DOI:
10.1039/D4RA03983A
(Paper)
RSC Adv., 2024,
14, 32101-32108
Highly sensitive colorimetric detection of Cd(II) based on silica sol modified with dithizone and cationic surfactant†
Received
30th May 2024
, Accepted 3rd October 2024
First published on 10th October 2024
Abstract
The cadmium ion (Cd2+) is highly poisonous and nondegradable and easily bioaccumulates through the food chain. Therefore, it is crucial to develop cost-effective chemical sensors for Cd2+ with fast response time, high selectivity, and very low detection limits. In this study, a colorimetric sensor for the determination of Cd2+ was fabricated by modifying silica sol with dodecyltrimethylammonium bromide (DTAB) and dithizone (DZ). Cd2+ formed a complex with DZ, changing the solution color immediately from purple to orange prior to detection using ultraviolet-visible spectrophotometry and a customized Cd analyzer for the precipitate. Under the optimum conditions, the developed Cd2+ sensor had a linear range of 0.01–0.25 mg L−1, a low limit of detection of 5.0 μg L−1, and outstanding repeatability. This sensor also showed good precision, with the relative standard deviations of less than 2.59% and 3.24% for the intra- and inter-day data, respectively. The proposed colorimetric method was successfully applied to determine Cd2+ in environmental water samples, and the results were comparable to those obtained using standard atomic absorption spectrometry. Moreover, quantitative analysis was conducted using the customized Cd analyzer to estimate the color intensity change, without requiring sophisticated scientific instruments. This colorimetric sensor can be used for the portable, cost-effective, and rapid on-site detection of Cd2+ in environmental water samples.
1. Introduction
The cadmium ion (Cd2+) is very toxic because it exhibits carcinogenicity and teratogenicity, severely impacting the kidneys, liver, and reproductive organs in humans.1,2 Moreover, it easily accumulates in the human body through the food chain owing to its nondegradable nature.3,4 Common sources of Cd2+ in the environment are paint factory effluents, plastics, hardware fittings, nuclear reactor control rods, radium detection devices, photoconductive elements in televisions, and electronic waste (e.g., mobile phones and their batteries as well as computer circuit boards).5 The World Health Organization established the maximum residue limit of Cd2+ in drinking water to be 0.003–0.005 mg L−1.6 Therefore, it is crucial to develop highly selective, rapid, and cost-effective chemical sensors with very low detection limits for Cd2+ in environmental water.
Reported high-performance techniques for Cd determination are based on inductively coupled plasma-mass spectrometry,7–9 inductively coupled plasma-atomic emission spectrometry,10 inductively coupled plasma-optical emission spectrometry,11 atomic absorption spectroscopy,12–15 and electrochemistry.16–18 However, these techniques require highly trained operators, the analysis process is time-consuming, and the instrument is typically expensive and nonportable. In recent years, colorimetric methods have received great attention due to being simple, inexpensive, and effective for quantitative analyses. They also allow detection by the naked eye without specialized detectors. Colorimetric methods for detecting heavy metals rely on the different chromogenic materials. The main content of this work focused on the aggregation (or the reverse dissociation process) of noble metals and nanoparticles such as gold nanoparticles, silver nanoparticles, and silica sol.19–24
Silica sol (also called colloidal silica) has desirable properties for colorimetric sensors. As a nanocolloid having a siloxane network structure (Si–O–Si),25 it is easily synthesized using the Stöber method that involves the hydrolysis and condensation of tetraethoxysilane (TEOS) and L-arginine (L-arg).25–27 This method is popular because the sol–gel process is inexpensive, easy, and highly efficient compared with other methods. Particles in the obtained silica sol are small and well dispersed, resulting in a large specific surface area. Notably, silica sol can be directly used as a sorbent without the energy-intensive and/or lengthy drying or calcination steps. We have previously used silica sol as a new sorbent for detecting trace levels of Fe2+ (ref. 27) and Cd2+ which use the trimethyl tetradecyl ammonium bromide (TTAB) and murexide on silica sol as a colorimetric probe.24
Cationic surfactants can facilitate the aggregation of silica sol and enhance its surface properties through electrostatic interactions between the positively charged surfactant and negatively charged silica sol particles.24,27–29 In addition, the sensitivity and selectivity of nanoparticle-based colorimetric sensors can be improved by modifying the particle surface using specific ligands. The adsorption of metal cations on silica sol occurs via electrostatic interactions between the hydroxyl groups of silanol and metal cations. However, the ligands should be carefully chosen to enhance metal adsorption.
In colorimetric detection, the derivatization of Cd2+ with an appropriate ligand produces complexes that display different colors. It also enhances sensitivity and selectivity for Cd2+ detection. The following ligands have been reported to form complexes with Cd2+: 5,7-dibromo-8-hydroxyquinoline,30 ammonium pyrrolidinedithiocarbamate,31, (E)-N′-(2-hydroxy-5-nitrobenzylidene)isonicotinoylhydrazone, 2-(4-fluoro (benzlideneamino)benzenothiol),32 1-(2-pyridylazo)-2-naphthol,33 and murexide.24 To the best of our knowledge, there have been some reports on using dithizone (DZ) as a ligand for Cd2+ detection, because the sulfur and nitrogen atoms in DZ can react with metal ions by donating free electron pairs.34,35 Notably, DZ binds strongly with Cd2+ with a high complex formation constant (log
Kf) under suitable conditions.36,37
In this study, we developed an effective, simple, and portable colorimetric sensor for the detection of Cd2+. A probe was prepared by modifying silica sol with DZ and dodecyltrimethylammonium bromide (DTAB, a cationic surfactant). This DTAB/DZ/silica sol probe is sensitive and selective to Cd2+. Specifically, the presence of Cd2+ caused a color change of the aqueous probe solution from purple to orange, and this color change was measured by spectrophotometry at 435 nm for quantification and a customized Cd analyzer. The proposed colorimetric probe was successfully applied to the determination of Cd2+ in environmental water samples.
2. Experimental section
2.1 Materials and chemicals
All chemicals were of analytical reagent grade except for TEOS (gas chromatography grade, Sigma-Aldrich). Cadmium chloride (CdCl2) was obtained from HIMEDIA. Cetyltrimethylammonium bromide (CTAB) and Dithizone (DZ) were purchased from Fluka. Cobalt(II) chloride hexahydrate (CoCl2·6H2O), copper(II) sulfate pentahydrate (CuSO4·5H2O), ammonium ferrous sulfate hexahydrate (Fe(NH4)2(SO4)2·6H2O), and potassium chloride (KCl) were obtained from CARLO ERBA. L-Arginine (C6H14N4O2) was obtained from ACROS. Sodium dihydrogen phosphate dihydrate (NaH2PO4·2H2O) and trimethyl tetradecyl ammonium bromide (TTAB) were obtained from KEMAUS.
Sodium acetate and acetic acid were purchased from Merck. Dodecyltrimethylammonium bromide (DTAB), nitric acid (HNO3, 65%), and methanol (MeOH) were obtained from Sigma-Aldrich. Magnesium sulfate (MgSO4), ortho-phosphoric acid (H3PO4), and sulfuric acid (H2SO4) were acquired from QRëC. Deionized water produced from ELGA (PURELAB Ultra Analytic) with a specific resistivity of 18.2 MΩ cm was used in all experiments.
A Cd2+ stock solution (1.0 mmol L−1) was prepared by dissolving an appropriate amount of Cd2+ in 0.1 mol L−1 H2SO4. Every day, Cd2+ working solutions were prepared by the stepwise dilution of this stock solution with deionized water. Phosphate buffers at various pH values (pH 2.0–3.0) were prepared by mixing 0.1 mol L−1 H3PO4 and 0.1 mol L−1 NaH2PO4 in different ratios. The acetate buffer solution at various pH values (pH 4.0–6.0) were prepared by 0.1 mol L−1 acetic acid and sodium acetate in different ratios. All glassware was stored in 10% (v/v) HNO3 for at least 24 h and washed thoroughly with deionized water before use.
2.2 Instrumentation
Absorption spectra were recorded on an ultraviolet-visible (UV-vis) spectrophotometer (Cary 60 Agilent, Australia) by scanning the reaction solution in a 3.5 mL quartz cuvette from 380 to 490 nm. Fourier-transform infrared spectroscopy was performed using a Bruker Tensor 27 spectrometer. The zeta potential was measured using a Zetasizer Nano ZS (Malvern, UK) to confirm the charge on the silica sol surface. The morphology of silica sol was studied using focused ion beam-scanning electron microscopy (FIB-SEM; Helios NanoLab G3 CX, FEI, USA). A customized Cd analyzer based on RGB analysis was used to measure the change in red (R) color intensity.
2.3 Synthesis of silica sol
Silica sol was prepared using the Stöber method according to our previous reports, based on the hydrolysis and condensation of TEOS and L-arg.24,27 L-arg (0.09 g) was dissolved in deionized water (250 mL) and stirred at 60 °C for 30 min in an oil bath. Then, TEOS (18.66 g) was added. The mixture was stirred continuously at 60 °C and 300 rpm for 26 h using a magnetic stirrer, and then kept at room temperature for subsequent experiments.
2.4 Colorimetric sensor for Cd2+ detection
The mechanism of the colorimetric DTAB/DZ/silica sol probe is illustrated in Fig. 1. To prepare this probe, silica sol (2000 μL), DZ (0.09 mg L−1), and DTAB (1000 μL) were added to a 15 mL centrifuge tube. Next, a standard solution containing 0.09 mg L−1 Cd2+ was added to the centrifuge tube, and it was then diluted with a 0.1 mol L−1 phosphate buffer solution (pH 3.0, up to 10 mL) through manual shaking. The obtained colloidal solution (DTAB/[Cd2+-DZ]/silica sol) was self-assembled and precipitated to the bottom of the centrifuge tube under gravity. Finally, the absorbance of the upper phase was measured at 435 nm. The efficiency in the adsorption or extraction of the formed [Cd2+-DZ] complex was evaluated using %adsorption defined in eqn (1):38–40 |
%Adsorption = [(A0 − At)/A0] × 100
| (1) |
where A0 and At are absorbances of the [Cd2+-DZ] complex before and after adsorption on the colorimetric probe, respectively. The precipitate was filtered through a membrane filter and evaluated using a customized Cd analyzer.
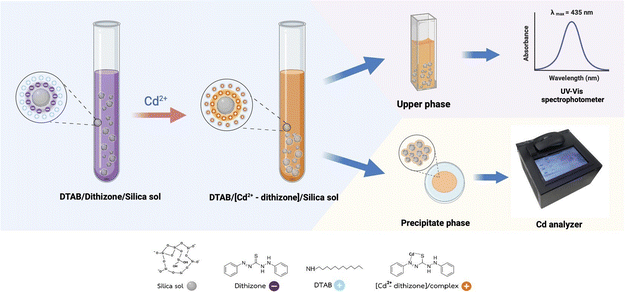 |
| Fig. 1 Proposed colorimetric sensor for Cd2+ determination based on dodecyltrimethylammonium bromide (DTAB)/dithizone (DZ)/silica sol. | |
In this work, Cd quantification was performed by the expression of calibration curves using a customized Cd analyzer to evaluate the channels red (R), green (G) and blue (B). The R channel displays a well-linear response with an acceptable correlation coefficient (R2) when compared with others. Because the color of cadmium complex was related to the R color. Thus, the R value was used as an analytical signal for the experiments.
2.5 Sample analysis
The prepared colorimetric sensor was used to detect Cd2+ in four environmental water samples (W1–W4) including tap water (W1), natural water (W2), and wastewater (W3–4). All samples were collected from Khon Kaen Province, Thailand and first passed through a 0.45 μm membrane filter (VertiClean™ NYLON) prior to Cd2+ detection.
3. Results and discussion
3.1 Characterization of DTAB/DZ/silica sol probe
The morphologies of silica sol, DTAB/silica sol, and DTAB/[Cd2+-DZ]/silica sol were observed through FIB-SEM. According to the surface morphology in Fig. 2A, the silica sol particles were spherical and had a diameter of 100 ± 2.35 nm (n = 20). After adding DTAB, DTAB/silica sol (particle size: 50 ± 4.25 μm, n = 20) formed large rod-shaped particles owing to the aggregation of DTAB and silica sol (Fig. 2B). The presence of the [Cd2+-DZ] complex promoted the aggregation of DTAB/[Cd2+-DZ]/silica sol, as shown in Fig. 2C.
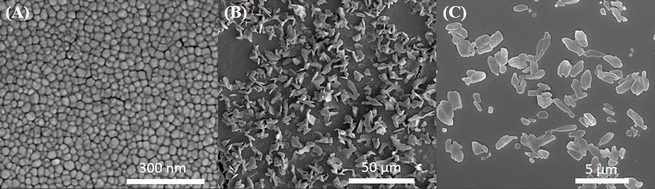 |
| Fig. 2 SEM images of (A) silica sol, (B) DTAB/silica sol, and (C) DTAB/[Cd2+-DZ]/silica sol. | |
To confirm the adsorption of [Cd2+-DZ] onto DTAB/silica sol, the surface charge of the probe particles was studied using a zeta potential analyzer. Pure silica sol had a negative potential (−31.23 mV), after the addition of DTAB which has a positive charge, the potential was slightly raised to positive value (−0.11 mV). When silica sol was combined with [Cd2+-DZ], a positive potential (2.13 mV) was observed instead. Furthermore, DTAB/[Cd2+-DZ]/silica sol had a more positive potential (5.07 mV). These changes suggest that the [Cd2+-DZ] complex was adsorbed on DTAB/silica sol.
3.2 Optimization of colorimetric probe for Cd2+ detection
3.2.1 Effects of pH and time. The pH of the aqueous solution plays a key role in the speciation of chelating agents, complex formation, and extraction efficiency. Here, complex formation between DZ and Cd2+ was investigated in the pH range of 2.0–6.0 using different buffer solutions (0.1 mol L−1 phosphate buffer and acetate buffer). As shown in Fig. S1A,† the [Cd2+-DZ] complex solution achieved maximum absorbance at pH 3.0 and a slightly lower absorbance at higher pH. Therefore, the phosphate buffer at pH 3.0 was selected for further experiments.To study stability of the [Cd2+-DZ] complex, its absorbance was monitored for 50 min. The absorbance increased during the first 30 min and then decreased slightly (Fig. S1B†). Thus, in the subsequent experiments, the detection of Cd2+ was carried out at 30 min after mixing.
3.2.2 Effect of probe volume. The effect of the amount of sorbent on extraction efficiency was studied by varying the volume of silica sol from 1.0 to 6.0 mL and measuring %adsorption and red (R) intensity. The %adsorption (obtained via UV-vis spectrophotometry) increased significantly with the increase in the silica sol volume from 1.0 to 3.0 mL and decreased when the volume was above 3 mL, as shown in Fig. 3A. The R intensity (measured for the precipitate on the membrane filter) was evaluated using a customized Cd analyzer based on the color change. The results showed a trend similar to that of %adsorption. Thus, the of silica sol volume was fixed at 3.0 mL in the subsequent experiments.
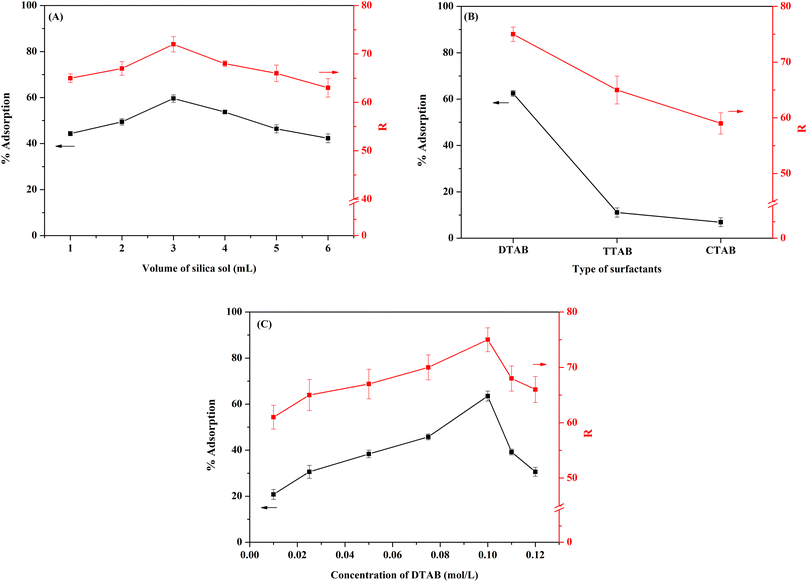 |
| Fig. 3 Effects of (A) volume of silica sol, (B) type of surfactant, and (C) concentration of DTAB on the colorimetric sensor (DTAB/DZ/silica sol) for Cd2+ determination. | |
3.2.3 Effect of surfactant. Surfactants are stabilizing agents that induce the aggregation of [Cd2+-DZ] complexes and nanoparticles (such as silica sol).24,27 Three cationic surfactants, namely, CTAB, DTAB, and TTAB, were tested. The addition of a cationic surfactant made the solution cloudy, because the surfactant aggregated with [Cd2+-DZ] and silica sol to produce surfactant/[Cd2+-DZ]/silica sol. Fig. 3B shows the %adsorption of [Cd2+-DZ] on silica sol modified with different cationic surfactants. It was obvious that DTAB caused the highest %adsorption. The color intensity of the precipitate on the membrane filter was evaluated using a customized Cd analyzer, and the results showed a trend similar to that of %adsorption. Therefore, DTAB was selected as the surfactant in the subsequent experiments.Next, the concentration of DTAB was studied in the 0.010–0.120 mol L−1 range, which is at or above the critical micellar concentration of DTAB (0.01401 mol L−1).41 The results (Fig. 3C) show that the %adsorption of the [Cd2+-DZ] complex increased significantly upon increasing the DTAB concentration from 0.01 to 0.1 mol L−1, similar to the trend of R intensity measured for the precipitate. The highest %adsorption and R intensity were obtained at 0.1 mol L−1 DTAB. Above this concentration, both values decreased significantly because of the dilution effect. Thus, 0.1 mol L−1 DTAB was selected for further study.
3.3 Selectivity toward Cd2+
Selectivity is a key parameter of colorimetric sensors because real environmental samples tend to contain interfering species such as other cations and anions. Therefore, we tested the probe's selectivity toward other common ions found in water samples (Co2+, Mg2+, Fe2+, Cu2+, K+, Cl−, NO3−, and SO42−). Two sets of experiments were carried out. (i) The DTAB/DZ/silica sol probe was mixed with a single ionic species (Cd2+ or coexisting ions) at 0.09 mg L−1. The corresponding absorbance intensities are shown in Fig. 4A. Only Cd2+ produced an orange complex with strong absorption, indicating that this ion alone substantially interacted with the DTAB/DZ/silica sol probe. (ii) A solution containing Cd2+ and a coexisting ion (both at 0.09 mg L−1) was tested. As shown in Fig. 4B, all other ions had a negligible effect on absorbance. In summary, the proposed colorimetric sensor exhibited excellent Cd2+ selectivity.
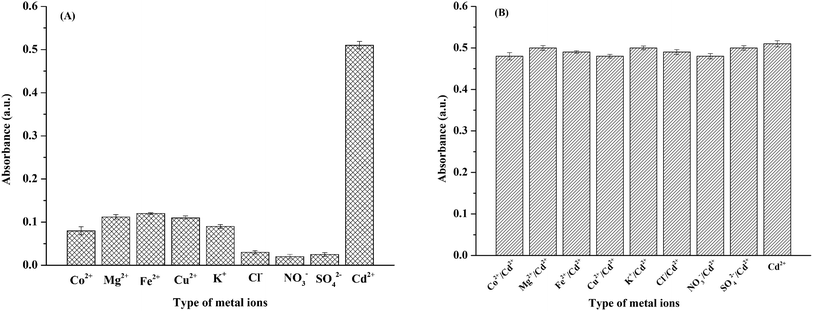 |
| Fig. 4 Absorbance of the colorimetric probe in the presence of (A) one ionic species at 0.09 mg L−1 and (B) Cd2+ (0.09 mg L−1) and a coexisting ion (0.09 mg L−1). | |
3.4 Analytical performance
The analytical performance of the proposed DTAB/DZ/silica sol colorimetric probe was investigated for Cd2+ solutions at various concentrations under the optimized experimental conditions through UV-vis spectroscopy and by using the customized Cd analyzer. The obtained absorption spectra are shown in Fig. 5A. The absorbance at 435 nm decreased as the Cd2+ concentration increased from 0.01 to 0.25 mg L−1. The linear range of %adsorption was 0.01–0.25 mg L−1 Cd2+ with a regression equation of Y = 116.70 X + 21.30, and the determination coefficient (R2) was 0.9944 (Fig. 5B).
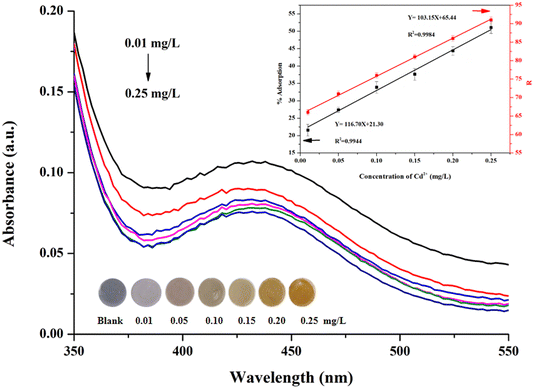 |
| Fig. 5 Quantitative Cd2+ detection using the colorimetric sensor. (A) Absorption spectra of DTAB/[Cd2+-DZ]/silica sol at various Cd2+ concentrations (inset: photographic images). Peak wavelength: 435 nm. (B) Calibration plots for %adsorption (left y-axis) and R intensity (right y-axis) versus Cd2+ concentration. | |
Moreover, the relationship between the R intensity and Cd2+ concentration was evaluated by taking a digital photograph and measuring the color intensity using the customized Cd analyzer (Fig. 5B). The regression equation was Y = 103.15X + 65.44, with R2 = 0.9984.
The limit of detection (LOD) and limit of quantitation (LOQ) were calculated on the basis of the ratio of the standard deviation of the blank solution (s) to the slope of the calibration plot (m) at 3 and 10, and the obtained values were 5.0 and 18 μg L−1, respectively. The precision of the DTAB/DZ/silica sol probe was expressed as the relative standard deviation in percentage (%RSD, n = 5) when measuring 0.09 mg L−1 Cd2+ in one (intra-day) and five consecutive days (inter-day). The results indicate that the proposed probe has good precision with %RSD values less than 7.67%. A comparison of the proposed sensor with other colorimetry-based sensors for the detection of Cd2+ is presented in Table 1. The LODs of the proposed sensor are comparable to those in the literature, thus serving as a potential alternative medium for Cd2+ detection.
Table 1 Different colorimetric sensors for Cd2+ detection
Method |
Colorimetric probe |
LOD |
Sample |
Reference |
Colorimetric |
Chitosan-dithiocarbamate-modified gold nanoparticles |
7.06 μg L−1 (0.063 μM) |
Tap water |
42 |
Colorimetric |
1-Amino-2-naphthol-4-sulfonic-acid-functionalized silver nanoparticles |
0.087 μM |
Milk powder, serum, and lake water |
43 |
Colorimetric |
Silver nanoparticles capped with chalcone carboxylic acid |
0.13 μM |
Drinking water and lake water |
44 |
Colorimetric |
TTAB/murexide/silica sol |
0.21 μM |
Rice |
24 |
Colorimetric |
DTAB/DZ/silica sol |
5 μg L−1 (44.5 nM) |
Environmental water |
This work |
3.5 Determination of Cd2+ in real water samples
The proposed colorimetric sensor (DTAB/DZ/silica sol) was used to detect Cd2+ contamination in environmental water samples. However, Cd2+ was not detected in any of the four as-collected samples. To evaluate recovery, Cd2+ was spiked at three concentrations (0.050, 0.125, and 0.250 mg L−1) into the samples before performing additional determination experiments. The recoveries are summarized in Table 2. High recoveries were obtained in the acceptable range of 96.8–105.6% with an RSD below 4.0%. These results indicated that the proposed colorimetric sensor suffered no matrix effect. A t-test was conducted to compare the accuracy of the proposed colorimetric and standard flame atomic absorption spectroscopy (FAAS) methods, considering a p-value of 0.05 (at the 95% confidence limit). The calculated t value (t-cal = 1.35) did not exceed the tabulated t value (t-table = 2.92), indicating that there was no significant difference between the two methods.
Table 2 Performance of the colorimetric and FAAS methods for the determination of Cd2+ in spiked environmental water samples including tap water (W1), natural water (W2), and wastewater (W3–4)
Samples |
Proposed colorimetric sensor |
FAAS method |
Spiked (mM) |
Foundb (mM) |
Recoveryc (%) |
%RSDd |
Foundb (mM) |
Recoveryc (%) |
%RSDd |
nd: not detected. Detected amount. Recovery = [(Cdetected − Csample)/Cspiked] × 100. n = 3. |
W1 |
— |
nd a |
— |
— |
nd |
— |
— |
0.050 |
0.051 |
101.7 |
1.6 |
0.049 |
98.0 |
3.8 |
0.125 |
0.128 |
102.8 |
1.2 |
0.123 |
98.4 |
2.1 |
0.250 |
0.259 |
103.5 |
1.5 |
0.245 |
98.0 |
1.9 |
W2 |
— |
nd |
— |
— |
nd |
— |
— |
0.050 |
0.048 |
96.8 |
1.3 |
0.045 |
90.0 |
4.2 |
0.125 |
0.130 |
104.1 |
2.6 |
0.122 |
97.6 |
1.6 |
0.250 |
0.251 |
100.3 |
2.4 |
0.252 |
100.8 |
2.3 |
W3 |
— |
nd |
— |
— |
nd |
— |
— |
0.050 |
0.048 |
96.8 |
3.0 |
0.047 |
94.0 |
3.5 |
0.125 |
0.132 |
105.6 |
2.3 |
0.121 |
96.8 |
1.7 |
0.250 |
0.259 |
103.7 |
1.8 |
0.243 |
97.2 |
2.4 |
W4 |
— |
nd |
— |
— |
nd |
— |
— |
0.050 |
0.049 |
98.0 |
2.1 |
0.044 |
88.0 |
3.1 |
0.125 |
0.124 |
99.2 |
3.9 |
0.123 |
98.4 |
2.3 |
0.250 |
0.252 |
100.8 |
2.5 |
0.249 |
99.6 |
2.4 |
4. Conclusion
A selective and sensitive colorimetric sensor was developed for detecting Cd2+ via complexation with DZ. The colorimetric probe was fabricated by applying self-assembled DTAB and DZ on a silica sol surface. In the presence of Cd2+, the probe immediately changed color from purple to orange owing to the complexation of Cd2+ with DZ, and an orange precipitate of DTAB/[Cd2+-DZ]/silica sol was formed simultaneously. Silica sol enhanced detection sensitivity via adsorption. The quantitative determination of Cd2+ was performed through UV-vis spectrophotometry and a customized Cd analyzer. The proposed method was sensitive, with an LOD of 5.0 μg L−1 that equals the permissible level of Cd in drinking water. When the colorimetric sensor was applied to environmental water samples, the results were comparable to those of the standard FAAS method, providing highly satisfactory recoveries. Therefore, this colorimetric sensor has great potential for the portable, cost-effective, and rapid on-site detection of Cd2+.
Data availability
The data supporting this article have been included as part of the ESI.†
Author contributions
Arpaporn Litluechai: conceptualization, methodology, writing – original draft. Arreerat Prompa: methodology, validation. Pikaned Uppachai: methodology, validation. Wirat Jarernboon: methodology, validation. Nutthaya Butwong: methodology, validation. Siriboon Mukdasai: conceptualization, supervision, writing – review & editing.
Conflicts of interest
There are no conflicts to declare.
Acknowledgements
Financial supports from Materials Chemistry Research Center (MCRC) and the Center of Excellence for Innovation in Chemistry (PERCH-CIC), Ministry of Higher Education, Science, Research and Innovative, Thailand are gratefully acknowledged. S. Mukdasai also would like to acknowledge the Fundamental Fund of Khon Kaen University which has received funding support from the National Science Research and Innovation Fund (NSRF).
References
- J. Y. Cabon, Spectrochim. Acta Part B At. Spectrosc., 2002, 57(3), 513–524 CrossRef.
- M. Xu, P. Hadi, G. Chen and G. McKay, J. Hazard. Mater., 2014, 273, 118–123 CrossRef CAS PubMed.
- G. Lee, Z. Suonan, S. H. Kim, D. W. Hwang and K. S. Lee, Mar. Pollut. Bull., 2019, 149, 110509 CrossRef CAS PubMed.
- M. P. Waalkes, J. Inorg. Biochem., 2000, 79(1–4), 241–244 CrossRef CAS PubMed.
- L. Wu, X. Fu, H. Liu, J. Li and Y. Song, Anal. Chim. Acta, 2014, 851, 43–48 CrossRef CAS PubMed.
- World Health Organization, Guidelines for drinking-water quality: fourth edition incorporating first addendum, 2017, https://iris.who.int/handle/10665/254637 Search PubMed.
- N. Zhang, K. Shen, X. Yang, Z. Li, T. Zhou, Y. Zhang, Q. Sheng and J. Zheng, Food Chem., 2018, 264, 462–470 CrossRef CAS PubMed.
- G. M. Lo Dico, F. Galvano, G. Dugo, C. D’ascenzi, A. Macaluso, A. Vella, G. Giangrosso, G. Cammilleri and V. Ferrantelli, Food Chem., 2018, 245, 1163–1168 CrossRef CAS PubMed.
- R. S. Lamarca, S. D. S. Ferreira, É. R. Paganini, N. D. S. Ferreira, S. C. Ayala-Durán and G. Isquibola, et al., J. Mol. Liq., 2024, 398, 124264 CrossRef CAS.
- M. Zougagh, Talanta, 2002, 56(4), 753–761 CrossRef CAS PubMed.
- Z. Cai and Z. Wang, Anal. Chim. Acta, 2022, 1203, 339724 CrossRef CAS PubMed.
- N. Pourreza and K. Ghanemi, J. Hazard. Mater., 2010, 178(1–3), 566–571 CrossRef CAS PubMed.
- S. Gunduz, S. Akman and M. Kahraman, J. Hazard. Mater., 2011, 186(1), 212–217 CrossRef CAS PubMed.
- A. Sixto, A. Mollo and M. Knochen, J. Food Compos. Anal., 2019, 82, 103229 CrossRef CAS.
- M. Soylak, A. N. Çoban and H. E. H. Ahmed, Food Chem., 2024, 442, 138426 CrossRef CAS PubMed.
- Z. Wang, H. Wang, Z. Zhang, X. Yang and G. Liu, Electrochim. Acta, 2014, 120, 140–146 CrossRef CAS.
- G. Chen, X. Hao, B. L. Li, H. Q. Luo and N. B. Li, Sens. Actuators, B, 2016, 237, 570–574 CrossRef CAS.
- X. Wang, W. Lin, C. Chen, L. Kong, Z. Huang, D. Kirsanov, A. Legin, H. Wan and P. Wang, Sens. Actuators, B, 2022, 366, 131922 CrossRef CAS.
- Y. M. Sung and S. P. Wu, Sens. Actuators, B, 2014, 201, 86–91 CrossRef CAS.
- Y. Gan, T. Liang, Q. Hu, L. Zhong, X. Wang, H. Wan and P. Wang, Talanta, 2020, 208, 120231 CrossRef CAS PubMed.
- G. Li, Q. Yang, X. Liu, L. Liu, J. Han and X. Li, J. Photochem. Photobiol. Chem., 2024, 447, 115228 CrossRef CAS.
- M. Maruthupandi, M. R. J. Stairish, S. Sahila and N. Vasimalai, J. Mol. Struct., 2022, 1269, 133798 CrossRef CAS.
- S. Ebrahim Mohammadzadeh, F. Faghiri and F. Ghorbani, Microchem. J., 2022, 179, 107475 CrossRef CAS.
- J. Charoensuk, J. Thonglao, B. Wichaiyo, K. Mukdasai, Y. Santaladchaiyakit, S. Srijaranai and S. Mukdasai, Microchem. J., 2021, 160, 105666 CrossRef CAS.
- P. A. Bazuła, P. M. Arnal, C. Galeano, B. Zibrowius, W. Schmidt and F. Schüth, Microporous Mesoporous Mater., 2014, 200, 317–325 CrossRef.
- Y. Takeda, Y. Komori and H. Yoshitake, Colloids Surf. A Physicochem. Eng. Asp., 2013, 422, 68–74 CrossRef CAS.
- S. Metarwiwinit, S. Mukdasai, C. Poonsawat and S. Srijaranai, New J. Chem., 2018, 42(5), 3401–3408 RSC.
- F. Accioni, D. García-Gómez, E. Girela and S. Rubio, Talanta, 2018, 182, 574–582 CrossRef CAS PubMed.
- A. Galal, N. F. Atta and E. H. El-Ads, Talanta, 2012, 93, 264–273 CrossRef CAS PubMed.
- M. J. Ahmed and M. T. I. Chowdhury, Anal. Sci., 2004, 20(6), 987–990 CrossRef CAS PubMed.
- N. Goudarzi, J. Agric. Food Chem., 2009, 57(3), 1099–1104 CrossRef CAS PubMed.
- B. N. Kumar, S. H. Kumar, G. G. Redhi and N. V. V. Jyothi, Asian J. Chem., 2016, 28(8), 1861–1866 CrossRef CAS.
- A. Thongsaw, W. C. Chaiyasith, R. Sananmuang, G. M. Ross and R. J. Ampiah-Bonney, Food Chem., 2017, 219, 453–458 CrossRef CAS PubMed.
- R. Sedghi, S. Kazemi and B. Heidari, Sens. Actuators, B, 2017, 245, 860–867 CrossRef CAS.
- N. Thiagarajan and M. Subbaiyan, Anal. Chim. Acta, 1992, 269(2), 269–272 CrossRef CAS.
- H. Fiedler, Talanta, 2004, 64(1), 190–195 CrossRef CAS PubMed.
- B. E. Saltzman, Anal. Chem., 1953, 25(3), 493–496 CrossRef CAS.
- Md. R. Awual, Md. M. Hasan and H. Znad, Chem. Eng. J., 2015, 259, 611–619 CrossRef CAS.
- Md. R. Awual, T. Yaita, H. Shiwaku and S. Suzuki, Chem. Eng. J., 2015, 276, 1–10 CrossRef CAS.
- Md. R. Awual, T. Yaita, S. Suzuki and H. Shiwaku, J. Hazard. Mater., 2015, 291, 111–119 CrossRef CAS PubMed.
- K. M. Sachin, S. A. Karpe, M. Singh and A. Bhattarai, R. Soc. Open Sci., 2019, 6(3), 181979 CrossRef CAS PubMed.
- V. N. Mehta, H. Basu, R. K. Singhal and S. K. Kailasa, Sens. Actuators, B, 2015, 220, 850–858 CrossRef CAS.
- P. Huang, B. Liu, W. Jin, F. Wu and Y. Wan, J. Nanoparticle Res., 2016, 18(11), 327 CrossRef.
- Y. Dong, L. Ding, X. Jin and N. Zhu, Microchim. Acta, 2017, 184(9), 3357–3362 CrossRef CAS.
|
This journal is © The Royal Society of Chemistry 2024 |
Click here to see how this site uses Cookies. View our privacy policy here.