DOI:
10.1039/D4RA04670F
(Paper)
RSC Adv., 2024,
14, 27972-27979
A novel extraction method for three ochratoxins in human urine based on polystyrene/polyethersulfone electrospun nanofibers coated with copper nanoparticles†
Received
27th June 2024
, Accepted 16th August 2024
First published on 2nd September 2024
Abstract
In this study, four types of nanofibers were prepared via electrospinning and characterized through SEM, TEM, EDS, FTIR, TG, XPS and water contact angle analyses, and the novel polystyrene/polyethersulfone nanofibers coated with copper nanoparticles (PS/PES-CuNPs nanofibers) were developed as an ideal adsorbent for the extraction of three ochratoxins from human urine. The solid-phase extractant of sample pretreatment displayed preferable sensitivity and an extraction effect, and the analytical method based on the novel packed-fiber solid-phase extraction strategy followed by high performance liquid chromatography-fluorescence detection (PFSPE-HPLC-FLD) achieved an exceedingly low limit of detection (LOD) and limit of quantification (LOQ) of 0.108–0.162 μg L−1 and 0.658–0.701 μg L−1, respectively; a high spiked recovery of 71.3–92.0% and a lower adsorption time of 7 min, thus demonstrating excellent results compared with other reported adsorbents for ochratoxins from various samples. With the application of this method for the detection of ochratoxins in human urine samples, six in thirty samples were tested positive. This study confirmed that the PS/PES-CuNP nanofibers and PFSPE showed promising potential as a sensitive method for simultaneous extraction and detection of ochratoxins in complex samples.
1 Introduction
As a highly toxic substance of the mycotoxin family, ochratoxins (OTs, including OTA, OTB and OTC) have been widely monitored since they were first reported in Nature in 1965.1 Particularly, OTA was classified as a possible human carcinogen (Group 2B) in 1993 by the International Agency for Research on Cancer because of its strong nephrotoxicity, hepatotoxicity, immunotoxicity, teratogenicity, carcinogenicity, genotoxicity, and neurotoxicity.2–4 Frequent exposure to OTs can lead to a range of health issues in animals and humans. Research has confirmed that ochratoxins have a causal relationship with Balkan endemic nephropathy (BEN).5,6 Notably, ochratoxins are directly excreted in urine instead of being removed through glomerular filtration.7 Therefore, the task of establishing a precise and effective analytical method for ochratoxin detection in human urine is imminent.
Owing to the complicated matrix of urine and the trace concentration of OTs, direct detection would affect the sensitivity and accuracy of analytical methods. Therefore, it is necessary to pretreat the samples before instrumental analysis. The purpose is to separate and enrich analytes and reduce matrix effects and interference in sample solution.8 Currently, QuEChERS,9 liquid–liquid extraction,10,11 and immunoaffinity columns12,13 have been reported to extract ochratoxins. However, selecting an appropriate pretreatment method is crucial for the enrichment of ochratoxins. Solid-phase extraction (SPE) is the best choice for sample pretreatment for its advantages of high selectivity, low cost, and simple preparation.14 Generally, some nanomaterials with high structural stability, such as metal organic frameworks, molecular imprinting materials or carbon nanotubes, are utilized as SPE adsorbents. However, secondary pollution may arise in the extraction process because of their weak separation ability.15,16 Therefore, the development of new adsorption materials for SPE has been one of the most challenging subject in analytical science.17
Polymer nanofibers stand out among other adsorbents as they exhibit superior characteristics, including exceptional mechanical and thermal stability.18 Research has demonstrated that nanofibers are beneficial as adsorbents, since they possess a diameter size of submicrometers or even nanometers, resulting in superior specific surface area and porosity and exhibiting satisfactory adsorption capacity.19,20 Furthermore, during the extraction process, nanofibers do not leak or accumulate in the SPE column and can be easily packed into the column.21 Additionally, electrospinning is emerging as a preferable method for synthesizing nanofibers due to its simplicity of operation, convenience, and controllable process parameters. In addition, the functionalized polymer nanofibers can be promoted by chemical modification, developing optimal adsorbents with great selectivity for the targets.22 It is reported that the detection of OTs by Au and Ag nanoclusters has been widely utilized, but copper nanoclusters have rarely been investigated.23,24 Considering the adjacent positions and similar effects of copper, gold, and silver, it is also feasible that copper nanoparticles were introduced in electrospun nanofibers for the extraction of OTs.25 Combining electrospun nanofibers with copper nanoparticles can effectively improve the adsorption performance of nanofibers, since more adsorption sites are introduced when the specific surface area of the adsorbent is enhanced.26,27 Therefore, the proposed modification methods are expected to have satisfactory adsorption efficiency.
The emerging packed-fiber solid-phase extraction (PFSPE) method is a solid-phase extraction technology that integrates extraction, enrichment, purification, and separation, greatly simplifying the steps of sample pretreatment.28 In this study, new polystyrene/polyethersulfone nanofibers coated with copper nanoparticles were prepared as a selective adsorbent for extracting ochratoxins from human urine samples. An analytical method based on the novel PFSPE followed by high performance liquid chromatography-fluorescence detection (PFSPE-HPLC-FLD) was established for simultaneous determination of three ochratoxins in human urine.
2 Materials and methods
2.1 Materials and reagents
Ochratoxin A (OTA, C20H18ClNO6, purity 98%, Mw = 403.81), Ochratoxin B (OTB, C20H19NO6, purity 98%, Mw = 369.37), Ochratoxin C (OTC, C22H22ClNO6, purity 98%, Mw = 431.87), analytically pure sodium borohydride, copper acetate, and sodium chloride were purchased from Shanghai Aladdin Company. Polystyrene (PS, [CH2CH(C6H5)]n, Mw = 18
500 Da) and polyethersulfone (C24H18Cl2O6S2, purity ≥ 99%) were obtained from the Department of Applied Chemistry, Nanjing University of Technology. Polyacrylonitrile (PAN, (C3H3N)n, Mw = 150
000) and analytically pure glacial acetic acid were purchased from Shanghai McLean Company. Polyvinylpyrrolidone (PVP) K30, N,N-dimethylformamide (DMF) and hydrochloric acid (analytically pure) were purchased from Shanghai National Pharmaceutical Group. Chromatographically pure methanol and acetonitrile were purchased from Tedia Corporation in the United States.
The preparation of artificial urine was based on previous research29 and slightly modified. The detailed preparation plan can be found in Part 1 in ESI.†
2.2 Instruments and reagents
The following instruments were utilized: Fourier transform infrared spectrometer (FTIR 5700, Thero Electron Co., Waltham, USA), scanning electron microscope (SEM 8100, Tokyo, Japan), transmission electron microscope (TEM FEI Talos-S, Oregon, America), energy dispersive spectrometer (EDS, Oregon, America), Waters e4695 separation unit and Waters 2475 fluorescence detector (Waters Co., Milford, America), 4.6 × 150 mm 5 μm InertSustain C18 column (GL Sciences, Tokyo, Japan), syringe pump (Hawk Medical Instrument Co., Ltd, Shenzhen, China), and high voltage direct current power (Dongwen High Voltage Power Supply Co., Ltd, Tianjin, China).
2.3 Preparation of nanofibers
The nanofibers were prepared by electrospinning. Firstly, 0.5 g each of PS, PES, and copper acetate were dissolved in 8.5 mL of DMF. A uniform polymer solution for electrospinning was obtained by stirring the blend continuously for 10 h at room temperature. After, the solution was transferred into a 10 mL syringe with a 23 gauge stainless steel needle. The electrospinning parameters were set as follows: a receiving distance of 20 cm from needle tip to the collection screen, 19 kV of high voltage, and 1.5 mL h−1 flow rate of the spinning solution. The entire electrospinning process was carried out at 25 °C and 35% relative humidity environment. Then, 50 mg of PS/PES-Cu2+ composite nanofibers obtained on the collection screen were soaked in 20 mL of fresh aqueous solution of NaBH4 (1.0 mol L−1) for 48 hours at room temperature. The surface color of the nanofibers gradually changed from light blue to brown-black. After the reaction, the fibers were washed with ultrapure water three times and dried in the constant temperature drying oven at 50 °C for 20 min. Finally, 10% (w/v) PS/5% (w/v) PES-5% (w/v) CuNP nanofibers were obtained, and, similarly, 10% (w/v) PS-5% (w/v) CuNP nanofibers, 5% (w/v) PS/5% (w/v) PVP-5% (w/v) CuNP nanofibers, and 5% (w/v) PS/5% (w/v) PAN-5% (w/v) CuNP nanofibers were also prepared with PS, PVP, PAN, and copper acetate by the same operation.
2.4 Sample collection
The morning urine samples of 30 participants, including 15 females and 15 males, all aged between 24 and 25 years old, living in Nanjing (China), were collected. Consent for sampling was obtained from all participants.
The sample processing method was based on previous research29 slightly modified. The collected samples were stored at −20 °C for future use. Before analysis, the sample was thawed, then frozen and thawed again until it reached room temperature. It was centrifuged at a speed of 12
000 rpm min−1 for 15 min and the supernatant was retained for subsequent PFSPE.
2.5 Nanofiber solid-phase extraction
In the SPE experiment, 5.0 mg of nanofibers were weighed and installed into a specially designed SPE column using a steel rod (1.0 mm diameter) to prepare PFSPE columns. The PFSPE column was assembled onto a semi-automatic SPE processor which can pretreat 12 samples simultaneously.
After that, the fibers in PFSPE columns were activated by 150 μL each of methanol and water, separately. For extraction, 500 μL of standard solution or sample supernatant was added to the PFSPE column and uniformly pushed out of the column at a speed of 4–5 seconds per drop by rotating the pressure rod to drive the booster. The targets were captured by the filled adsorbent when the solution passed through the PFSPE column. After the adsorption process completed, 100 μL of MeOH/ACN/HAc/H2O (40
:
50
:
5
:
5, v/v/v/v) was used to elute the target adsorbed on the nanofibers. Finally, the eluate was treated with a 0.22 μm filter membrane and then injected into the HPLC for further analysis. The process of PFSPE of the three ochratoxins is shown in Fig. 1.
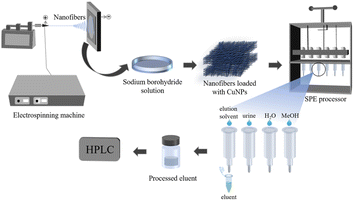 |
| Fig. 1 Schematic illustration of PFSPE process and detection of OTs. | |
2.6 HPLC analysis conditions
The analysis of the targets was accomplished on a Waters 2695 high-performance liquid chromatograph equipped with a Waters 2475 fluorescence detector. The separation of the target substance was performed on an InsertSustain C18 column (150 mm × 4.6 mm, 5 μm). HPLC operation conditions were set as follows: mobile phase: 1% acetic acid water
:
acetonitrile (45
:
55, v/v); elution method: isocratic elution; flow rate: 1.0 mL min; column temperature: 40 °C; injection amount: 20 μL. The optimal fluorescence responses of the three ochratoxins were obtained by setting excitation wavelength and emission wavelength to 333 nm and 460 nm, respectively.
2.7 Method validation
2.7.1 Linearity, LOD, and LOQ. The series standard working solutions were made by adding three ochratoxins to blank artificial urine and were used to construct standard curves. Each point on the calibration curve corresponds to the average value of three independent repeated experiments. The detection limit (LOD) and quantification limit (LOQ) of each target were defined as the concentrations corresponding to signal-to-noise ratios of 3 and 10,30 respectively.
2.7.2 Recovery. The recovery and intra-day and inter day precision were tested by the blank sample spiking method. The intra-day precision was evaluated by the relative standard deviation of the high, medium, and low concentration levels for 1 day, and the inter day precision was evaluated by the relative standard deviation of the results obtained from continuous measurements for 5 days.The extraction recovery was evaluated by comparing the total targets of the elution solution and that of the sample solution before PFSPE.14 The equation of recovery was as follows,
|
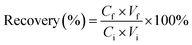 | (1) |
where
Ci and
Vi are the added concentration (μg L
−1) of OTs and the volume of spiked solution before PFSPE treatment, respectively, and
Cf and
Vf are the obtained concentration (μg L
−1) of OTs and the volume of eluent after PFSPE treatment, respectively.
3 Results and discussion
3.1 Characterization of nanofibers
The morphology of the nanofibers was studied by SEM and TEM. As shown in Fig. 2, SEM images of all four nanofibers showed good fiber morphology at a submicron level. The copper nanoparticles synthesized on the surface of the nanofibers exhibited a uniform particle state without aggregation. This property of nanofibers was speculated have significant impact on the extraction efficiency of OTs.25
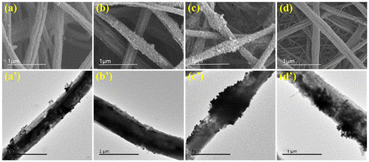 |
| Fig. 2 SEM and TEM images of PS-CuNP nanofibers (a and a’), PS/PVP-CuNP nanofibers (b and b’), PS/PAN-CuNP nanofibers (c and c’) and PS/PES-CuNP nanofibers (d and d’). | |
The elemental content of the composite nanofibers was measured by EDS spectroscopy analysis. As shown in Fig. 3, the EDS spectra of four fibers confirmed the successful synthesis of copper nanoparticles on the surface of the nanofibers. The EDS spectrum of PS/PES-CuNP nanofibers showed a higher presence of Cu element than the other nanofibers, which might be closely related to the high adsorption efficiency of this adsorbent.25
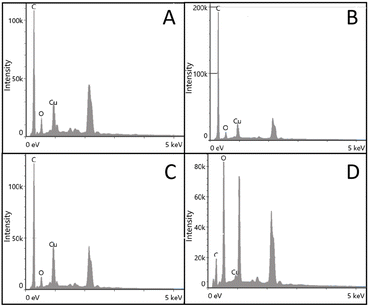 |
| Fig. 3 EDS spectra of PS-CuNP nanofibers (A), PS/PVP-CuNP nanofibers (B), PS/PAN-CuNP nanofibers (C) and PS/PES-CuNP nanofibers (D). | |
FT-IR spectroscopy also confirmed the successful incorporation of PVP, PAN and PES into the nanofibers, as shown in Fig. 4A. The four types of nanofibers exhibited spectral bands around 2960 and 1647 cm−1, corresponding to the C–H stretching vibration and the C
O tensile vibration,31 respectively, which is a characteristic of PS. For the PS/PES-CuNP nanofibers, the peaks at 1090 and 1150 cm−1 were attributed to the absorption peaks of C
O
C and its related groups,32 and the peak at 1240 cm−1 was attributed to the C–O vibration caused by the C–O–C related groups adjacent to the benzene ring.33 In the spectrum of the PS/PAN-CuNP nanofibers, the characteristic absorption peak at 1454 cm−1 is attributed to the C–H stretching vibration.34 The spectra of PS/PVP-CuNP nanofibers exhibited significant peaks at 1289 cm−1 caused by the vibration of the C–N bonds in the PVP material.30
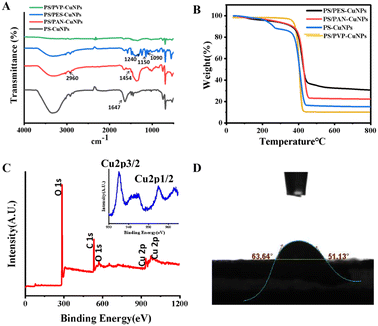 |
| Fig. 4 (A) FTIR spectra of PS-CuNPs, PS/PVP-CuNPs, PS/PAN-CuNPs and PS/PES-CuNP nanofibers. (B) Thermal weight loss curves (TG) of PS-CuNPs, PS/PVP-CuNPs, PS/PAN-CuNPs and PS/PES-CuNP nanofibers. (C) X-ray photoelectron spectra (XPS) of PS/PES-CuNP nanofibers, and the inset is the Cu2p spectrum. (D) Contact angle measurement (CA) of water droplet on the surface of PS/PES-CuNP nanofibers. | |
As shown in Fig. 4B, with the increase of temperature, the weight loss of the four nanomaterials gradually increased as the coordinated water molecules were removed. The quick weight loss at 420 °C of the four nanofibers is attributed to the collapse of the CuNPs structure.25 When the temperature was 800 °C, the weight loss rates of the PS-CuNPs, PS/PVP-CuNPs, PS/PAN-CuNPs and PS/PES-CuNP nanofibers reached 16.1%, 7.6%, 22.4% and 30.1%, respectively. As shown in Fig. 4C, the binding energies of 527.4 and 281.7 eV correspond to the photoelectron spectra of C1s and O1s,14 respectively, and the core-level energy photoelectron peaks of Cu2p1/2 and 2p3/2 were exhibited at 754.1 and 735.5 eV, which implies the successful synthesis of CuNPs.25
3.2 Evaluation of PS/PES-CuNPs as an excellent adsorbent for ochratoxins
The impact of nanofibers with different compositions on the simultaneous adsorption of ochratoxins was researched by comparing the adsorption efficiencies of PS/PES-CuNPs and PS-CuNPs, PS/PVP-CuNPs, and PS/PAN-CuNP nanofibers. Specifically, 5 mg of nanofibers filled the SPE column and 500 μL of spiked artificial urine (5 μg L−1 for each type of ochratoxin) was treated with the method described in Section 1.4. The concentration of ochratoxins was determined by the HPLC method described in Section 1.5. As Fig. 5 shows, the adsorption recoveries of PS-CuNPs, PS/PVP-CuNPs, and PS/PAN-CuNP nanofibers for the three ochratoxins only achieved 20.1–67.4%, while the recovery of PS/PES-CuNPs significantly increased to 71.2–78.9%. Therefore, it can be confirmed that PES and Cu modification can contribute to significant improving the adsorption efficiency of the three ochratoxins.
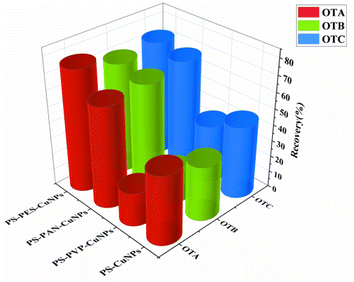 |
| Fig. 5 Comparison of adsorption efficiencies for ochratoxins among four types of nanofibers. | |
3.3 Optimization of conditions in the PFSPE process
3.3.1 Amount of adsorbent PS/PES-CuNPs. The adsorption mechanism of the nanofibers for the targets depends on the hydrophobic interaction and electrostatic interaction between PS/PES CuNP nanofibers and the carboxylic acid esters of the ochratoxins.25 Therefore, the amount of nanofibers directly affected the adsorption efficiency of the PFSPE columns. As shown in Fig. 6A, when the dosage of adsorbent increased from 1 mg to 3 mg, the extraction recovery of ochratoxins significantly enhanced, indicating that a larger filling amount would improve adsorption efficiency. As the dosage of PS/PES-CuNP nanofibers increased to 5 mg and 8 mg, the recoveries of the three targets reached 69.5–73.2%, and a significant difference in adsorption efficiency between 5 mg and 8 mg of nanofibers was not seen. Based on the experimental results, 5 mg was chosen as the optimal filling amount for PS/PES CuNP nanofibers.
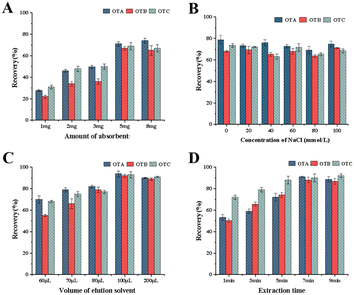 |
| Fig. 6 Optimization of PFSPE (n = 3): (A) amount of absorbent, (B) ion species, (C) volume of elution solvent and (D) extraction time. | |
3.3.2 Effect of ionic strength. The ion effect in a sample solution is crucial to the adsorption efficiency. The extraction of the nanofibers to OTs could be affected by the salt concentration because of the salting-out and competition effects in sample solutions.28 The adsorption performance of PS/PES-CuNP nanofibers was investigated with 0–100 mmol L−1 NaCl solution. As shown in Fig. 6B, apparent improvement of recovery for the three ochratoxins was not observed as the concentration of NaCl increased; this result revealed that PS/PES-CuNP nanofibers possess a great anti-interference property. Considering the achieved results, there is no NaCl added in the subsequent experiments.
3.3.3 Effect of desorbent volume. The volume of desorbent is an important factor in optimizing PFSPE, and can significantly affect the desorption efficiency of the targets from PS/PES-CuNP nanofibers. As shown in Fig. 6C, different levels of desorbent volume were examined (60 μL, 70 μL, 80 μL, 100 μL, and 200 μL). As the volume of desorbent increased within 60–80 μL, the extraction efficiency gradually improved. When the desorbent volume reached 100 μL, the best extraction efficiency was achieved, and the recovery of three ochratoxins was 92.1–94.7%. As the volume of the desorbent further increased, there was no significant improvement in the extraction efficiency. For environmental protection and cost saving considerations, 100 μL was chosen as the volume of desorbent for PFSPE.
3.3.4 Effect of adsorption time. The adsorption time is described as the required time for each active site on the adsorbent to be maximally occupied by the targets. The influence on extraction efficiency by adsorption time was investigated in this experiment. As shown in Fig. 6D, the extraction efficiency significantly improves with the extension of extraction time and achieved saturation at 7 min; the extraction recovery of the three ochratoxins reached 88.1–92.7%. The adsorption rate slightly changed but basically reached equilibrium as the extraction time was extended. This result indicated that the three ochratoxins can quickly accumulate on PS/PES CuNP nanofibers. Therefore, the optimal extraction time was determined to be 7 min for subsequent experiments.
3.4 Method validation
The calibration curve in a concentration range of 0.5–50 μg L−1 was obtained by diluting the standard stock solution of ochratoxins with artificial urine, as shown in Table 1. The standard curves of the three ochratoxins showed good linearity (R2 ≥ 0.992) within the specified concentration range. LOD and LOQ were 0.108–0.162 μg L−1 and 0.658–0.701 μg L−1, respectively.
Table 1 Figures of merit for the PS/PES-CuNP nanofibers as sorbent for HPLC-FLD determination of OTs
Analyte |
Linearity (μg L−1) |
Linear equation |
R2 |
LOD (μg L−1) |
LOQ (μg L−1) |
OTA |
0.5–50.0 |
y = 4.3006x − 1.0130 |
0.992 |
0.162 |
0.701 |
OTB |
0.5–50.0 |
y = 3.9075x − 1.4198 |
0.992 |
0.108 |
0.658 |
OTC |
0.5–50.0 |
y = 11.1675x − 3.4206 |
0.989 |
0.147 |
0.682 |
The recovery was verified by adding standards to artificial urine, and three concentration levels of low, medium, and high (1.0, 5.0, and 10.0 μg L−1) for the targets were obtained. The absolute recovery was calculated as the ratio of the amounts of targets in the elution solution to that in the spiked solution before PFSPE.12 As shown in Table 2, for the low, medium, and high concentrations of ochratoxins in spiked artificial urine samples, the recoveries were 71.3–92.0%, indicating that satisfactory extraction efficiency of ochratoxins was achieved in the urine sample. The intra-day and inter-day precision were both less than 7.1%. Overall, the detection method established in this experiment is sensitive and accurate and can be used for the detection of ochratoxins in human urine.
Table 2 Analytical parameters of the proposed method (n = 5)
Analyte |
Spiked concentration (μg L−1) |
RSDs (%) |
Recovery (%) |
Intra-day |
Inter-day |
OTA |
1.0 |
5.3 |
1.5 |
89.5–92.0 |
5.0 |
2.9 |
4.2 |
88.9–90.1 |
10.0 |
3.2 |
4.4 |
78.2–86.0 |
OTB |
1.0 |
2.9 |
2.8 |
84.9–89.3 |
5.0 |
5.1 |
5.6 |
85.7–91.1 |
10.0 |
1.9 |
3.9 |
71.3–85.0 |
OTC |
1.0 |
2.1 |
3.6 |
84.6–90.7 |
5.0 |
3.8 |
2.8 |
87.3–89.9 |
10.0 |
5.6 |
7.1 |
77.0–86.3 |
The chromatogram of the ochratoxins is shown in Fig. S1.† After PFSPE treatment, most of the interfering matrix in the urine sample was removed, and the response of the targets in urine was significantly enhanced. This result indicated that PS/PES CuNPs composite nanofibers have a good ability for impurity removal and selective adsorption for ochratoxins.
3.5 Comparison between PS/PES CuNP nanofibers and reported adsorbents
At present, there are several articles on methods for extracting OTs from food matrices. As displayed in Table S1,† the proposed method was comparable to most techniques in terms of adsorbent filled, organic solution usage, minimum detection line, recovery, and a shorter extraction time (5–6 min), which was not mentioned in most articles. As seen from the main methodological parameters of each method listed in Table S1,† the proposed method has the advantages of simple preparation of adsorbent materials, high detection sensitivity, good recovery rate, and low detection limit. Moreover, three ochratoxins can be extracted simultaneously from complex matrices. Therefore, the detection method provided in this study shows great promise for application in the accurate quantitative analysis of OTs in complicated samples.
3.6 Actual sample analysis
3.6.1 Matrix effect evaluation. The practical application of PS/PES CuNP nanofibers was evaluated by testing non-invasive human samples with the analytical method developed. Similar to urine samples, saliva contains many complex substances, such as proteins and fats, which may interfere or prevent the accurate detection of targets. The reserve solution was diluted with methanol, blank saliva, and blank artificial urine, separately. All solutions had internal standards added and were processed and analyzed according to procedures 2.5 and 2.6. The matrix effect (ME) of the sample solution was evaluated using the following equation,29 |
ME (%) = (S1/S2) × 100
| (2) |
where S1 is the slope of the matrix matching the calibration curve and S2 is that of the calibration standard in the standard solution (methanol).As shown in Table 3, the results showed that the matrix effects of ochratoxins in artificial urine samples and saliva samples were 89.6–112.8% and 96.1–110.9%, respectively. A value of ME from 85.0–115.0% can be considered to have a negligible matrix effect,35 indicating that the influences of saliva and urine matrices were well controlled.
Table 3 Evaluation of matrix effect and analytical results of three OTs in samples
Analytes |
Matrix |
Slope (104) |
Slope matrix/solvent |
ME % |
OTA |
Methanol |
3.8137 |
|
|
Urine |
4.3006 |
1.128 |
112.8 |
Saliva |
3.6641 |
0.961 |
96.1 |
OTB |
Methanol |
3.9020 |
|
|
Urine |
3.9075 |
1.002 |
100.2 |
Saliva |
4.3269 |
1.109 |
110.9 |
OTC |
Methanol |
12.4701 |
|
|
Urine |
11.1675 |
0.896 |
89.6 |
Saliva |
13.4930 |
1.082 |
108.2 |
3.6.2 Analysis of ochratoxins in actual human urine samples. The applicability of the PS/PES-CuNP nanofibers coupled with HPLC was investigated by analyzing 30 actual urine samples (15 males and 15 females). The test results are shown in Table S2 in ESI.† In the 15 male samples, 3 positive samples were detected to have been exposed OTA at different levels (0.96 μg L−1, 1.64 μg L−1, 0.52 μg L−1), and one positive sample was detected of OTC at 0.31 μg L−1. The detection rate in females is lower than that in males: only OTC was detected, with concentrations of 0.48 μg L−1 and 0.33 μg L−1 in two females' positive urine samples. Overall, PS/PES-CuNP nanofibers can achieve simultaneous adsorption of three ochratoxins in human urine samples and showed great potential on pretreatment for large amounts of human samples.
4 Conclusion
In this work, a new strategy was proposed that used polystyrene/polyethersulfone electrospun nanofibers coated with copper nanoparticles as an excellent adsorbent for the rapid and sensitive extraction of ochratoxins from human urine. The nanofibers were characterized by TEM, SEM, XPS, EDS, TG, XPS, FTIR and CA. The results proved that the copper nanoparticles with positive charges were successfully integrated onto the PS/PES nanofibers, which played a key role in the sample pretreatment for extracting ochratoxins. The nanofiber-packed solid-phase extraction (PFSPE) requires less time, less sorbent dosage, and less volumes of sample and eluent than classic solid-phase extraction techniques and has greater application potential. In addition, an analytical method based on PFSPE was proposed, and extraction factors, such as adsorbent amount, ionic strength, adsorption time and desorbent volume, were optimized. Under optimal conditions, the novel PFSPE-HPLC-FLD method was established and utilized to detected ochratoxins in actual human urine samples, and OTs were detected in 5 positive samples of 30 samples. The results indicated that the novel PFSPE-HPLC-FLD method is sensitive, simple, low-cost and time-saving and suitable to trace and monitor mycotoxins in complex matrix samples.
Data availability
The data supporting this article have been included as part of the ESI.†
Conflicts of interest
There are no conflicts of interest to declare.
Acknowledgements
This work was supported by Yantai Key Laboratory of Special Medical Food (Preparatory), the Guangdong Foundation for Program of Science and Technology Research (No. 2023B1212060049), National Natural Science Foundation of China (No. 82003507).
References
- K. J. van der Merwe, P. S. Steyn, L. Fourie, D. B. Scott and J. J. Theron, Ochratoxin A, a toxic metabolite produced by Aspergillus ochraceus Wilh, Nature, 1965, 205(976), 1112–1113 CrossRef CAS PubMed.
- A. Ochratoxin, IARC monographs on the evaluation of carcinogenic risks to humans, 1993, vol. 56, pp. 489–521 Search PubMed.
- T. R. Bui-Klimke and F. Wu, Ochratoxin A and Human Health Risk: A Review of the Evidence, Crit. Rev. Food Sci. Nutr., 2015, 55(13), 1860–1869 CrossRef CAS PubMed.
- Y. H. Sun, Y. Q. Song, M. Long and S. H. Yang, Immunotoxicity of Three Environmental Mycotoxins and Their Risks of Increasing Pathogen Infections, Toxins, 2023, 15(3), 13 CrossRef PubMed.
- C. A. Tatu, W. H. Orem, R. B. Finkelman and G. L. Feder, The etiology of Balkan endemic nephropathy: still more questions than answers, Environ. Health Perspect., 1998, 106(11), 689–700 CrossRef CAS PubMed.
- A. P. Grollman, S. Shibutani, M. Moriya, F. Miller, L. Wu and U. Moll, Aristolochic acid and the etiology of endemic (Balkan) nephropathy, Proc. Natl. Acad. Sci. U. S. A., 2007, 104(29), 12129–12134 CrossRef CAS PubMed.
- T. Lincoln, Toxicology - Danger in the diet, Nature, 2007, 448(7150), 148 CrossRef CAS PubMed.
- Y. Cui, H. Ma, D. Liu, M. Li, R. Hao, J. Li and Y. Jiang, Graphene Oxide Adsorbent-Based Dispersive Solid Phase Extraction Coupled with Multi-pretreatment Clean-up for Analysis of Trace Ochratoxin A in Chicken Liver, Chromatographia, 2020, 83(10), 1307–1314 CrossRef CAS.
- K. P. Mabunda, B. R. Maseko and S. Ncube, Development and application of a new QuEChERS-molecularly imprinted solid phase extraction (QuEChERS-MISPE) technique for analysis of DDT and its derivatives in vegetables, Food Chem., 2024, 436, 8 Search PubMed.
- H. J. Jiang, S. X. Yang, H. Y. Tian and B. G. Sun, Research progress in the use of liquid-liquid extraction for food flavour analysis, Trends, J. Food Sci. Technol., 2023, 132, 138–149 CrossRef CAS.
- B. B. Zhu, H. Y. Qiu, C. L. Ma, S. L. Chen, J. C. Zhu and S. Q. Tong, Recent progress on chiral extractants for enantioselective liquid-liquid extraction, J. Chromatogr. A, 2023, 1709, 14 CrossRef PubMed.
- S. Palmieri, D. Elfadil, F. Fanti, F. Della Pelle, M. Sergi, A. Amine and D. Compagnone, Study on Molecularly Imprinted Polymers Obtained Sonochemically for the Determination of Aflatoxins in Food, Molecules, 2023, 28(2), 703 CrossRef CAS PubMed.
- F. Kardani, R. Mirzajani, Y. Tamsilian, A. Kiasat and F. B. Farajpour, A novel immunoaffinity column based metal-organic framework deep eutectic solvents @ molecularly imprinted polymers as a sorbent for the solid phase extraction of aflatoxins AFB1, AFB2, AFG1 and AFG2 from cereals samples, Microchem. J., 2023, 187, 13 CrossRef.
- H. Xu, J. Sun, H. Wang, Y. Zhang and X. Sun, Adsorption of aflatoxins and ochratoxins in edible vegetable oils with dopamine-coated magnetic multi-walled carbon nanotubes, Food Chem., 2021, 365, 130409 CrossRef CAS PubMed.
- D. Wei, A. Pan, C. Zhang, M. Guo, C. Lou, J. Zhang, H. Wu and X. Wang, Fast extraction of aflatoxins, ochratoxins and enniatins from maize with magnetic covalent organic framework prior to HPLC-MS/MS detection, Food Chem., 2023, 404, 134464 CrossRef CAS PubMed.
- X. S. Yang, J. Zhao, T. T. Ma, Z. Y. Li, L. L. Wang, S. L. Ji, M. Y. Sun, Y. S. Liu, Z. H. Hu, Q. W. Liu, C. W. Jin, S. Y. Sun and H. S. Gong, Magnetic covalent organic framework for effective solid-phase extraction and HPLC determination of ochratoxin A in food, Lwt, 2023, 179, 114639 CrossRef CAS.
- E. V. S. Maciel, A. L. de Toffoli, E. S. Neto, C. E. D. Nazario and F. M. Lanças, New materials in sample preparation: Recent advances and future trends, Trends Anal. Chem., 2019, 119, 115633 CrossRef CAS.
- L. H. Rong, X. Cheng, J. Ge, K. K. Olivia, R. Jeffrey and B. C. Eugene, Synthesis of hyperbranched polymer films via electrodeposition and oxygen-tolerant surface-initiated photoinduced polymerization, J. Colloid Interface Sci., 2023, 637, 33–40 CrossRef CAS PubMed.
- J. P. Fan, J. J. Luo, X. H. Zhang, B. Zhen, C. Y. Dong, Y. C. Li, J. Shen, Y. T. Cheng and H. P. Chen, A novel electrospun β-CD/CS/PVA nanofiber membrane for simultaneous and rapid removal of organic micropollutants and heavy metal ions from water, Chem. Eng. J., 2019, 378, 122232 CrossRef CAS.
- J. Xue, T. Wu, Y. Dai and Y. Xia, Electrospinning and Electrospun Nanofibers: Methods, Materials, and Applications, Chem. Rev., 2019, 119(8), 5298–5415 CrossRef CAS.
- S. Amini, H. Ebrahimzadeh, S. Seidi and N. Jalilian, Preparation of Polyacrylonitrile/Ni-MOF electrospun nanofiber as an efficient fiber coating material for headspace solid-phase microextraction of diazinon and chlorpyrifos followed by CD-IMS analysis, Food Chem., 2021, 350, 129242 CrossRef CAS PubMed.
- H. Yang, M. J. Lu, D. Chen, R. Y. Chen a, L. Li and W. Han, Efficient and rapid removal of Pb2+ from water by magnetic Fe3O4@MnO2 core-shell nanoflower attached to carbon microtube: Adsorption behavior and process study, J. Colloid Interface Sci., 2020, 563, 218–228 CrossRef CAS PubMed.
- D. Sarma, K. K. Nath, S. Biswas, I. Chetia, L. S. Badwaik, G. A. Ahmed and P. Nath, SERS determination and multivariate classification of antibiotics in chicken meat using gold nanoparticle–decorated electrospun PVA nanofibers, Microchim. Acta, 2023, 190(2), 64 CrossRef CAS.
- Y. J. Hou, N. Long, Q. B. Xu, Y. Li, P. Y. Song, M. H. Yang, J. Wang, L. D. Zhou, P. Sheng and W. J. Kong, Development of a Nafion-MWCNTs and in-situ generated Au nanopopcorns dual-amplification electrochemical aptasensor for ultrasensitive detection of OTA, Food Chem., 2023, 403, 134375 CrossRef CAS PubMed.
- L. Wei, Y. Song, P. Liu and X. Kang, Polystyrene nanofibers capped with copper nanoparticles for selective extraction of glutathione prior to its determination by HPLC, Microchim. Acta, 2018, 185(7), 134375 CrossRef.
- L. L. Chu, J. J. Deng and X. J. Kang, Packed-nanofiber solid phase extraction coupled with HPLC for the determination of chloramphenicol in milk, Anal. Methods, 2017, 9(46), 6499–6506 RSC.
- Y. Fang, F. Zhou, Q. Zhang, C. Deng, M. Wu, H. h. Shen, Y. Tang and Y. Wang, Hierarchical covalent organic framework hollow nanofibers-bonded stainless steel fiber for efficient solid phase microextraction, Talanta, 2024, 267, 125223 CrossRef CAS PubMed.
- L. L. Chu and X. J. Kang, Adsorption/Desorption Performance of Electrospun Nanofibers on Volatile Sulfur Compounds from Onion Juice, Nanosci. Nanotechnol. Lett., 2019, 11(6), 776–783 CrossRef.
- Y. Sun, Y. Yan and X. Kang, Packed-Fiber Solid Phase-Extraction Coupled with HPLC-MS/MS for Rapid Determination of Lipid Oxidative Damage Biomarker 8-Iso-Prostaglandin F2α in Urine, Molecules, 2022, 27(14), 4417 CrossRef CAS PubMed.
- Y. Z. Wang, C. Hou, Y. Q. Dai, L. L. Chu, S. W. Geng, S. L. Zheng and X. J. Kang, Determination of aflatoxin B1 by novel nanofiber-packed solid-phase extraction coupled with a high performance liquid chromatography-fluorescence detector, Anal. Methods, 2023, 15(4), 472–481 RSC.
- Z. Deji, X. Zhang, P. Liu, X. Wang, K. Abulaiti and Z. Huang, Electrospun UiO-66-F4/polyacrylonitrile nanofibers for efficient extraction of perfluoroalkyl and polyfluoroalkyl substances in environmental media, J. Hazard. Materials, 2022, 430, 128494 CrossRef CAS PubMed.
- Y. S. Lee, D. H. Park, S. H. Park, Y. H. Gu, D. Lim, S. B. Han and K. W. Park, Lithium-Ion Exchange Membrane Water Electrolysis Using a Cationic Polymer-Modified Polyethersulfone Membrane, ACS Sustain. Chem. Eng., 2023, 11(27), 10183–10190 CrossRef CAS.
- X. Zheng, C. J. Ni, W. W. Xiao, G. P. Yu and Y. J. Li, In vitro hemocompatibility and hemodialysis performance of hydrophilic ionic liquid grafted polyethersulfone hollow fiber membranes, Sep. Purif. Technol., 2022, 298, 121464 CrossRef CAS.
- S. Liu, P. P. Liang, J. Liu, J. Y. Xin, X. H. Li, C. L. Shao, X. W. Li and Y. C. Liu, Anchoring bismuth oxybromo-iodide solid solutions on flexible electrospun polyacrylonitrile nanofiber mats for floating photocatalysis, J. Colloid Interface Sci., 2022, 608(3), 3178–3191 CrossRef CAS PubMed.
- S. Tisler, D. I. Pattison, J. H. Christensen and H. Jan, Correction of Matrix Effects for Reliable Non-target Screening LC-ESI-MS Analysis of Wastewater, Anal. Chem., 2021, 93(24), 8432–8441 CrossRef CAS PubMed.
|
This journal is © The Royal Society of Chemistry 2024 |
Click here to see how this site uses Cookies. View our privacy policy here.