilization of His-Tagged Protein via Ni2+-Functionalized Fe3O4@polydopamine Magnetic Nanoparticles, Biotechnol. Bioprocess Eng., 2015, 20, 901–907, DOI:10.1007/s12257-015-0136-7.
- C.-T. Chen, W.-J. Chen, C.-Z. Liu, L.-Y. Chang and Y.-C. Chen, Glutathione-Bound Gold Nanoclusters for Selective-Binding and Detection of Glutathione S-Transferase-Fusion Proteins from Cell Lysates, Chem. Commun., 2009, 7515–7517, 10.1039/B916919A.
- J. Castillo, L. Bertel, E. Páez-Mozo and F. Martínez, Photochemical synthesis of the bioconjugate folic acid-gold nanoparticles, Nanomater. Nanotechnol., 2013, 3, 132–145 Search PubMed.
- Z. Zhang, J. Jia, Y. Lai, Y. Ma, J. Weng and L. Sun, Conjugating Folic Acid to Gold Nanoparticles through Glutathione for Targeting and Detecting Cancer Cells, Bioorg. Med. Chem., 2010, 18, 5528–5534, DOI:10.1016/j.bmc.2010.06.045.
- W.-C. Kuan, J.-W. Lai and W.-C. Lee, Covalent Binding of Glutathione on Magnetic Nanoparticles: Application for Immobilizing Small Fragment Ubiquitin-like-Specific Protease 1, Enzyme Microb. Technol., 2021, 143, 109697, DOI:10.1016/j.enzmictec.2020.109697.
- C. D. Spicer, C. Jumeaux, B. Gupta and M. M. Stevens, Peptide and Protein Nanoparticle Conjugates: Versatile Platforms for Biomedical Applications, Chem. Soc. Rev., 2018, 47, 3574–3620, 10.1039/C7CS00877E.
- A. M. M. Brito, E. Belleti, L. R. Menezes, A. J. C. Lanfredi and I. L. Nantes-Cardoso, Proteins and Peptides at the Interfaces of Nanostructures, An. Acad. Bras. Cienc., 2019, 91(4), e20181236, DOI:10.1590/0001-3765201920181236.
- S. Dutta, S. Saravanabhupathy, R. C. Rajak, R. Banerjee, P. K. Dikshit, C. T. Padigala, A. K. Das and B. S. Kim, Recent Developments in Lignocellulosic Biofuel Production with Nanotechnological Intervention: An Emphasis on Ethanol, Catalysts, 2023, 13, 11, DOI:10.3390/catal13111439.
- E. Zanuso, H. A. Ruiz, L. Domingues and J. A. Teixeira, Magnetic Nanoparticles as Support for Cellulase Immobilization Strategy for Enzymatic Hydrolysis Using Hydrothermally Pretreated Corn Cob Biomass, BioEnergy Res., 2022, 15, 1946–1957, DOI:10.1007/s12155-021-10384-z.
- L. F. Peffi Ferreira, T. Mazzi De Oliveira, S. H. Toma, M. M. Toyama, K. Araki and L. H. Avanzi, Superparamagnetic Iron Oxide Nanoparticles (SPIONs) Conjugated with Lipase: Candida Antarctica A for Biodiesel Synthesis, RSC Adv., 2020, 10, 38490–38496, 10.1039/d0ra06215d.
- B. M. C. de Araújo, I. O. Costa, H. G. de Brito, N. S. Rios and E. S. dos Santos, Enzyme Technology in Bioethanol Production from Lignocellulosic Biomass: Recent Trends with a Focus on Immobilized Enzymes, Bioresources, 2023, 8653–8687 Search PubMed.
- M. V. C. da Silva, A. B. S. Rangel, C. M. R. Rosa, G. P. de Assis, L. G. Aguiar and L. de Freitas, Development of a Magnetically Stabilized Fluidized Bed Bioreactor for Enzymatic Synthesis of 2-Ethylhexyl Oleate, Bioprocess Biosyst. Eng., 2023, 46, 1665–1676, DOI:10.1007/s00449-023-02928-8.
- D. Yu, X. Ma, Y. Huang, L. Jiang, L. Wang, C. Han and F. Yang, Immobilization of Cellulase on Magnetic Nanoparticles for Rice Bran Oil Extraction in a Magnetic Fluidized Bed, Int. J. Food Eng., 2022, 18, 15–26, DOI:10.1515/ijfe-2021-0111.
- K. Chen, T. Wang, H. Wang, H. Geng, J. Du, D. Yu, W. Elfalleh, Z. Mehrez and L. Wang, Application of Magnetic Nano-Immobilized Enzyme in Soybean Oil Degumming: Numerical Simulation in a Liquid-Solid MFB, J. Food Qual., 2021, 2021, 6652780, DOI:10.1155/2021/6652780.
- N. K. Abd-Elrahman, N. Al-Harbi, Y. Al-Hadeethi, A. B. Alruqi, H. Mohammed, A. Umar and S. Akbar, Influence of Nanomaterials and Other Factors on Biohydrogen Production Rates in Microbial Electrolysis Cells—A Review, Molecules, 2022, 27, 1–24, DOI:10.3390/molecules27238594.
- A. V. Bounegru and C. Apetrei, Tyrosinase Immobilization Strategies for the Development of Electrochemical Biosensors—A Review, Nanomaterials, 2023, 13, 760, DOI:10.3390/nano13040760.
- J. Zhao, V. E. Lee, R. Liu and R. D. Priestley, Responsive Polymers as Smart Nanomaterials Enable Diverse Applications, Annu. Rev. Chem. Biomol. Eng., 2019, 10, 361–382, DOI:10.1146/annurev-chembioeng-060718-030155.
- D. Maureira, O. Romero, A. Illanes, L. Wilson and C. Ottone, Industrial Bioelectrochemistry for Waste Valorization: State of the Art and Challenges, Biotechnol. Adv., 2023, 64, 78, DOI:10.1016/j.biotechadv.2023.108123.
- D. D. Nguyen, M. Tanveer, H. N. Mai, T. Q. D. Pham, H. Khan, C. W. Park and G. M. Kim, Guiding the Optimization of Membraneless Microfluidic Fuel Cells via Explainable Artificial Intelligence: Comparative Analyses of Multiple Machine Learning Models and Investigation of Key Operating Parameters, Fuel, 2023, 349, 128742, DOI:10.1016/j.fuel.2023.128742.
- S. Bosu, R. P. Pooja and M. Rajasimman, Role of Nanomaterials in Enhanced Ethanol Production through Biological Methods – Review on Operating Factors and Machine Learning Applications, Fuel, 2022, 320, 123905, DOI:10.1016/j.fuel.2022.123905.
- J. Xu, J. Sun, Y. Wang, J. Sheng, F. Wang and M. Sun, Application of Iron Magnetic Nanoparticles in Protein Immobilization, Molecules, 2014, 19, 11465–11486, DOI:10.3390/molecules190811465.
- C. G. C. M. Netto, H. E. Toma and L. H. Andrade, Superparamagnetic Nanoparticles as Versatile Carriers and Supporting Materials for Enzymes, J. Mol. Catal. B: Enzym., 2013, 85–86, 71–92, DOI:10.1016/j.molcatb.2012.08.010.
- Y. Habibi, L. A. Lucia and O. J. Rojas, Cellulose Nanocrystals: Chemistry, Self-Assembly, and Applications, Chem. Rev., 2010, 110, 3479–3500, DOI:10.1021/cr900339w.
- S.-H. Huang and R.-S. Juang, Biochemical and Biomedical Applications of Multifunctional Magnetic Nanoparticles: A Review, J. Nanopart. Res., 2011, 13, 4411–4430, DOI:10.1007/s11051-011-0551-4.
- U. T. Bornscheuer, Immobilizing Enzymes: How to Create More Suitable Biocatalysts, Angew. Chem., Int. Ed., 2003, 42, 3336–3337, DOI:10.1002/anie.200301664.
- M. Bilal, Y. Zhao, T. Rasheed and H. M. N. Iqbal, Magnetic Nanoparticles as Versatile Carriers for Enzymes Immobilization: A Review, Int. J. Biol. Macromol., 2018, 120, 2530–2544, DOI:10.1016/j.ijbiomac.2018.09.025.
- S. A. Ansari and Q. Husain, Potential Applications of Enzymes Immobilized on/in Nano Materials: A Review, Biotechnol. Adv., 2012, 30, 512–523, DOI:10.1016/j.biotechadv.2011.09.005.
- E. T. Hwang and M. B. Gu, Enzyme Stabilization by Nano/Microsized Hybrid Materials, Eng. Life Sci., 2013, 13, 49–61, DOI:10.1002/elsc.201100225.
- M. Bilal, M. Asgher, H. Cheng, Y. Yan and H.
M. N. Iqbal, Multi-Point Enzyme Immobilization, Surface Chemistry, and Novel Platforms: A Paradigm Shift in Biocatalyst Design, Crit. Rev. Biotechnol., 2019, 39, 202–219, DOI:10.1080/07388551.2018.1531822.
- C. Bernal, K. Rodríguez and R. Martínez, Integrating Enzyme Immobilization and Protein Engineering: An Alternative Path for the Development of Novel and Improved Industrial Biocatalysts, Biotechnol. Adv., 2018, 36, 1470–1480, DOI:10.1016/j.biotechadv.2018.06.002.
- P. Wang, Nanoscale Engineering for Smart Biocatalysts with Fine-Tuned Properties and Functionalities, Top. Catal., 2012, 55, 1107–1113, DOI:10.1007/s11244-012-9904-1.
- S. Ariaeenejad, E. Motamedi and G. Hosseini Salekdeh, Immobilization of Enzyme Cocktails on Dopamine Functionalized Magnetic Cellulose Nanocrystals to Enhance Sugar Bioconversion: A Biomass Reusing Loop, Carbohydr. Polym., 2021, 256, 117511, DOI:10.1016/j.carbpol.2020.117511.
- N. Grishkewich, N. Mohammed, J. Tang and K. C. Tam, Recent Advances in the Application of Cellulose Nanocrystals, Curr. Opin. Colloid Interface Sci., 2017, 29, 32–45, DOI:10.1016/j.cocis.2017.01.005.
- M. Mariano, N. El Kissi and A. Dufresne, Cellulose Nanocrystals and Related Nanocomposites: Review of Some Properties and Challenges, J. Polym. Sci., Part B: Polym. Phys., 2014, 52, 791–806, DOI:10.1002/polb.23490.
- J. George and S. N. Sabapathi, Cellulose Nanocrystals: Synthesis, Functional Properties, and Applications, Nanotechnol., Sci. Appl., 2015, 45, 45–54, DOI:10.2147/NSA.S64386.
- S. Eyley and W. Thielemans, Surface Modification of Cellulose Nanocrystals, Nanoscale, 2014, 6, 7764–7779, 10.1039/C4NR01756K.
- D. Trache, M. H. Hussin, M. K. M. Haafiz and V. K. Thakur, Recent Progress in Cellulose Nanocrystals: Sources and Production, Nanoscale, 2017, 9, 1763–1786, 10.1039/C6NR09494E.
- A. Gennari, A. J. Führ, G. Volpato and C. F. Volken de Souza, Magnetic Cellulose: Versatile Support for Enzyme Immobilization - A Review, Carbohydr. Polym., 2020, 246, 116646, DOI:10.1016/j.carbpol.2020.116646.
- L. Li, R.-Y. Bao, T. Gao, Z.-Y. Liu, B.-H. Xie, M.-B. Yang and W. Yang, Dopamine-Induced Functionalization of Cellulose Nanocrystals with Polyethylene Glycol towards Poly(L-Lactic Acid) Bionanocomposites for Green Packaging, Carbohydr. Polym., 2019, 203, 275–284, DOI:10.1016/j.carbpol.2018.09.057.
- M. Martín, P. Salazar, R. Villalonga, S. Campuzano, J. M. Pingarrón and J. L. González-Mora, Preparation of Core–Shell Fe 3 O 4 @poly(Dopamine) Magnetic Nanoparticles for Biosensor Construction, J. Mater. Chem. B, 2014, 2, 739–746, 10.1039/C3TB21171A.
- H. Kang, X. Song, Z. Wang, W. Zhang, S. Zhang and J. Li, High-Performance and Fully Renewable Soy Protein Isolate-Based Film from Microcrystalline Cellulose via Bio-Inspired Poly(Dopamine) Surface Modification, ACS Sustain. Chem. Eng., 2016, 4, 4354–4360, DOI:10.1021/acssuschemeng.6b00917.
- P. Winterwerber, S. Harvey, D. Y. W. Ng and T. Weil, Photocontrolled Dopamine Polymerization on DNA Origami with Nanometer Resolution, Angew. Chem., Int. Ed., 2020, 59, 6144–6149, DOI:10.1002/anie.201911249.
- M. Bilal, S. S. Ashraf, L. F. R. Ferreira, J. Cui, W.-Y. Lou, M. Franco and H. M. N. Iqbal, Nanostructured Materials as a Host Matrix to Develop Robust Peroxidases-Based Nanobiocatalytic Systems, Int. J. Biol. Macromol., 2020, 162, 1906–1923, DOI:10.1016/j.ijbiomac.2020.08.122.
- S. S. Nadar, P. D. Patil and N. M. Rohra, Magnetic Nanobiocatalyst for Extraction of Bioactive Ingredients: A Novel Approach, Trends Food Sci. Technol., 2020, 103, 225–238, DOI:10.1016/j.tifs.2020.07.007.
- E. P. Cipolatti, A. Valério, R. O. Henriques, D. E. Moritz, J. L. Ninow, D. M. G. Freire, E. A. Manoel, R. Fernandez-Lafuente and D. de Oliveira, Nanomaterials for Biocatalyst Immobilization – State of the Art and Future Trends, RSC Adv., 2016, 6, 104675–104692, 10.1039/C6RA22047A.
- M. Bilal and H. M. N. Iqbal, Armoring Bio-Catalysis via Structural and Functional Coordination between Nanostructured Materials and Lipases for Tailored Applications, Int. J. Biol. Macromol., 2021, 166, 818–838, DOI:10.1016/j.ijbiomac.2020.10.239.
- K. Mylkie, P. Nowak, P. Rybczynski and M. Ziegler-Borowska, Polymer-Coated Magnetite Nanoparticles for Protein Immobilization, Materials, 2021, 14, 248, DOI:10.3390/ma14020248.
- M. Singhvi and B. S. Kim, Current Developments in Lignocellulosic Biomass Conversion into Biofuels Using Nanobiotechology Approach, Energies, 2020, 13, 5300, DOI:10.3390/en13205300.
- K. Khoshnevisan, F. Vakhshiteh, M. Barkhi, H. Baharifar, E. Poor-Akbar, N. Zari, H. Stamatis and A.-K. Bordbar, Immobilization of Cellulase Enzyme onto Magnetic Nanoparticles: Applications and Recent Advances, Mol. Catal., 2017, 442, 66–73, DOI:10.1016/j.mcat.2017.09.006.
|
This journal is © The Royal Society of Chemistry 2024 |
Click here to see how this site uses Cookies. View our privacy policy here.