DOI:
10.1039/D4RA05238B
(Paper)
RSC Adv., 2024,
14, 32883-32892
Functionalizable poly-terthiophene/Cu2O heterojunction constructed in situ for sensitive photoelectrochemical detection of long non-coding RNA markers†
Received
19th July 2024
, Accepted 11th October 2024
First published on 18th October 2024
Abstract
A photoelectrochemical (PEC) sensor based on the poly-2,2,5,2-terthiophene (pTTh)/Cu2O heterojunction was constructed and applied for the detection of long non-coding RNA (lncRNA) TROJAN, a biomarker of triple-negative breast cancer. Cu2O and pTTh were electrodeposited in situ and sequentially onto an indium tin oxide substrate. The bandgap of the resultant type II heterojunction was measured spectroscopically and the morphology was found to effectively separate photogenerated holes from electrons. A photocurrent density as high as 250 μA cm−2 was attained, which is about three times higher than those of only pTTh or Cu2O. Owing to the close contact between pTTh and Cu2O, this PEC sensor is highly stable. Oligonucleotide probes for lncRNA can be cross-linked to carboxyl moieties of mercaptopropionic acid molecules adsorbed on pTTh/Cu2O. The desirable band structure and the high density of probe molecules collectively yielded a linear range of 0.1–10
000 pM. Our PEC sensor has been demonstrated to be amenable for detection of lncRNA markers with excellent analytical performance.
1 Introduction
With improved diagnoses and modalities, early identification and precise treatments of cancers have become possible and the survival rates of patients are enhanced.1,2 Methods such as enzyme-linked immunosorbent assay,3 fluorescence,4,5 surface-enhanced Raman scattering,6,7 surface plasmon resonance,8,9 electrochemical methods,10–14 real-time quantitative polymerase chain reaction,15 and various electrochemical methods10 have shown promise in cancer biomarker detection. Many studies have reported that long non-coding RNAs (lncRNAs) carried by exosomes can be used to diagnose cancer subtypes and assess prognosis.16–20 Recent research has shown that a lncRNA termed as TROJAN is expressed in exosomes and cells of the triple-negative breast cancer.20 Its expression is associated with poor prognosis of breast cancer patients.21 TROJAN has a low abundance of 246–361 copies per cell in certain cell lines,22 which is a challenge for early cancer detection and diagnosis. This is one of the reasons that few sensing methods have been developed to quantify TROJAN.
Among the different electrochemical methods, photoelectrochemical (PEC) detection is an attractive variant owing to its superior sensitivity.23 A key step in the PEC detection is the efficient separation of the photogenerated holes from their electron counterpart. This aspect is the same as that essential to the performance of photocatalysts,24 photoelectronic components,25,26 and memory devices.26 Hence, for enhancing their performance, different types of heterojunctions have been developed.25,26 Type II heterojunction is arguably one of the most widely used in the aforementioned applications because its staggered band structure affords a better spatial hole/electron separation than type I heterojunction and others.24,27 We should note that the lowered redox activity of type II heterojunctions are not problematic in the PEC detection because an easily oxidizable species can be added into the sample solution according to the valence band position. Although a number of heterojunctions have also been incorporated in the PEC detection, the predominant ones are constructed with two inorganic compounds of different bandgaps. Attaching heterojunctions synthesized ex situ to the substrate electrode for PEC detection is generally inconvenient or not highly controllable. For those that can be produced in situ, the preparative method (e.g., chemical vapor deposition28,29) is cumbersome or requires expensive devices (e.g., atomic layer deposition30). Moreover, for heterogeneous biosensors requiring pre-immobilized capture probes, heterojunctions based on inorganic compounds need to be modified with an organic or polymeric layer to afford anchoring sites. We envision that an inorganic/organic heterojunction constructed in situ should be advantageous because (1) the same instrument used for the PEC detection can also be used to electrodeposit the heterojunction constituents, reducing the assay cost; (2) the preparation is straightforward and controllable; and (3) if the organic compound is the topmost layer, it can be functionalized for subsequent probe attachment. Furthermore, if the organic layer is a conducting polymer, the photogenerated electrons can rapidly transfer inside because of the good conductivity.
In this work, we constructed in situ a type II heterojunction via sequential electrodepositions of Cu2O and poly-2,2,5,2-terthiophene (pTTh) onto an indium tin oxide (ITO) electrode for sensitive PEC detection. Cu2O is a p-type inorganic semiconductor31 with a narrow bandgap and photosensitivity in the visible light region.32 Poly-thiophene and its derivative such as pTTh are among the various polymers used for heterojunction fabrication and modification. However, each compound alone is not effective in preventing the hole/electron recombination. As demonstrated below, the underlying Cu2O nanocubes are highly uniform and the upper pTTh film has a good surface coverage. Although heterojunctions comprising Cu2O and conducting polymers have been firmly established as of type II, they have been mainly used in photocatalysis and photodevice fabrications.33,34 For those used in (bio)chemical sensing, they were either not used in conjunction with the PEC detection35,36 or needed further derivatization with a film to confer molecular recognition (e.g., molecular imprinting matrices37,38) or yielding a detection signal specific to the target molecule. To the best of our knowledge, few studies have employed a conducting polymer-based heterojunction onto which probe molecules can be linked for sensitive detection of disease biomarkers. We measured the bandgap of this heterojunction with UV-visible spectroscopy and deduced the valence and conduction band positions of Cu2O and pTTh through X-ray photoelectron spectroscopy (XPS) and Mott–Schottky measurements. The uniformity of deposited Cu2O and the excellent pTTh coverage are effective in preventing the hole/electron recombination. The combination of hole/electron separation and immobilization of oligonucleotide probes resulted in a PEC sensor that can accurately detect lncRNA at fM levels, affording an alternative method for early typing diagnosis of triple-negative breast cancer.
2 Materials and methods
2.1. Reagents and materials
2,2,5,2-Terthiophene (TTh, 99.0%), acetonitrile, N-hydroxy-succinimide (NHS, ≥99.9%) and 1-ethyl-3-(3-dimethyl-aminopropyl) carbodiimide hydrochloride (EDC, 99.0%) were purchased from Aladdin Biochemical Technology Co., Ltd (Shanghai, China). Copper sulfate (CuSO4, 98.0%) and lactic acid (85.0%) were acquired from Nine-Dinn Chemistry Co., Ltd (Shanghai, China). Lithium perchlorate (LiClO4, AR, ≥99.0%) and sodium hydroxide (NaOH, AR, ≥99.0%) were obtained from Sinopharm Group Chemical Reagent Co., Ltd (Beijing, China). 3-Mercaptopropionic acid (MPA, 99.0%) and sodium chloride (NaCl, 99.8%) were purchased from Shanghai Macklin Biochemical Co., Ltd Indium tin oxide (ITO)-coated glass substrates were obtained from Zhuhai Kaivo Optoelectronic Technology Co., Ltd (Zhuhai, China). All lncRNAs were synthesized by BoShang Biotech Co., Ltd (Shanghai, China). The RNeasy Plus Mini Kit was purchased from Qiagen Co., Ltd (German).
2.2. Equipment
X-ray diffraction (XRD) patterns were collected from a smart lab se X-ray diffractometer (Japan Electric Equipment Co., Ltd, Beijing, China). The elemental analysis data were obtained from an Escalab 250Xi X-ray photoelectron spectroscopic instrument (Seymour Fisher Technology Co., Ltd, Waltham, Massachusetts, USA). Fourier transform-infrared spectra (FT-IR) were recorded with a Bruker-Alpha infrared spectrometer (Bruker Co., Ltd, Billerica, Massachusetts, USA). Scanning electron microscopic (SEM) images and energy dispersive spectra (EDS) were acquired with an SU8010 microscope (Hitachi Co., Ltd, Tokyo, Japan). The TEM images were collected from a JEOL microscope (JEM-2100, Nippon Electronics Co., Ltd, Tokyo, Japan). Ultraviolet-visible absorption spectroscopy (UV-vis) spectra were obtained with a UV-Vis spectrophotometer (Shimadzu Instrument Co., Ltd, Suzhou, China).
2.3. Synthesis of the photoactive materials
Cu2O were electrochemically deposited in a plating bath containing 0.4 M CuSO4 and 3 M lactic acid.39 Prior to the process, the electrode substrate was ultrasonically cleaned with acetone, isopropanol, ethanol, and water in sequence (40 min each). The pH value of the plating bath was adjusted to 11.0 with NaOH and the temperature was maintained at 60 °C. The potential was kept at −0.4 V (vs. Ag/AgCl) for 30 min. Finally, the substrate was rinsed with deionized water and dried at 37 °C.
pTTh films were electropolymerized onto Cu2O. Specifically, the pTTh/Cu2O heterojunction was prepared using cyclic voltammetry (CV) in an acetonitrile solution containing 10 mM TTh and 0.1 M LiClO4. Ten CV cycles were conducted within the potential range from 0.0 to 1.2 V (vs. Ag/AgCl) at a scan rate of 25 mV s−1. Each electrode was rinsed with acetonitrile and deionized water and dried in an oven at 37 °C.
2.4. PEC sensor construction
Each substrate electrode was coated with the pTTh/Cu2O heterojunction and further modified through immersion in a 0.5 mL 0.1 M phosphate buffer saline (PBS) buffer containing 0.1 M NaCl and 3 mM MPA at 37 °C for 2 h. This was then followed by dipping the electrode in a solution containing the for TROJAN probe (C = 1.0 μM) and the EDC/NHS mixture at 37 °C for 30 min. Subsequently, each electrode was rinsed thoroughly with PBS buffer and deionized water to rid the surface of unlinked TROJAN probe molecules. To detect TROJAN, each electrode was exposed to different concentrations of TROJAN at 37 °C for 2 h, and the surface was rinsed with PBS and deionized water. The sequence of the TROJAN probe for TROJAN is 5′-NH2-ACA AGT GTG AGT TGA CTG GAA GTC TGA GAG GGA AAG TCC CCT CTA GAG TA-3′.
2.5. PEC measurements
All electrochemical measurements were carried out on a CHI850D electrochemical workstation (ChenHua Instruments Co., Ltd, Shanghai, China). The photoelectrochemical measurements were conducted in a three-electrode cell. An Ag/AgCl electrode (saturated KCl) and a Pt wire electrode served as the reference and auxiliary electrodes, respectively. A PBS buffer comprising 0.1 M ascorbic acid (AA) as the hole scavenger was found to be the best, because in it a high photocurrent can be generated (panel A in Fig. S1†). Glucose (0.05 M) was also added to maintain a steady flow of photocurrent (panels B–D in Fig. S1†).
The light source was a CEL-LED 100-WL white LED (Zhongjiao Jinyuan Technology Co., Ltd, Beijing, China). The irradiation wavelength was set at 430 nm with an exposure time of 25 s and a bias voltage of −0.1 V.
Total RNA was isolated from MCF7 cells using a RNeasy Plus Mini Kit (QIAGEN) following the manufacturer's protocol.
3 Results and discussion
3.1. Sensor design
Scheme 1 shows the construction of our PEC sensor. Briefly, Cu2O is electrodeposited on an ITO substrate, with the follow-up in situ electropolymerization of TTh. As demonstrated by the data below, the electropolymerized pTTh film forms a type II heterojunction with Cu2O for efficient electron delocalization. Prior to sample analyses, MPA molecules are allowed to adsorb for the follow-up probe immobilization (Fig. S2†). This is followed by crosslink of the probe molecules and the duplex formation with the TROJAN target. The number of DNA/RNA duplexes formed is dependent on the target concentration. As the TROJAN concentration increases, the photocurrent signal continually decreases from the baseline current (J1), because the higher the density of the DNA/RNA duplexes, the greater extent of the blockage of the receiving of photoholes by the redox species penetrated to the pores of pTTh from solution (a characteristic of an “off-sensor”). The excellent reproducibility described in the section below suggests that our sensor preparation is highly controllable. With the same potentiostat used for the PEC detection, our sensor fabrication is also cost-effective.
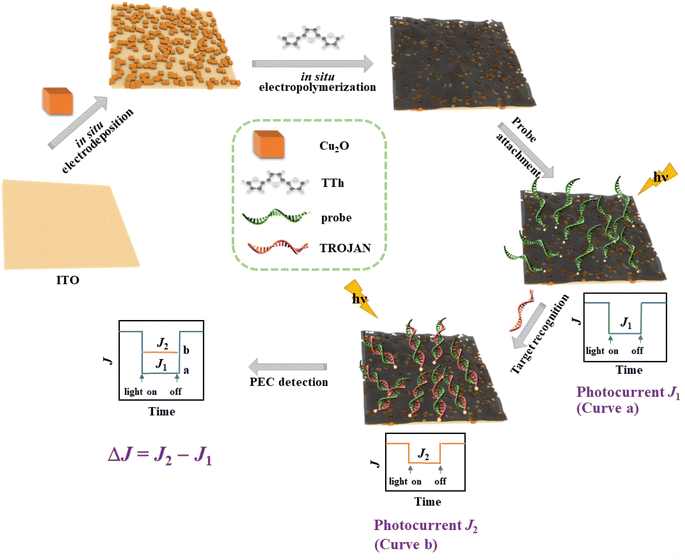 |
| Scheme 1 Schematic illustration of the PEC sensor construction and its use for detecting the exosomal TROJAN target. In the PEC measurement, curve a represents the baseline photocurrent at the probe-covered pTTh/Cu2O biosensor (J1), which is reduced to J2 when the probe molecule forms a duplex with the target (curve b). The difference, ΔJ = J2 − J1, is used to construct the calibration curve and sample quantification. The holes accumulated in Cu2O can oxidize an oxidizable solution species penetrated through the pores of pTTh, while the photoelectrons are separated into pTTh and transferred to the ITO substrate through the pTTh/ITO interface. | |
3.2. Characterizations of pTTh/Cu2O
The surface morphologies of the electrodeposited pTTh, Cu2O, and pTTh/Cu2O were initially examined by SEM. In Fig. 1A depicts some highly uniform Cu2O cubes, whose length is around 500 nm, while Fig. 1B shows that the pTTh film illustrates a pleated structure with a large specific surface area. Owing to this uniform and tight coverage, the diffusion length of the photogenerated carriers is shortened, allowing the photogenerated holes at pTTh to readily move to the underlying Cu2O tubes and be effectively separated from the photoelectrons.40
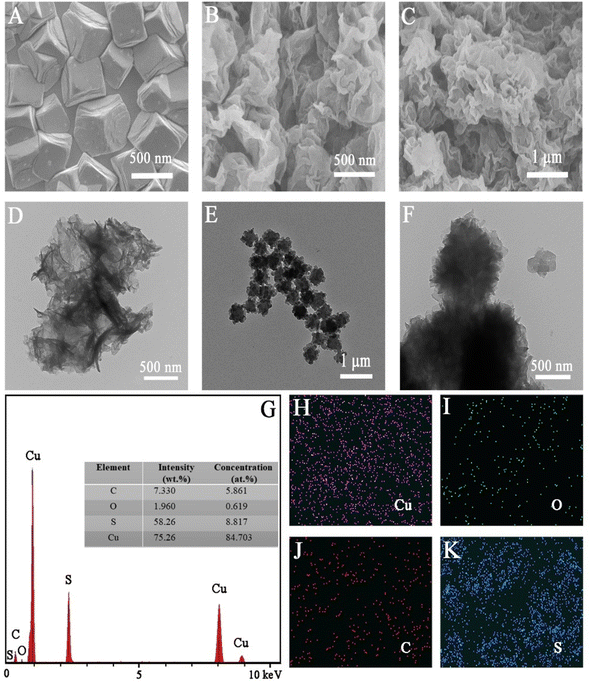 |
| Fig. 1 SEM images of (A) Cu2O, (B) pTTh, and (C) pTTh/Cu2O. The TEM images of (D) pTTh and (E) pTTh/Cu2O are shown. The TEM image of pTTh/Cu2O shown in (F) is in higher resolution. (G) EDS and elemental mapping images of (H) Cu, (I) O, (J) C and (K) S of pTTh/Cu2O. | |
The surface morphology and structure of pTTh/Cu2O were also examined with TEM and compared to those of the individual constituents. Fig. 1D confirms that pTTh is indeed a pleated, amorphous film. Fig. 1E and F indicate that the length of the Cu2O cube is consistent with the SEM result (Fig. 1B). The pTTh film completely enveloped the Cu2O cube as well as the gaps between the Cu2O tubes (see also Scheme 1). Fig. 1G reveals the coexistence of Cu, O, C, and S, while Fig. 1H–K suggest that these four elements are evenly distributed throughout the pTTh/Cu2O heterojunction.
The crystal structure and surface composition of the heterojunction materials were analyzed with XRD and XPS. The XRD pattern in Fig. 2A shows that Cu2O has the typical cuprite diffraction (JCPDS no. 05-0667) at 29.6°, 36.4°, 42.3°, 61.4°, and 73.5°, corresponding to the (110), (111), (200), (220) and (311) crystal planes.41 In Fig. 2A, the peaks in the XRD pattern are characteristic of diffuse scattering and can be attributed to the amorphous feature of the polymer.
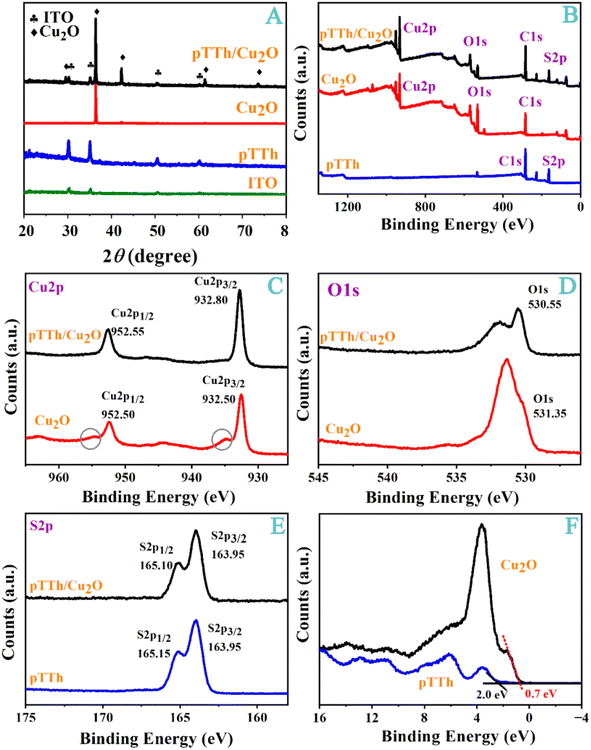 |
| Fig. 2 (A) XRD patterns, (B) a full-scan XPS spectrum, and the corresponding narrow scan XPS spectra of (C) Cu2p, (D) O1s, and (E) S2p. (F) Valence band edge spectra of pTTh and pTTh/Cu2O are shown. | |
In Fig. 2B, only the peak characteristic of Cu2p, O1s and C1s can be seen in the full-scan XPS spectra of Cu2O. The additional S2p peak in the pTTh/Cu2O heterojunction verifies the successful deposition of the pTTh film onto Cu2O. The Cu2p3/2 and Cu2p1/2 peaks of Cu2O can be found at 932.50 and 952.50 eV in Fig. 2C, respectively. Two small peaks, indicative of slight Cu2O oxidation,34 are present in the regions of 953–957 and 933–937 eV; however, they are absent in the XPS spectra of pTTh/Cu2O. Thus, the pTTh film is impermeable to oxygen in solution. Even though the pTTh film is amorphous and porous, its hydrophilicity prevents O2, which is more hydrophobic, from reaching the underlying Cu2O tubes. The electrochemically active surface area (ECSA) of this heterojunction was measured to be 1.81 cm2 (Fig. S3†), which is greater than the geometric area of the electrode (1.5 cm2). Therefore, the heterojunction formed on the substrate electrode also increased the reactive surface area.
As shown in Fig. 2D, the O1s peak is located at 531.35 eV with a broad peak, which is attributable to the hydroxyl groups (531.61 eV) adsorbed on the Cu2O surface.42 The O1s peak of pTTh/Cu2O shifts slightly to 530.55 eV after the pTTh formation, because pTTh reduces the O1s binding energy of the hydroxyl groups on the Cu2O surface. As shown in Fig. 2E, the peaks of S2p3/2 and S2p1/2 are located at 163.95 and 165.15 eV, consistent with the literature data.43 The S2p3/2 and S2p1/2 peaks are at 163.95 and 165.10 eV, respectively. The positions of the valence bands (VB) of Cu2O (0.7 eV) and pTTh (2.0 eV) were determined through linear extrapolation fitting of the XPS data presented in Fig. 2F. The results are consistent with those deduced from our Mott–Schottky measurements (Fig. S4†).
The optical properties of Cu2O, pTTh, and pTTh/Cu2O were further characterized with UV-vis spectroscopy (Fig. 3A). The optical absorption edge of pTTh is 620 nm. A bandgap energy of 1.9 eV for pTTh was deduced from normalization and Tauc fitting of the spectrum in Fig. 3B.44 For Cu2O, the optical absorption edge is 610 nm, which greatly attenuates in the range of 500–600 nm. Similarly, the bandgap of Cu2O was deduced from Fig. 3C to be 2.1 eV, in agreement with the literature value.45 The conduction bands (CB) are therefore around 0.1 and −1.4 eV for pTTh and Cu2O, respectively. As mentioned in the Introduction, such a staggered band structure favors the separation of photoelectrons. Apparently, a sensitization effect exists between the pTTh and Cu2O, which widens the range of visible light absorption.
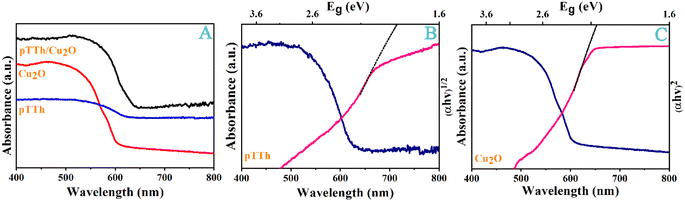 |
| Fig. 3 (A) UV-vis spectra for the heterojunction and its constituents. The Tauc plot (red) calculated from the absorption spectrum (blue) of (B) pTTh and (C) Cu2O are also shown. | |
3.3. Performance and the photovoltaic mechanism of the PEC sensor
As shown in Fig. 4A, pTTh and Cu2O both have lower photoelectric activities when compared to that of pTTh/Cu2O. The photocurrent density of pTTh is about −80 μA cm−2, and that of Cu2O is about −90 μA cm−2. By contrast, the photocurrent density of the pTTh/Cu2O was augmented to −250 μA cm−2. This increase can be again ascribed to the staggered band structure that inhibits the hole/electron recombination.46 We should add that the resonance structure of pTTh helps delocalize the photogenerated electrons (the electron/hole lifetime of Cu2O has been reported to be only 1 ps (ref. 47)), further limiting the hole/electron recombination. This effect of the heterojunction on prolonging the photoelectron lifetime is reflected from the Nyquist plots shown in Fig. 4B and the equivalent circuit depicted in Fig. 4C. The values of Ret (charge transfer resistance) are 2.6 Ω for pTTh, 10.6 Ω for Cu2O and 51.5 Ω for pTTh/Cu2O. Concurrently, Cdl (double-layer capacitance) also increases in the same order (see also values summarized in Table S1†). Linear sweep voltammetry (LSV, Fig. S5†) and incident photon-to-current conversion efficiency (IPCE, Fig. S6†) measurements directly demonstrate that the formation of this heterojunction enhances the photoactivity and accelerates the separation of the photogenerated electron–hole pairs. The IPCE values range from 13 to 32% within 360–700 nm and are higher in the visible region than some other reports.27
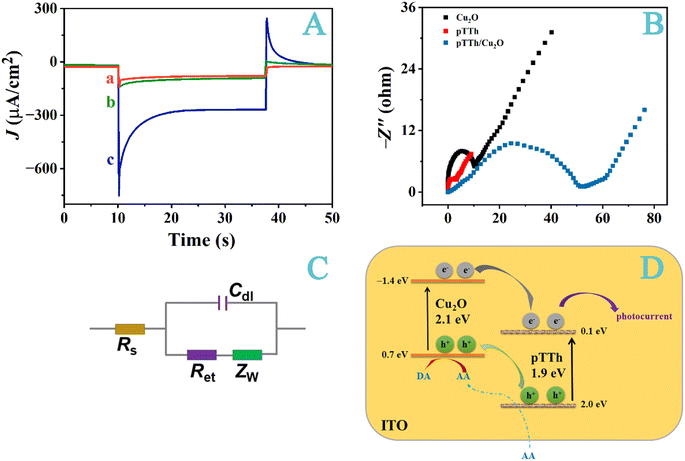 |
| Fig. 4 Signal responses at (a) pTTh, (b) Cu2O, and (c) pTTh/Cu2O (A), Nyquist plots of pTTh, Cu2O, and pTTh/Cu2O (B), as well as the Randles equivalent circuit (C), are presented. Additionally, (D) a schematic depiction of the mechanism behind the photocurrent generation is shown, including the band energy values and bandgap. AA added in the sample solution penetrate through the pores of pTTh (denoted by the red arrows) to the Cu2O tubes and are oxidized to deoxyascorbic acid (DA) by the photogenerated holes. | |
Fig. 4D depicts the mechanism behind the photocurrent generation. In a type II heterojunction, upon light irradiation, the electrons are accumulated in the CB of pTTh44 while the photogenerated holes are concentrated in the VB of Cu2O.45 The photogenerated holes will oxidize an easily oxidizable species in the solution. It is likely that AA, being hydrophilic, should be able to diffuse through the pTTh pores to reach the Cu2O tubes. Concurrently, electrons accumulated in the pTTh flow through the area in contact with the substrate electrode to produce the photocurrent (see also Scheme 1).27 Thus, the heterojunction separates the holes from their electron counterparts into different materials and the spatial separation impedes the recombination.33,39,48–50 Notice that the redox capability is compromised with a heterojunction. However, this is not an issue in the PEC detection because an additive more readily oxidizable than AA can be used if the oxidation potential is lowered.
We assessed the feasibility of our method for quantifying lncRNA TROJAN (Fig. 5). The performance of this sensor was first evaluated after the functionalization of this sensor (Fig. S7†). As depicted in Fig. 5A, the photocurrent decreases as the TROJAN concentration is below 1.0 nM and begins to plateau thereafter, after most of the anchored probe molecules have formed duplexes with the target molecules. This behavior is typical of a heterogeneous sensor whereon the amount of analyte adsorption is directly proportional to the concentration when the surface coverage is low. When the adsorption amount is high, more incident light is blocked and less AA molecules can move closer to the Cu2O tubes to annihilate the photoholes. These two factors jointly decrease the amount of photoelectrons or photocurrent.
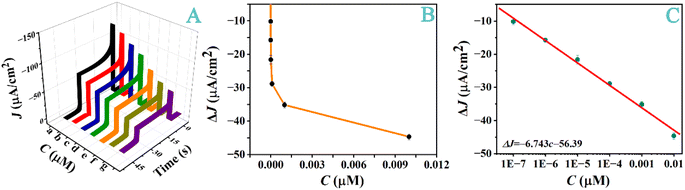 |
| Fig. 5 (A) Photocurrent responses of the PEC sensor to different concentrations of TROJAN (a: blank and baseline current and b to g: 10 nM to 0.1 pM and their corresponding currents). (B) ΔJ plotted against concentration. (C) ΔJ plotted as a function of log C. The error bars are relative standard deviations (RSDs) of three replicates. | |
Fig. 5B plots the decreases of the photocurrents with respect to the baseline value of the blank solution (ΔJ) against the TROJAN concentration, while Fig. 5C shows that ΔJ is proportional to the logarithm of the TROJAN concentration, log
C, within the concentration range of 0.1–10
000 pM. The linear regression equation is ΔJ = −6.743
log
C − 56.39, with a linear correlation coefficient of R2 = 0.9934. Using the lower portion of the curve in Fig. 5B and a signal-to-noise ratio that is higher than three folds of the noise level (S/N ≥ 3), we gauged the detection limit of our PEC sensor to be 15.8 fM. Such a level compares well with those attainable with many previously reported methods (Table S2†). We should note that some recent work, based on polarity-switching PEC sensors for lncRNA detections, have achieved sensitivity levels down to low fM or to even aM levels.12,13 Although the polarity-switching strategy involves complicated sensor fabrication and detection procedures, superb sensitivity can be attained because of the much enhanced signal-to-noise ratio. Using the diameter of a typical breast cancer cell (about 10 μm (ref. 51)), one copy of lncRNA corresponds to about 3 pM in the interior of such a cancer cell. Because the expression level of TROJAN is in the hundreds, our sensor is more than adequate for measuring the low abundance of this cancer biomarker.22 Indeed, we found that our method can easily measure the amount of TROJAN lncRNAs in MCF7 cells (vide infra).
To assess the feasibility of our sensor for real-sample analysis, we evaluated its stability, selectivity, and reproducibility. As shown in Fig. 6A, the signals were continuously measured for 800 s in PBS with 24 irradiation on/off cycles. It is evident that the photocurrent response is highly repeatable, with an RSD value of only 2.09%. We also stored electrodes covered with dry pTTh/Cu2O at room temperature in dark for three months and measured signals for the same sample intermittently. As shown by the representative data in Fig. 6B, the storage life is as long as three months. We opted to store the heterojunction-covered electrodes instead of the probe-covered sensors because MPA used to anchor the probe molecules is prone to oxidation.52 The stability and reproducibility exhibited in Fig. 6B indicate that it is a viable approach as the MPA adsorption and probe immobilization are apparently repeatable under the same experimental condition. To assess the selectivity, we measured a buffer solution containing 1.0 nM TROJAN and compared the signal to those from solutions with the ANCR, BCRT1, UCA1, L1 gene fragments and their mixture as additives. ANCR53 and BCRT1 (ref. 54) are lncRNAs linked to the etiology of the triple-negative breast cancer, while UCA1 (ref. 55) and L1 (ref. 56) are gene markers of colon cancer. As shown in Fig. 6C, our sensor did not show a significant attenuation of photocurrent in the presence of these fragments, suggesting that the additives did not adsorb nonspecifically onto the sensor surface. In fact, the recovery rate of the sample with these lncRNAs is 102 ± 2%. To further demonstrate the suitability of our sensor for real sample analyses, we measured lncRNA TROJAN extracted from MCF7 tumor cells, and found that the lncRNA TROJAN was at 480 pM corresponding to the literature,22 with a RSD value of 1.45%.
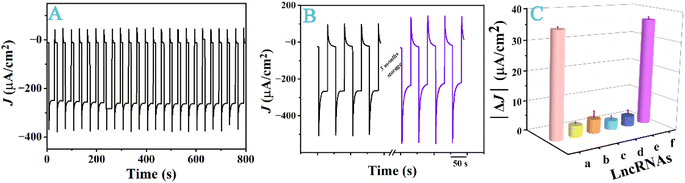 |
| Fig. 6 (A) Repeatability of the PEC sensor, (B) storage life of the heterojunction in dry state, and (C) selectivity of the PEC sensor for detecting TROJAN (1 nM) in (a) a buffer and (b) buffer solutions containing L1 (1 nM), (c) ANCR (1 nM), (d) UCA1 (1 nM), (e) BCRT1 (1 nM), and the mixture of these additives (f, 1 nM each). | |
The aforementioned features, along with the fact that small ITO substrates can be mass-produced, render the possibility of fabricating our PEC sensors as of the disposable kind. Finally, we gauged the sensor-to-sensor reproducibility by employing 25 sensors to measure the same sample. The resulting RSD value, 2.35%, is exceedingly low. This again indicates that the in situ electrodepositions, as well as the follow-up MPA adsorption and probe immobilization are controllable in producing PEC sensors of consistent composition and structure.
4 Conclusion
We have developed a sensitive PEC sensor for reproducible and reliable lncRNA detection. Sequential electrodepositions of Cu2O and pTTh produced a type II heterojunction structure at an ITO substrate. Owing to the uniform coverage and tight contact of pTTh, the heterojunction is not only highly stable (preventing the underlying Cu2O from oxidation), but also effective in accelerating the photoelectron transfer and enhancing the photocurrent. Furthermore, the use of pTTh facilitates the affixation of oligonucleotide probe molecules. We demonstrate that the judicious choice of inorganic and polymeric materials, in conjunction with simple yet reproducible surface preparative methods, can lead to a wide linear range with a remarkably low detection limit. These are prerequisites for robust detections of biomarkers at ultratrace levels in clinical settings. Thus, our method can be easily extended to the immobilization of other types of biomolecules such as proteins and peptides, significantly expanding the range of biological applications of PEC biosensors based on the inorganic/conductive heterojunctions.
Data availability
The data for this article have been included in the manuscript and also as part of the ESI.†
Author contributions
Xuehui Pang, Xiaoyi Lv, Yingxin Pang, Zhen Li, Qing Kang, Rui Liu, and Jiandong Xie performed experiments. Wenjun Lu, Lebin Sun, Qiuyan Wang. Rui Liu, and Jiandong Xie conducted some data analysis. Pang and Feimeng Zhou conceptualized the work and wrote the manuscript.
Conflicts of interest
The authors declare that they have no known competing financial interests or personal relationships that could have appeared to influence the work reported in this paper.
Acknowledgements
This work was supported by The Program of Science and Technology Plan of the City of Tianjin (No. 24JRRCRC00040), the National Natural Science Foundation of China (No. 21904081 and No. 81902657), and a grant from the start-up funds provided by Tiangong University.
References
- D. Nandi, S. Parida and D. Sharma, Gut Microbes, 2023, 15, 2221452 CrossRef PubMed.
- T. Sorlie, C. M. Perou, R. Tibshirani, T. Aas, S. Geisler, H. Johnsen, T. Hastie, M. B. Eisen, M. van de Rijn, S. S. Jeffrey, T. Thorsen, H. Quist, J. C. Matese, P. O. Brown, D. Botstein, P. E. Lonning and A. L. Borresen-Dale, Proc. Natl. Acad. Sci. U. S. A., 2001, 98, 10869–10874 CrossRef CAS.
- J. Lengfeld, H. T. Zhang, S. Stoesz, R. Murali, F. Pass, M. I. Greene, P. N. Goel and P. Grover, Breast Cancer, 2021, 13, 575–593 CAS.
- H. You, M. Wang, S. Wang, J. Xu, S. H. Hu, T. H. Li, Z. Z. Yu, D. P. Tang and N. Gan, Anal. Chem., 2023, 95, 11211–11218 CrossRef CAS.
- Z. Wang, L. Balembois, M. Rančić, E. Billaud, M. Le Dantec, A. Ferrier, P. Goldner, S. Bertaina, T. Chanelière, D. Esteve, D. Vion, P. Bertet and E. Flurin, Nature, 2023, 619, 276–281 CrossRef CAS.
- X. Bi, D. M. Czajkowsky, Z. Shao and J. Ye, Nature, 2024, 628, 771–775 CrossRef CAS PubMed.
- R. Ni, K. Ge, Y. Luo, T. Zhu, Z. Hu, M. Li, P. Tao, J. Chi, G. Li, H. Yuan, Q. Pang, W. Gao, P. Zhang and Y. Zhu, Biosens. Bioelectron., 2024, 264, 116616 CrossRef CAS PubMed.
- N. Debnath, L. S. Live and M. Poudineh, Lab Chip, 2023, 23, 572–579 RSC.
- H. Liu, Y. Wang, S. Huang, J. Tai, X. Wang, X. Dai, C. Qiu, D. Gu, W. Yuan, H.-P. Ho, J. Chen and Y. Shao, Anal. Chem., 2024, 96, 8791–8799 CrossRef CAS.
- L. Cui, J. Shen, C.-C. Li, P.-P. Cui, X. Luo, X. Wang and C.-Y. Zhang, Anal. Chem., 2021, 93, 10310–10316 CrossRef CAS PubMed.
- X. H. Pang, X. Zhang, K. K. Gao, S. Wan, C. Cui, L. Li, H. B. Si, B. Tang and W. H. Tan, ACS Nano, 2019, 13, 1817–1827 CAS.
- R. Yang, J. Ji, L. Ding, X. Yuan, L. Qu, Y. Wu and Y. Li, Anal. Chem., 2024, 96, 13278–13284 CrossRef CAS.
- Y. Li, L.-e. Liu, H. Han, X. Yuan, J. Ji, L. Xue, Y. Wu and R. Yang, Talanta, 2024, 273, 125878 CrossRef CAS.
- M. Zuo, Y. Yang, S. Jiang, C. Zhu, Y. Han, J. Hu, K. Ren, L. Cui and C.-Y. Zhang, Food Chem., 2024, 445, 138716 CrossRef CAS PubMed.
- J. Yin, Z. Zou, F. Yin, H. Liang, Z. Hu, W. Fang, S. Lv, T. Zhang, B. Wang and Y. Mu, ACS Nano, 2020, 14, 10385–10393 CrossRef CAS PubMed.
- Y. Zhang, C. Wang, X. Zou, X. Tian, J. Hu and C.-y. Zhang, Nano Lett., 2021, 21, 4193–4201 CrossRef CAS.
- W.-j. Liu, L. Zhang and C.-y. Zhang, Anal. Chem., 2023, 95, 16343–16351 CrossRef CAS.
- S. Jiang, T. Liu, Q. Liu, Q. Zhang, Y. Han, X. Tian and C.-y. Zhang, Anal. Chem., 2023, 95, 15133–15139 CrossRef CAS PubMed.
- C. Jiang, X. Li, H. Zhao and H. Liu, Mol. Cancer, 2016, 15, 62 CrossRef.
- X. Jin, L. Ge, D. Li, Z. Shao, G. Di, X. Xu and Y.-Z. Jiang, Mol. Cancer, 2020, 19, 87 CrossRef CAS.
- K. K. Thakur, A. Kumar, K. Banik, E. Verma, E. Khatoon, C. Harsha, G. Sethi, S. C. Gupta and A. B. Kunnumakkara, J. Cell. Physiol., 2021, 236, 7938–7965 CrossRef CAS.
- X. Jin, X. Xu, Y. Jiang, Y. Liu, W. Sun, Y. Guo, Y. Ren, W. Zuo, X. Hu, S. Huang, H. Shen, F. Lan, Y. He, G. Hu, G. Di, X. He, D. Li, S. Liu, K. Yu and Z. Shao, Sci. Adv., 2019, 5, eaat9820 CrossRef CAS.
- X. H. Pang, C. Cui, M. H. Su, Y. G. Wang, Q. Wei and W. H. Tan, Nano Energy, 2018, 46, 101–109 CrossRef CAS PubMed.
- J. X. Low, J. G. Yu, M. Jaroniec, S. Wageh and A. A. Al-Ghamdi, Adv. Mater., 2017, 29, 1601694 CrossRef.
- F. H. Chu, X. L. Qu, Y. C. He, W. L. Li, X. Q. Chen, Z. L. Zheng, M. Yang, X. N. Ru, F. G. Peng, M. H. Qu, K. Zheng, X. X. Xu, H. Yan and Y. Z. Zhang, Nat. Commun., 2023, 14, 3596 CrossRef CAS PubMed.
- X. Y. Huang, L. M. Zhang, L. Tong, Z. Li, Z. R. Peng, R. F. Lin, W. H. Shi, K. H. Xue, H. W. Dai, H. Cheng, D. D. Branco, J. B. Xu, J. B. Han, G. J. Cheng, X. S. Miao and L. Ye, Nat. Commun., 2023, 14, 2190 CrossRef CAS.
- Y. Wang, H. Shi, K. Cui and J. Yu, Appl. Catal., B, 2019, 250, 171–180 CrossRef CAS.
- J. L. Fan, X. F. Hu, W. W. Qin, Z. Y. Liu, Y. S. Liu, S. J. Gao, L. P. Tan, J. L. Yang, L. B. Luo and W. Zhang, Nanoscale, 2022, 14, 13204–13213 RSC.
- Y. M. Kou, L. D. Chen, J. L. Mu, H. Miao, Y. S. Wang, X. Y. Hu and F. Teng, Nanotechnology, 2020, 31, 195601 CrossRef CAS PubMed.
- X. H. Zhou, R. Liu, K. Sun, K. M. Papadantonakis, B. S. Brunschwig and N. S. Lewis, Energy Environ. Sci., 2016, 9, 892–897 RSC.
- X. Deng, R. Li, S. Wu, L. Wang, J. Hu, J. Ma, W. Jiang, N. Zhang, X. Zheng, C. Gao, L. Wang, Q. Zhang, J. Zhu and Y. Xiong, J. Am. Chem. Soc., 2019, 141, 10924–10929 CrossRef CAS PubMed.
- D. Barreca, P. Fornasiero, A. Gasparotto, V. Gombac, C. Maccato, T. Montini and E. Tondello, ChemSusChem, 2009, 2, 230–233 CrossRef CAS.
- X. Chang, T. Wang, P. Zhang, Y. Wei, J. Zhao and J. Gong, Angew. Chem., Int. Ed., 2016, 55, 8840–8845 CrossRef CAS PubMed.
- W. Niu, T. Moehl, P. Adams, X. Zhang, R. Lefèvre, A. M. Cruz, P. Zeng, K. Kunze, W. Yang and S. D. Tilley, Energy Environ. Sci., 2022, 15, 2002–2010 RSC.
- H. Ullah, R. Ahmad, A. A. Khan, N. E. Lee, J. Lee, A. U. Shah, M. Khan, T. Ali, G. Ali, Q. Khan and S. O. Cho, ACS Omega, 2022, 7, 42377–42395 CrossRef CAS.
- Y. Zhao, L. Fan, Y. Zhang, H. Zhao, X. Li, Y. Li, L. Wen, Z. Yan and Z. Huo, ACS Appl. Mater. Interfaces, 2015, 7, 16802–16812 CrossRef CAS.
- K. Dashtian, S. Hajati and M. Ghaedi, Anal. Chem., 2022, 94, 6781–6790 CrossRef CAS PubMed.
- K. Dashtian, S. Hajati and M. Ghaedi, Biosens. Bioelectron., 2020, 165, 112346 CrossRef CAS.
- C. Yang, P. D. Tran, P. P. Boix, P. S. Bassi, N. Yantara, L. H. Wong and J. Barber, Nanoscale, 2014, 6, 6506–6510 RSC.
- S. W. Lee, Y. S. Lee, J. Heo, S. C. Siah, D. Chua, R. E. Brandt, S. B. Kim, J. P. Mailoa, T. Buonassisi and R. G. Gordon, Adv. Energy Mater., 2014, 4, 1301916 CrossRef.
- Y. Li, X. Zhong, K. Luo and Z. Shao, J. Mater. Chem. A, 2019, 7, 15593–15598 RSC.
- H. Li, Z. Su, S. Hu and Y. Yan, Appl. Catal., B, 2017, 207, 134–142 CrossRef CAS.
- K. Wang, Z. Mo, S. Tang, M. Li, H. Yang, B. Long, Y. Wang, S. Song and Y. Tong, J. Mater. Chem. A, 2019, 7, 14129–14135 RSC.
- W. Fan, B. Zhang, X. Wang, W. Ma, D. Li, Z. Wang, M. Dupuis, J. Shi, S. Liao and C. Li, Energy Environ. Sci., 2020, 13, 238–245 RSC.
- A. Paracchino, N. Mathews, T. Hisatomi, M. Stefik, S. D. Tilley and M. Grätzel, Energy Environ. Sci., 2012, 5, 8673–8681 RSC.
- W. Zhao, Y. Feng, H. Huang, P. Zhou, J. Li, L. Zhang, B. Dai, J. Xu, F. Zhu, N. Sheng and D. Y. C. Leung, Appl. Catal., B, 2019, 245, 448–458 CrossRef CAS.
- M. A. Mahmoud, W. Qian and M. A. El-Sayed, Nano Lett., 2011, 11, 3285–3289 CrossRef CAS.
- W. Fan, C. Chen, H. Bai, B. Luo, H. Shen and W. Shi, Appl. Catal., B, 2016, 195, 9–15 CrossRef CAS.
- C. Lin, Y. Lai, D. Mersch and E. Reisner, Chem. Sci., 2012, 3, 3482–3487 RSC.
- J. Bai, Y. Li, R. Wang, K. Huang, Q. Zeng, J. Li and B. Zhou, J. Mater. Chem. A, 2015, 3, 22996–23002 RSC.
- K. Kyriakopoulou, E. Riti, Z. Piperigkou, K. K. Sarri, H. Bassiony, M. Franchi and N. K. Karamanos, Cells, 2020, 9, 2256 CrossRef CAS PubMed.
- Q. Shen, L. Han, G. Fan, J.-R. Zhang, L. Jiang and J.-J. Zhu, Anal. Chem., 2015, 87, 4949–4956 CrossRef CAS.
- S. B. Wang, F. Lan and Y. Xia, Med. Sci. Monit., 2018, 24, 6002–6009 CrossRef CAS PubMed.
- G. Han, Q. Y. Guo, N. Ma, W. Z. Bi, M. Xu, J. P. Jia and W. Wang, Aging, 2021, 13, 15501–15510 CrossRef CAS PubMed.
- M. H. Wang, Z. J. Zhang, D. Pan, Z. G. Xin, F. Bu, Y. Zhang, Q. W. Tian and X. D. Feng, Biosci. Rep., 2021, 41, BSR20211115 CrossRef CAS PubMed.
- https://www.ncbi.nlm.nih.gov/nuccore/XR_927227.2/.
|
This journal is © The Royal Society of Chemistry 2024 |
Click here to see how this site uses Cookies. View our privacy policy here.