DOI:
10.1039/D4RA03826F
(Paper)
RSC Adv., 2024,
14, 20056-20060
Squaramide-catalyzed enantioselective Michael addition of nitromethane to 2-enoylazaarenes: synthesis of chiral azaarene-containing γ-nitroketones†
Received
24th May 2024
, Accepted 10th June 2024
First published on 21st June 2024
Abstract
Bifunctional chiral squaramide-catalyzed highly enantioselective Michael addition of nitromethane to diverse 2-enoylazaarenes was successfully performed. This protocol provided a set of chiral azaarene-containing γ-nitroketones with up to 98% yield and 98% ee in a solvent-free catalytic system under mild conditions. Furthermore, gram-scale synthetic utility was also showcased.
Introduction
N-Heteroaromatics (azaarenes) are widely prevalent in natural products, pharmaceuticals, bioactive compounds, and other functional molecules.1–6 The development of methodologies for accessing diverse azaarene derivatives has garnered significant attention. Among these strategies, there has been a growing demand for the exploration of highly enantioselective approaches to synthesize chiral compounds or intermediates that incorporate azaarene moieties. In recent decades, substantial focus has been directed towards the utilization of 2-enoylpyridines and their N-oxides for a variety of asymmetric transformations.7–30 In particular, the enantioselective Michael-type reactions catalyzed by organometallic complexes8–18 and organocatalysts19–30 have gained much more attention. Of the current catalytic systems, bifunctional chiral squaramides and (thio)ureas have emerged as undoubtedly the privileged catalysts for organocatalytic conjugate addition reactions between 2-enoylpyridines and various carbanion nucleophiles, including malononitrile, nitroalkanes, 1,3-dicarbonyl compounds, and so on (Scheme 1a).19–29 Despite extensive research on the conjugate additions of nitroalkanes as valuable carbanion nucleophiles to numerous α,β-unsaturated carbonyl compounds,31–33 there have been limited reports on the organocatalytic enantioselective Michael reactions of nitroalkanes with a range of 2-enoylazaarenes, such as 2-enoylpyridine,20,25 2-enoylpyrazine, and 2-enoylthiazole among others. Notably, Song et al. exclusively disclosed the asymmetric Michael reaction of nitromethane with β-(hetero)aryl 2-enoylpyridines promoted by the thiourea catalyst, affording the optically pure pyridine-containing γ-nitroketones with high antibacterial activities.25
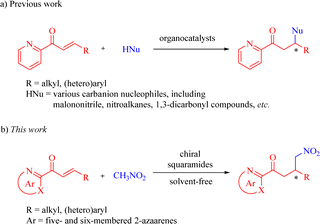 |
| Scheme 1 Organocatalytic enantioselective conjugate additions of various nucleophiles to 2-enoylazaarenes. | |
In light of the asymmetric addition reactions of 2-enoylpyridines catalyzed by chiral squaramides,21–24,27–29 we envisioned that bifunctional chiral squaramides could enhance the addition reaction of nitroalkanes to 2-enoylazaarenes, wherein nitroalkanes can be deprotonated by the catalyst's tertiary amine moiety and 2-enoyl substrates may be activated through double H-bonding interactions formed by the squaramide moiety with both the azaarene nitrogen and carbonyl oxygen (Scheme 1b). To the best of our knowledge, no investigations have been reported on the squaramide-catalyzed enantioselective additions of nitroalkanes to various 2-enoylazaarenes. As part of our unremitting research interest in chiral squaramide-promoted asymmetric reactions,34 we herein present a solvent-free enantioselective Michael reaction between nitromethane and a broad range of β-substituted 2-enoylazaarenes in the presence of chiral squaramides for the facile synthesis of diverse enantioenriched azaarene-containing γ-nitroketones.
Results and discussion
Reaction optimization
The model reaction chosen for optimizing the conditions was the Michael addition of nitromethane to β-phenyl 2-enoylpyridine 1a. The results are listed in Table 1.
Table 1 Optimization of reaction conditionsa
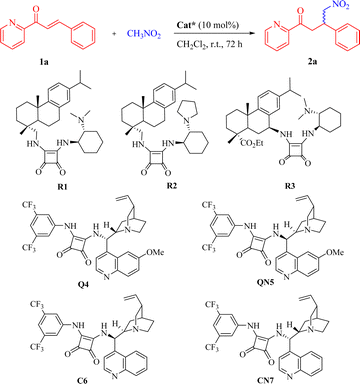
|
Entry |
Cat. |
Yieldb [%] |
eec [%] |
Configurationd |
All reactions were conducted by using 1a (0.1 mmol), nitromethane (1 mL), and the catalyst (10 mol%) in CH2Cl2 at room temperature for 72 h, unless otherwise noted. NR = no reaction. Isolated yields. Detection by chiral HPLC analysis. The absolute configuration was determined by comparing the HPLC and optical rotation data with the literature.8,25 Without CH2Cl2. In the presence of 5 mol% catalyst. At 10 °C for 144 h. At 50 °C for 48 h. At 80 °C for 48 h. |
1 |
R1 |
NR |
- |
- |
2 |
R2 |
NR |
- |
- |
3 |
R3 |
NR |
- |
- |
4 |
Q4 |
33 |
96 |
R |
5 |
QN5 |
32 |
92 |
S |
6 |
C6 |
11 |
96 |
S |
7 |
CN7 |
8 |
96 |
R |
8e |
Q4 |
98 |
98 |
R |
9e |
QN5 |
95 |
98 |
S |
10e,f |
Q4 |
94 |
96 |
R |
11e,g |
Q4 |
96 |
96 |
R |
12e,h |
Q4 |
97 |
97 |
R |
13e,i |
Q4 |
96 |
96 |
R |
Initially, inspired by the application of bifunctional organocatalysts derived from natural rosin in asymmetric Michael-type reactions,34 we anticipated their potential to facilitate the model reaction. Nevertheless, the expected transformation was not achieved when the reaction was conducted in CH2Cl2 at room temperature in the presence of the rosin-based squaramide catalysts R1–R3 (Table 1, entries 1–3). Encouragingly, the bifunctional squaramides Q4, QN5, C6 and CN7 derived from cinchona alkaloids exhibited certain catalytic activities, and the target product 2a was generated with over 92% ee in each case, albeit at lower yields (entries 4–7). Both quinine-derived Q4 and cinchonidine-derived CN7 provided the R-configured product, while the complementary S-configured enantiomer was obtained by employing quinidine-derived QN5 or cinchonine-derived C6.8,25,35 In contrast, Q4 and QN5 demonstrated comparatively higher reactivity in this addition reaction (entries 4 and 5 vs. 6 and 7, respectively). The adoption of a solvent-free approach has been recognized as an effective optimization strategy for enhancing the efficiency of chemical processes.36 Consequently, the Michael reaction was conducted in neat nitromethane. To our delight, in a solvent-free system, both Q4 and QN5 enabled full conversion of 1a with significantly improved outcomes (entries 8 and 9 vs. 4 and 5, respectively). Considering the comparable reactivity and enantiocomplementary synthesis displayed by Q4 and QN5, we selected Q4 as the catalyst for further screening purposes (QN5 can potentially be used for generating the opposite enantiomers if required). Next, it was observed that the reactivity and enantioselectivity decreased slightly upon adjusting the catalyst loading to 5 mol% (entry 10). Additionally, when manipulating the reaction temperature within a specific range, there seemed to be an impact on the reactivity for the evident that a prolonged reaction time at the lower temperature was essential for achieving a favorable yield, but no discernible improvement in enantioselectivity was observed (entries 11–13). Thus, the optimized reaction conditions were ultimately identified as 1a (0.1 mmol), nitromethane (1 mL), Q4 (10 mol%) at room temperature.
Substrate scope
Under the optimal reaction conditions, the substrate scope of β-substituted 2-enoylazaarenes 1 was investigated by varying 2-azaarene rings (2-pyridinyl, 2-pyrazinyl, and 2-thiazolyl) and β-substituted groups (aryl, heteroaryl and alkyl), as summarized in Table 2. In general, the squaramide Q4 has demonstrated noticeable functional group tolerance in the solvent-free system for the Michael addition of various 2-enoyl substrates, affording the products 2 with yields as high as 98% and excellent enantioselectivities (up to 98% ee).
Table 2 Scope of β-substituted 2-enoylazaarenes 1a
All reactions were conducted by using 1a–1w (0.1 mmol), nitromethane (1 mL), and Q4 (10 mol%) at room temperature for the specified reaction time. |
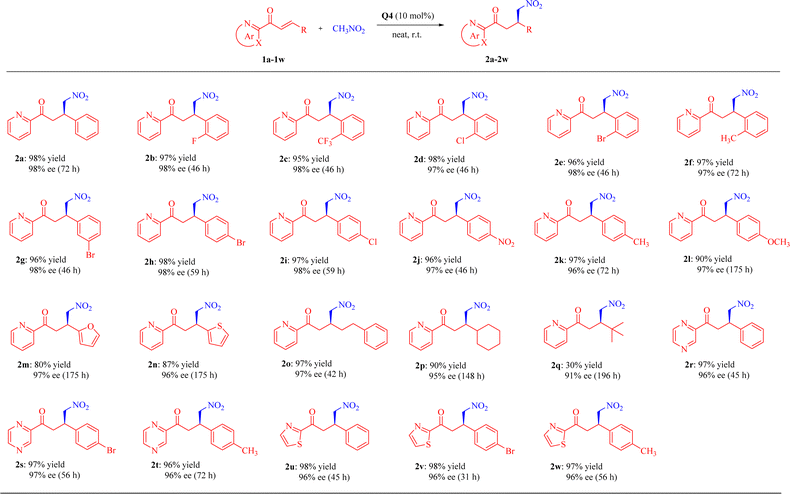 |
The conjugate addition of β-aryl and β-heteroaryl 2-enoylpyridines 1a–1n proceeded smoothly, leading to the formation of the corresponding products 2a–2n with high yields (80–98%) and excellent enantioselectivities (96–98% ees). β-Aryl 2-enoylpyridines 1a–1l showed favorable reactivities, irrespective of the electronic properties or substitution positions of either electron-withdrawing or electron-donating substituents on the phenyl ring. In comparison, the reactivity of β-heteroaryl 2-enoylpyridines 1m and 1n appeared comparatively lower.
Moreover, β-alkyl 2-enoylpyridines 1o–1q were tolerated as well, furnishing the desired Michael adducts with excellent enantioselectivities (91–97% ees), whereas the introduction of a bulky tert-butyl group on the β-position (substrate 1q) resulted in an acceptable enantiomeric excess value (91%), albeit with an unsatisfactory yield (30%), probably attributed to steric hindrance.8 The noteworthy aspect lies in the fact that β-cyclohexyl 2-enoylpyridine 1p demonstrated superior outcomes compared to those reported in the literature.35
Finally, we also evaluated the addition of 2-enoyl substrates featuring different 2-azaarenes, such as six-membered pyrazine and five-membered thiazole. β-Aryl 2-enoylpyrazines (1r–1t) and 2-enoylthiazoles (1u–1w) underwent rather well to deliver the corresponding products in high reactivities and excellent enantioselectivities (up to 98% yield and 97% ee).
Scale-up synthesis and utility
To demonstrate the synthetic applicability of the established catalytic system, we carried out the Michael reaction of 1a on a larger scale (4.8 mmol) and observed that this solvent-free catalytic system was equally efficient even using only 5 mol% Q4 to prepare the adduct 2a in high yield (86%) and excellent enantioselectivity (97% ee) (Scheme 2, the first step). The obtained γ-nitrocarbonyl pyridine derivative underwent a facile reduction of the nitro group, followed by the in situ cyclization, enabling the gram-scale synthesis of dihydro-2H-pyrrole N-oxide 3a, an analogue of nicotine,1,10 with no loss in enantioselectivity (98% ee) (Scheme 2, the second step).
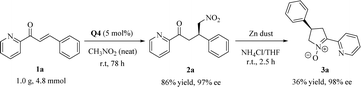 |
| Scheme 2 Gram-scale preparation experiment. | |
Proposed transition-state model
Based on these findings and previous generally accepted mechanistic studies on 2-enoylpyridines and bifunctional squaramides,19,25,29 we proposed a rational dual activation transition-state model to elucidate the observed stereochemistry, as illustrated in Scheme 3.
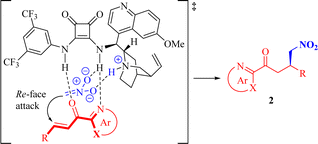 |
| Scheme 3 Possible transition-state model. | |
The squaramide moiety of the bifunctional catalyst Q4 activates 2-enoyl substrate 1 through double H-bonding interactions with both the azaarene nitrogen and the carbonyl oxygen. Simultaneously, the catalyst's tertiary amine moiety facilitates the deprotonation of nitromethane, leading to the generation of a pronucleophile, establishing a H-bond with the quinuclidine ammonium ion while forming an additional weak hydrogen-bonding interaction between the nitro oxygen and the NH group of the squaramide moiety. The subsequent 1,4-addition of this pronucleophile to the Re face (the bottom face) of the electrophile 1 results in the formation of the major enantiomer of the target product 2. It is worth mentioning that, according to our plausible activation model, the stereogenic center at the β-position to the carbonyl group should consistently possess R-configuration in all adducts, with the exception of compounds 2m and 2o, where it should be designated as S-configuration due to a change in the priority order of substituents as dictated by the Cahn–Ingold–Prelog rules.
Conclusions
In summary, we have successfully discovered an efficient squaramide-catalyzed asymmetric Michael addition of nitromethane to a range of 2-enoylazaarenes bearing β-substituted (hetero)aryl and alkyl groups under mild conditions, providing diverse enantiomerically pure azaarene-containing γ-nitroketones in high yields (80–98%) and excellent enantioselectivities (95–98% ee), except for β-tBu 2-enoylpyridine, which exhibited higher enantioselectivity (91% ee), albeit with a low reactivity (30% yield). The scale-up synthetic utility of the present solvent-free enantioselective conjugate addition reaction was accomplished by converting the resulting adduct into a nicotine analogue. Ongoing investigations in our laboratory encompass a broader substrate scope of azaarene-containing enones, as well as exploration of the biological activities of these synthesized 2-azaarene derivatives.
Data availability
The data supporting this article have been included as part of the ESI.†
Author contributions
Conceptualization and funding acquisition, Z.-W. Zhang and Y.-Q. Deng; writing—original draft preparation, H.-P. Huang, Y.-H. Xie, X.-M. Gan, X.-Y. Wen and C.-X. Wang; writing—review and editing, Z.-W. Zhang, Y.-Q. Deng and H.-P. Huang.
Conflicts of interest
There are no conflicts to declare.
Acknowledgements
Financial supports from Guangxi Natural Science Foundation (2020GXNSFAA297215 and AD19245138); Guangxi Graduate Joint Training Base (Gui Degree 2021-6); Guangxi University of Chinese Medicine ‘Qihuang Project’ High-level Talent Team Cultivation Programme (202408); Thousands of Young and Middle-aged Backbone Teachers Training Project of Guangxi Colleges and Universities (Gui-Jiao 2019-81); Guangxi University of Chinese Medicine General Program (2021MS006); Guangxi University of Chinese Medicine Graduate Education Innovation Program (YCSW2023394).
References
- D. Pogocki, T. Ruman, M. Danilczuk, M. Danilczuk, M. Celuch and E. Waajtys-Rode, Eur. J. Pharmacol., 2007, 563, 18–39 CrossRef CAS PubMed.
- R. D. Taylor, M. Maccoss and A. D. G. Lawson, J. Med. Chem., 2014, 57, 5845–5859 CrossRef CAS PubMed.
- E. Vitaku, D. T. Smith and J. T. Njardarson, J. Med. Chem., 2015, 57, 10257–10274 CrossRef PubMed.
- D. Best and H. W. Lam, J. Org. Chem., 2014, 79, 831–845 CrossRef CAS PubMed.
- R. P. Jumde, F. Lanza, M. J. Veenstra and S. R. Harutyunyan, Science, 2016, 352, 433–437 CrossRef CAS PubMed.
- U. H. F. Bunz and J. Freudenberg, Acc. Chem. Res., 2019, 52, 1575–1587 CrossRef CAS PubMed.
- G. Desimoni, G. Faita and P. Quadrelli, Chem. Rev., 2014, 114, 6081–6129 CrossRef CAS PubMed.
- G. Blay, C. Incerti, M. C. Muñoz and J. R. Pedro, Eur. J. Org Chem., 2013, 9, 1696–1705 CrossRef.
- S. Mukherjee, S. Mondal, A. Patra, R. G. Gonnade and A. T. Biju, Chem. Commun., 2015, 51, 9559–9562 RSC.
- L. Li, S. Zhang, Y. Hu, Y. Li, C. Li, Z. Zha and Z. Wang, Chem.–Eur. J., 2015, 21, 12885–12888 CrossRef CAS PubMed.
- S.-W. Li, J. Gong and Q. Kang, Org. Lett., 2017, 19, 1350–1353 CrossRef PubMed.
- L. B. Balázs, W. S. Tay, Y. Li, S. A. Pullarkat and P. H. Leung, Organometallics, 2018, 37, 2272–2285 CrossRef.
- M. Li, V. Carreras, A. Jalba and T. Ollevier, Org. Lett., 2018, 20, 995–998 CrossRef CAS PubMed.
- D. Markad, S. Khullar and S. K. Mandal, Inorg. Chem., 2019, 58, 12547–12554 CrossRef CAS PubMed.
- H. Zhang, Y. Luo, C. Zhu, S. Dong, X. Liu and X. Feng, Org. Lett., 2020, 22, 5217–5222 CrossRef CAS PubMed.
- N. Fujieda, H. Ichihashi, M. Yuasa, Y. Nishikawa, G. Kurisu and S. Itoh, Angew. Chem. Int. Ed., 2020, 59, 7717–7720 CrossRef CAS PubMed.
- J. Luo, P. Chenzhang, W. Lan, J. Li, Z. Qin and B. Fu, ChemistrySelect, 2021, 6, 6890–6894 CrossRef CAS.
- J.-J. Huang, X.-Q. Zhang, J.-J. Yang, J.-F. Gong and M.-P. Song, Dalton Trans., 2022, 51, 8350–8367 RSC.
- N. Molleti, N. K. Rana and V. K. Singh, Org. Lett., 2012, 14, 4322–4325 CrossRef CAS PubMed.
- Y. Zhou, Q. Liu and Y. Gong, Org. Biomol. Chem., 2012, 10, 7618–7627 RSC.
- K. S. Yang, A. E. Nibbs, Y. E. Türkmen and V. H. Rawal, J. Am. Chem. Soc., 2013, 135, 16050–16053 CrossRef CAS PubMed.
- Z.-H. Wang, Z.-J. Wu, X.-Q. Huang, D.-F. Yue, Y. You, X.-Y. Xu, X.-M. Zhang and W.-C. Yuan, Chem. Commun., 2015, 51, 15835–15838 RSC.
- N. Molleti and V. K. Singh, Org. Biomol. Chem., 2015, 13, 5243–5254 RSC.
- Z.-H. Wang, Z.-J. Wu, D.-F. Yue, Y. You, X.-Y. Xu, X.-M. Zhang and W.-C. Yuan, Org. Biomol. Chem., 2016, 14, 6568–6576 RSC.
- G. Zhang, C. Zhu, D.-Y. Liu, J. Pan, J. Zhang, D. Hu and B. Song, Tetrahedron, 2017, 73, 129–136 CrossRef CAS.
- B. Cui, Y. Chen, J. Shan, L. Qin, C. Yuan, Y. Wang, W. Han, N. Wan and Y. Chen, Org. Biomol. Chem., 2017, 15, 8518–8522 RSC.
- V. Sharma, A. Kaur, S. C. Sahoo and S. S. Chimni, Org. Biomol. Chem., 2018, 16, 6470–6478 RSC.
- K. Sivamuthuraman and V. Kesavan, Org. Biomol. Chem., 2019, 17, 7166–7171 RSC.
- M.-X. Zhao, G.-Y. Zhu, X.-L. Zhao and M. Shi, Tetrahedron, 2019, 75, 1171–1179 CrossRef CAS.
- S. Rout, S. K. Ray, R. A. Unhale and V. K. Singh, Org. Lett., 2014, 16, 5568–5571 CrossRef CAS PubMed.
- R. Ballini, A. Palmieri and M. Petrini, Org. Chem. Front., 2022, 9, 6077–6113 RSC.
- D. Roca-Lopez, D. Sadaba, I. Delso, R. P. Herrera, T. Tejero and P. Merino, Tetrahedron, 2010, 21, 2561–2601 CrossRef CAS.
- X. Li, L. Guo, C. Peng and B. Han, Chem. Rec., 2019, 19, 394–423 CrossRef CAS PubMed.
- Z.-W. Zhang, S.-W. Liu, H.-P. Huang, Y.-H. Xie, R.-C. Huang, Y.-Q. Deng and N. Lin, RSC Adv., 2023, 13, 31047–31058 RSC.
- A. J. Simpson and H. W. Lam, Org. Lett., 2013, 15, 2586–2589 CrossRef CAS PubMed.
- M. Tavakolian, S. Vandati-Khajeh and S. Asgari, ChemCatChem, 2019, 11, 2943–2977 CrossRef CAS.
Footnotes |
† Electronic supplementary information (ESI) available: Experimental procedures, and spectral data of product. See DOI: https://doi.org/10.1039/d4ra03826f |
‡ These two authors contribute equally. |
|
This journal is © The Royal Society of Chemistry 2024 |
Click here to see how this site uses Cookies. View our privacy policy here.