DOI:
10.1039/D4RA05269B
(Review Article)
RSC Adv., 2024,
14, 33143-33190
Sustainable polymeric adsorbents for adsorption-based water remediation and pathogen deactivation: a review
Received
2nd August 2024
, Accepted 17th September 2024
First published on 21st October 2024
Abstract
Water is a fundamental resource, yet various contaminants increasingly threaten its quality, necessitating effective remediation strategies. Sustainable polymeric adsorbents have emerged as promising materials in adsorption-based water remediation technologies, particularly for the removal of contaminants and deactivation of water-borne pathogens. Pathogenetic water contamination, which involves the presence of harmful bacteria, viruses, and other microorganisms, poses a significant threat to public health. This review aims to analyze the unique properties of various polymeric materials, including porous aromatic frameworks, biopolymers, and molecularly imprinted polymers, and their effectiveness in water remediation applications. Key findings reveal that these adsorbents demonstrate high surface areas, tunable surface chemistries, and mechanical stability, which enhance their performance in removing contaminants such as heavy metals, organic pollutants, and emerging contaminants from water sources. Furthermore, the review identifies gaps in current research and suggests future directions, including developing multifunctional polymeric materials and integrating adsorption techniques with advanced remediation technologies. This comprehensive analysis aims to contribute to advancing next-generation water purification technologies, ensuring access to clean and safe water for future generations.
1. Introduction
Water is an essential resource for all forms of life, playing a critical role in public health, economic development, and ecological balance.1 The increasing demand for clean water is driven by population growth, industrialization, and agricultural expansion, leading to significant pressure on water resources.2 Unfortunately, the quality of these resources is increasingly compromised by various contaminants, including industrial effluents, agricultural runoff, and domestic waste.3 These pollutants threaten human health and the environment, necessitating effective water remediation strategies.4 Contaminants in water can be broadly classified into several categories: physical, chemical, biological, and radiological. Physical contaminants, such as suspended solids and sediments, can impair water quality and aesthetic appeal.5 They are generally easier to remove through conventional methods such as sedimentation and filtration. Chemical contaminants include many organic and inorganic substances, such as heavy metals, pesticides, pharmaceuticals, and nutrients.6 These contaminants often require specific remediation techniques tailored to their unique chemical properties, making their removal more challenging.7
Biological contaminants consist of microorganisms, including bacteria, viruses, and protozoa, which can lead to waterborne diseases.8 Traditional disinfection methods, such as chlorination and UV irradiation, are commonly employed to inactivate these pathogens, but they must be carefully managed to avoid the formation of harmful byproducts.9 Radiological contaminants like radon and uranium may arise from natural geological formations or human activities like mining and nuclear power generation.10 Removing these contaminants requires specialized techniques, including ion exchange and advanced oxidation processes.11 As the complexity of water contaminants continues to evolve, traditional remediation methods often fall short. Conventional techniques such as coagulation–flocculation, sedimentation, and filtration may not effectively address emerging contaminants like endocrine-disrupting chemicals and microplastics. These challenges highlight the need for advanced remediation technologies to remove a broader spectrum of pollutants.12
Among the various approaches to water remediation, adsorption has gained significant attention due to its versatility and efficiency.13 Adsorption involves the accumulation of substances (adsorbates) from a liquid or gas phase onto the surface of a solid material (adsorbent). The effectiveness of this process is influenced by the properties of the adsorbent, including its surface area, pore size distribution, and surface chemistry.14
Polymeric materials have emerged as particularly promising adsorbents for water remediation applications. Their unique properties, such as tunable surface chemistry, high mechanical strength, and diverse structural forms, enable them to be engineered for specific adsorption tasks.15 For instance, polymeric adsorbents can be synthesized or modified to incorporate a variety of hydrophilic groups, enhancing their affinity for targeted contaminants.16 This ability to tailor surface chemistry provides significant advantages over traditional adsorbents like activated carbon and natural clays.17
One of the key strengths of polymeric adsorbents is their high surface area and controlled pore size distribution, which are crucial for efficient contaminant binding.18 Recent research has highlighted significant findings in environmental pollution and advanced material applications.19–21 It is complemented by exploring innovative materials for environmental application, demonstrating the efficacy of polymer.22–25 The large surface area provides ample active sites for adsorption, while the porosity facilitates rapid diffusion and access to these sites. Polymeric materials exhibit excellent mechanical stability and chemical resistance, allowing them to withstand harsh water remediation environments without significant degradation.26 Various polymeric adsorbents have been developed and studied for their adsorption capabilities.27 Notable examples include polyaniline (PANI), known for its high capacity to adsorb heavy metals and organic pollutants, and chitosan, a biopolymer derived from chitin that demonstrates strong binding sites for various contaminants. Polyacrylamide (PAM) and its derivatives are commonly used as flocculants but also serve as effective adsorbents for dyes and heavy ions.28 Poly(vinyl alcohol) (PVA)-based hydrogels are particularly effective for adsorbing organic pollutants due to their high water content and swelling capacity.29
The versatility of polymeric adsorbents extends to their structural forms, which can include fibers, beads, membranes, and gels.30 This adaptability allows for their application in various water remediation scenarios, from centralized treatment facilities to decentralized systems such as point-of-use water filters.31 The scalability of polymeric materials also makes them attractive for large-scale applications, where cost and efficiency are critical considerations. Despite their advantages, the development of polymeric adsorbents is an evolving field with ongoing research aimed at enhancing their performance and sustainability.32 Future research directions include the integration of polymeric adsorbents with other remediation technologies, such as membrane filtration and advanced oxidation processes. This synergistic approach could improve overall remediation efficiency and address complex water quality challenges more effectively. Moreover, there is significant potential for the development of multifunctional polymeric materials that combine adsorption with additional capabilities, such as catalytic activity or antimicrobial properties.33 These innovations could lead to the creation of next-generation adsorbents that are effective in removing contaminants and contribute to the broader goals of environmental sustainability and resource conservation.34 In adsorption-based water remediation technologies, the ability of polymeric adsorbents to deactivate pathogens plays a critical role in ensuring the safety of treated water. By integrating antimicrobial agents such as metal nanoparticles or using polymers with inherent antibacterial properties, researchers have developed adsorbents that not only capture contaminants but also neutralize harmful microorganisms. This approach significantly enhances the overall efficacy of water purification processes, contributing to safer and more sustainable water management practices.
In conclusion, the pressing need for adequate water remediation technologies has highlighted the potential of polymeric adsorbents as versatile and efficient solutions for removing a wide range of contaminants from water.35 Their unique properties, tunable surface chemistries, and adaptability make them valuable tools for clean and safe water.36 As research advances in this field, polymeric adsorbents are poised to play a significant role in sustainable water purification technologies, ensuring the protection and preservation of vital water resources for future generations.37
2. Fundamentals of adsorption
Adsorption is a broadly used physical process in water remediation and purification, offering an efficient and versatile approach to removing a diverse range of contaminants.38 The fundamental principles of adsorption underpin its effectiveness and guide the development of advanced adsorbent materials for various applications.39 Adsorption is the adhesion of atoms, ions, or molecules from a gas, liquid, or dissolved solid to a surface. It differs from absorption, where the entire volume of another material takes up a substance. In water remediation, adsorption occurs when contaminants are attracted to and accumulate on the surface of a solid adsorbent material.40 The adsorbent material, which may be a porous solid like activated carbon, zeolites, or polymeric resins, offers a large surface area for adsorption. As the water flows through the adsorbent bed, the contaminants are selectively removed and retained on the adsorbent's surface. The efficiency and effectiveness of the adsorption process are influenced via several key factors: Surface area: a high surface area offers more sites for adsorption, upgrading the adsorbent's capacity.41 Pore size distribution: adsorbents with various pore sizes may accommodate different-sized contaminant molecules. Surface chemistry: the chemical nature of the adsorbent's surface, like the presence of hydrophilic groups, affects its affinity for specific contaminants.42 Molecular size and structure: smaller molecules and those with specific hydrophilic groups are more readily adsorbed.
Solubility: less soluble contaminants are generally more easily adsorbed. Charge: electrostatic interactions among the adsorbent and contaminant may upgrade adsorption. pH: the pH of the solution may affect the charge and speciation of both the adsorbent and the contaminant, influencing the adsorption process. Temperature: adsorption is generally an exothermic process, so higher temperatures may decrease the adsorption capacity.43 Ionic strength: increased ionic strength may affect the solubility and speciation of contaminants, as well as the surface charge of the adsorbent.44 Contact time: longer contact times allow more time for the adsorption equilibrium to be reached.45 Mixing: efficient mixing upgrades mass transfer and improves the contact among the adsorbent and contaminants.
Fig. 1 illustrates the three main stages of water remediation: water purification, wastewater remediation, and industrial water remediation. Water purification, represented via orange icons, involves removing contaminants from raw water to create safe drinking water through filtration and disinfection. Wastewater remediation, shown in green, is devoted to cleaning used water from various sources before releasing it into the environment, employing physical, biological, and chemical processes. Finally, industrial water remediation, depicted in purple, tailors water quality for specific industrial needs, which may consist of removing minerals or adjusting pH. The image uses a flow chart design with distinct icons and descriptions for each stage, highlighting the progression and specialization of water remediation processes from basic purification to industrial applications.
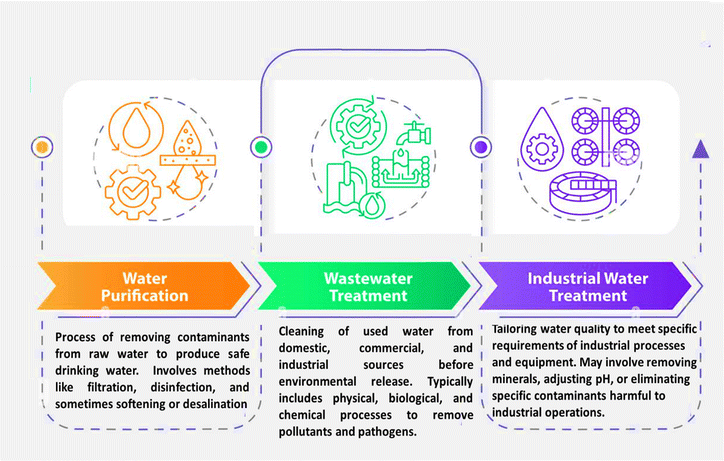 |
| Fig. 1 Illustrates the difference among water remediation, water purification, and industrial water remediation. | |
In Table 1, water remediation encompasses three primary categories: purification, wastewater remediation, and industrial water remediation, each addressing specific needs and challenges in water management. Water purification is devoted to producing potable water via eliminating biological, chemical, and physical contaminants through filtration, disinfection, and reverse osmosis.46 Wastewater remediation targets removing organic and inorganic pollutants and pathogens from domestic and industrial effluents using a combination of physical, biological, and chemical processes to protect environmental and public health. Industrial water remediation tailors quality to meet the specific requirements of industrial processes, often involving removing minerals, pH adjustment, and eliminating process-specific impurities. While these categories employ overlapping technologies, like advanced oxidation and membrane filtration, they differ significantly in their scale of operation, regulatory standards, and typical applications.47 Each category of water remediation faces unique challenges, including the emergence of contaminants like microplastics and pharmaceuticals. In response, the field of water remediation is constantly evolving, with advancements in technologies and adsorbents aimed at addressing these issues more effectively.
Table 1 Comprehensively compares water purification, wastewater, and industrial water remediation
Aspect |
Water purification |
Wastewater remediation |
Industrial water remediation |
Objective |
Offer clean and safe drinking water |
Remove contaminants to protect the environment and public health |
Remove impurities to prevent equipment damage and improve efficiency |
Primary contaminants |
Biological (bacteria, viruses), chemical (chlorine, lead, mercury), physical (sediment) |
Organic (BOD, COD), inorganic (heavy ions, nutrients), pathogens |
Specific to industrial processes (chemicals, heavy ions, organic compounds) |
Typical processes |
Filtration, disinfection (chlorination, UV), reverse osmosis |
Primary (screening, sedimentation), secondary (biological remediation), tertiary (advanced remediation) |
Filtration, ion exchange, reverse osmosis, chemical remediation |
Advanced technologies |
UV irradiation, advanced oxidation, nanofiltration |
Membrane bioreactors, advanced oxidation processes, nutrient removal |
Reverse osmosis, advanced oxidation processes, nanofiltration |
Scale of operation |
Municipal or household levels |
Municipal, industrial, or decentralized systems |
Industrial plants tailored to specific industry needs |
Regulatory standards |
EPA, WHO guidelines for drinking water quality |
EPA, local environmental protection standards |
Industry-specific standards, OSHA, EPA |
Disposal of viaproducts |
Minimal, generally safe for direct consumption |
Sludge remediation and disposal, safe discharge into water bodies |
Proper disposal or recycling of industrial by products |
Typical applications |
Drinking water supply for homes and communities |
Remediation of municipal and industrial sewage before discharge |
Water used in manufacturing, cooling, and other industrial processes |
Environmental impact |
Ensures safe potable water, reduces waterborne diseases |
Protects water bodies from pollution, reduces health hazards |
Reduces operational costs, protects the environment from industrial discharges |
Commonly used adsorbents |
Activated carbon, polymeric adsorbents |
Activated sludge, polymeric materials for specific contaminants |
Polymeric adsorbents, ion exchange resins |
Arising contaminants |
Microplastics, pharmaceuticals |
Pharmaceuticals, personal care products, microplastics |
Industry-specific contaminants like PFAS, VOCs |
Cost considerations |
Varies based on technology and scale, often subsidized via governments |
Infrastructure and operational costs, potential for government subsidies |
Capital and operational costs, efficiency savings |
Examples |
Municipal water plants, home water filters |
Municipal wastewater remediation plants, industrial effluent remediation plants |
Industrial cooling towers, boiler feed water remediation systems |
3. Adsorption mechanisms and principles
Adsorption is a fundamental process governed by various mechanisms and principles, crucial in fields ranging from environmental remediation to industrial separations.48 The mechanisms of adsorption primarily consist of physical (physisorption) and chemical (chemisorption) interactions among the adsorbate and adsorbent surfaces.49 Physisorption involves weak van der Waals forces like London dispersion, dipole–dipole interactions, and hydrogen bonding.50 These non-specific and reversible interactions occur at relatively low temperatures and high pressures.51 Conversely, chemisorption entails stronger, specific chemical bonds like covalent or ionic bonds forming among the adsorbate and adsorbent. It involves electron transfer and is typically irreversible without external energy input.52 Principles governing adsorption consist of ion exchange, where ions in the solution are swapped with similarly charged ions on the adsorbent's surface, driven via electrostatic forces. Surface complexation adsorption forms complexes among hydrophilic groups on the adsorbent surface and the adsorbate, influencing adsorption capacity and selectivity.
Additionally, adsorption principles like precipitation involve the formation of insoluble compounds that precipitate on the adsorbent surface, which is crucial in purification processes.53 Understanding these mechanisms and principles is essential for designing efficient adsorption processes tailored to specific water remediation, gas purification, catalysis, or pharmaceutical production applications. Each mechanism and principle offers unique advantages, influencing the efficacy and sustainability of adsorption-based technologies across diverse industries and environmental contexts.54
Fig. 2a represents the adsorption process for treating toxic metals in wastewater. This process involves the transfer of toxic material onto the surface of an adsorbent through physical or chemical means. The adsorbent, shown as a complex layered structure of brown and green shapes, represents the polymeric material with a large surface area and various functional groups, which are key characteristics for efficient adsorption. Fig. 2 demonstrates how the toxic materials (represented by red filled circles labeled as “Adsorbate”) are shifted from the solution onto the adsorbent surface. This process is driven by the interactions between the adsorbate and the adsorbent, which can be either physical (such as van der Waals forces, dispersion interactions, and hydrogen bonding) or chemical (involving covalent or electrostatic bonds). Fig. 2 also shows the dynamic nature of adsorption, with arrows indicating both adsorption (attachment to the surface) and desorption (detachment from the surface) processes. This illustrates the potential for regeneration and reuse of the adsorbents, which contributes to the method's environmental friendliness and cost-effectiveness. Fig. 2b shows a single layer of adsorbate molecules on the adsorbent surface, representing a simpler adsorption scenario. Fig. 2c depicts multiple layers of different molecules adsorbed onto the surface, illustrating the capacity of some adsorbents to accumulate multiple layers of adsorbate molecules.
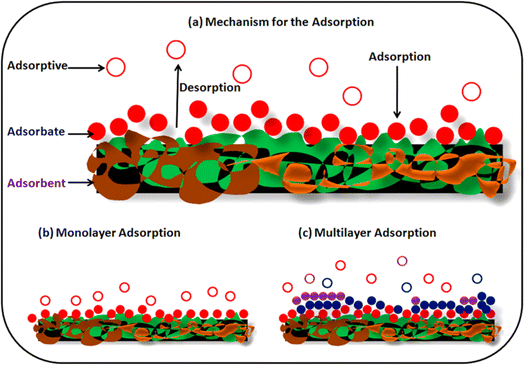 |
| Fig. 2 (a) General mechanism for the adsorption, (b) monolayer adsorption, and (c) multilayer adsorption. Reproduced from ref. 55 with permission from MDPI, copyright 2021. | |
Physisorption or physical adsorption is a complex process that occurs through various mechanisms and principles, each dependent on the adsorbent's and adsorbate's nature,56 is involves weak van der Waals forces among the adsorbate molecules and the surface of the adsorbent.57 These forces consist of dispersion forces, dipole–dipole interactions, and hydrogen bonding, which are non-specific and relatively weak. Physisorption is typically reversible and occurs at lower temperatures and higher pressures.58 Chemisorption or chemical adsorption is another fundamental adsorption mechanism where chemical bonds, like covalent or ionic bonds, form among the adsorbate and the adsorbent surface.59 This interaction is stronger and more specific than physical adsorption, often involving electron transfer among the adsorbate and the surface atoms of the adsorbent. Chemisorption processes are typically irreversible under normal conditions and require activation energy.60 In some cases, adsorption processes exhibit physical and chemical adsorption characteristics depending on environmental conditions like temperature, pressure, and the surface properties of the adsorbent.61 The dominant mechanism may shift between physisorption and chemisorption, impacting the adsorption capacity and selectivity.62 Ion exchange adsorption involves the exchange of ions among the adsorbent surface and ions in the solution.63 The mechanism is based on electrostatic interactions and the affinity of ions for charged sites on the adsorbent. It is broadly used in water softening, purification processes, and soil remediation to remove contaminants like heavy ions and radionuclides.64 Surface complexation adsorption occurs when specific hydrophilic groups on the adsorbent surface form complexes with the adsorbate molecules.65 This mechanism involves chemical interactions like ligand exchange, chelation, or coordination, depending on the adsorbent's surface chemistry and the adsorbate species' nature.66 Precipitation adsorption occurs when the adsorbate forms a solid residue on the surface of the adsorbent due to changes in solubility equilibrium.67 This mechanism is crucial in wastewater remediation and separation processes where insoluble compounds are formed and subsequently adsorbed onto the adsorbent surface.68 Biosorption utilizes biological materials like microorganisms, algae, or plant materials as adsorbents to remove pollutants or ions from aqueous solutions.69 The mechanism involves biochemical interactions among the biological material's adsorbate and surface hydrophilic groups.70 This includes ion exchange, complexation, and physical adsorption, making biosorption an eco-friendly and cost-effective method for environmental remediation.71 Hydrophobic adsorption occurs when hydrophobic molecules preferentially adsorb onto hydrophobic surfaces of adsorbents.72 This mechanism is driven via the tendency of non-polar molecules to minimize contact with water molecules, thereby adsorbing onto surfaces that reduce their exposure to the aqueous phase.73 It is broadly applied in areas like wastewater remediation and the recovery of organic compounds from aqueous solutions.74 Chiral adsorption involves the selective adsorption of enantiomers (mirror-image molecules) onto chiral surfaces or adsorbents.75 This mechanism exploits specific interactions among the chiral centers of the adsorbent and the chiral molecules, enabling the separation or purification of enantiomers in pharmaceuticals, fine chemicals, and food industries. Chiral adsorption is crucial in ensuring the purity and efficacy of chirally pure compounds.76 Electrostatic adsorption occurs when charged ions or molecules are attracted to oppositely charged surfaces or adsorbents.77 This mechanism relies on coulombic attraction among the charged species in the solution and the charged hydrophilic groups on the adsorbent surface. Electrostatic adsorption is utilized in applications like wastewater remediation, ion exchange chromatography, and separation processes where selective removal or recovery of charged species is required.78
Fig. 3 illustrates the complex adsorption process, showcasing various mechanisms by which ions and molecules interact with an adsorbent material. The adsorbent is depicted as a large gray semicircle with a porous structure. Different interactions are shown, including ion exchange, van der Waals forces, electrostatic interactions, coordination bonding, and precipitation. The diagram uses color-coded circles to represent reactive sites (green), ions like CO32− and PO43− (red), and exchangeable metal ions (blue). Metal ions, labeled “M”, are shown participating in different adsorption processes. Fig. 3 also highlights two key aspects of the adsorption process: surface diffusion, where ions move along the adsorbent's surface, and intra-particle diffusion, where ions penetrate the adsorbent's pores. This comprehensive visual effectively demonstrates the multifaceted nature of adsorption, encompassing both surface phenomena and internal material interactions, providing a clear overview of the various adsorption mechanisms.
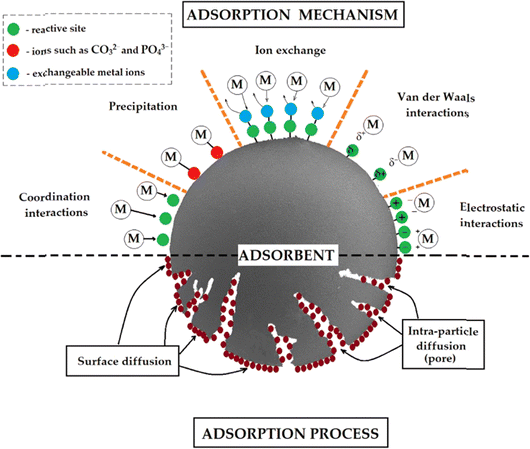 |
| Fig. 3 Shows the proposed adsorption mechanism of ion removal. Reproduced from ref. 79 with permission from MDPI, copyright 2022. | |
4. Factors affecting adsorption performance
The performance of the adsorption process is influenced via a combination of factors related to the adsorbent, the adsorbate, and the solution conditions.80 The adsorbent properties, like surface area, pore size distribution, and surface chemistry, involve crucial in determining the accessibility and affinity of the adsorbate molecules. The adsorbate's molecular size, structure, and solubility also significantly impact the adsorption capacity.81 Solution conditions, including pH, temperature, and ionic strength, may affect the speciation, solubility, and the interactions among the adsorbate and adsorbent. Additionally, operational parameters, like contact time and mixing, influence the mass transfer and the establishment of adsorption equilibrium. Understanding and optimizing these multifaceted factors is essential for designing, operating, and performing adsorption-based processes in various applications, like water remediation, air purification, and catalysis.82
Polymeric adsorbents differ from other adsorbents in several important routes. They may be chemically modified with tailored hydrophilic groups to improve surface chemistry and target specific pollutants, allowing for more customized and efficient adsorption.83 Many polymeric adsorbents also exhibit a multilevel porous structure consisting of submicron cavities and interconnected mesopores, which upgrade adsorption capacity and selectivity. Additionally, polymeric adsorbents, especially those derived from biomaterials, are considered more environmentally friendly and offer advantages in terms of regeneration and reusability compared to traditional adsorbents.84 The detailed characterization and modeling of polymeric adsorbents offer valuable insights for optimizing their design and performance for diverse applications in water remediation, environmental remediation, and industrial purification processes, where their versatility, selectivity, and sustainability make them a preferred choice in many cases. One of the key advantages of polymeric adsorbents is their ability to be chemically modified with tailored hydrophilic groups.85 Unlike traditional adsorbents, which often have fixed surface chemistry, polymers may be synthesized or modified to incorporate specific hydrophilic groups that upgrade their adsorption properties. For instance, ion exchange resins may be tailored to selectively adsorb ions based on their charge and affinity, making them highly effective in water softening and purification processes. Polymeric adsorbents also allow for surface modifications targeting specific pollutants or molecules of interest. This customization is particularly valuable in applications where the removal of specific contaminants, like heavy ions or organic pollutants, is required. By optimizing the surface chemistry through chemical modification, polymeric adsorbents may achieve higher selectivity and efficiency than traditional materials. Another distinguishing feature of many polymeric adsorbents is their multilevel porous structure.86 These materials often exhibit a hierarchy of pores, including micropores, mesopores, and sometimes macropores, contributing to their high adsorption capacity and selectivity. The submicron cavities and interconnected mesopores offer ample surface area for interactions with target molecules, allowing for rapid diffusion of pollutants into the adsorbent matrix. This porous structure is advantageous in several routes. It increases the adsorption capacity via maximizing the available surface area and facilitates the accessibility of adsorbate molecules to active sites within the polymer matrix. This feature is particularly beneficial in dynamic environments where rapid adsorption kinetics are necessary for efficient pollutant removal. Polymeric adsorbents, especially those derived from biomaterials or renewable sources, are often considered more environmentally friendly than traditional adsorbents like activated carbon, which may be derived from fossil fuels.84 Biomass-derived polymers, like chitosan or cellulose-based adsorbents, offer the dual benefits of biodegradability and sustainability. They may be produced from agricultural waste or renewable feedstocks, reducing the environmental footprint associated with their manufacturing and disposal. Furthermore, polymeric adsorbents typically exhibit advantages in regeneration and reusability.87 Unlike some traditional adsorbents that may require energy-intensive processes for regeneration or disposal after a single use, many polymeric materials may be regenerated through simple processes like washing or desorption with appropriate solvents. This capability reduces operational costs and minimizes waste generation, aligning with sustainable practices in waste management and resource conservation.88 The detailed characterization and modeling of polymeric adsorbents are crucial in optimizing their design and performance for specific applications. Via understanding the structure–property relationships through advanced modeling techniques like molecular dynamics simulations or adsorption isotherm modeling, researchers may tailor polymeric adsorbents to meet the requirements of diverse industrial processes.89 This optimization process includes adjusting pore size distribution, surface functionalization, and material composition to upgrade adsorption efficiency, selectivity, and durability under varying environmental conditions. Polymeric adsorbents are extensively used in water remediation applications, where they are employed for the removal of heavy ions, organic pollutants, and arising contaminants from contaminated water sources. Their ability to selectively target specific contaminants while minimizing the release of harmful via-products makes them indispensable in municipal water purification systems and industrial wastewater remediation plants. In environmental remediation, polymeric adsorbents contribute to the cleanup of polluted sites via sequestering hazardous substances from soil, groundwater, or air.90 The versatility of these materials allows for the development of tailored solutions to address complex pollution challenges, ranging from oil spill remediation to the containment of radioactive contaminants. Beyond environmental applications, polymeric adsorbents involve crucial in industrial purification processes across sectors like pharmaceuticals, food and beverage, and biotechnology. They purify high-value products, separate biomolecules, and recover valuable compounds from process streams. Their high affinity and selectivity enable efficient separation and purification steps, contributing to the overall quality and yield of final products.91 Table 2 outlines the factors affecting polymer adsorption offer both advantages and disadvantages. A larger surface area increases adsorption capacity and enhances contaminant removal efficiency, but often requires complex manufacturing processes and higher production costs. Appropriate pore size improves selectivity and allows for targeted adsorption of specific molecules; however, small pores can limit the adsorption of larger molecules, while large pores reduce overall surface area. Functional groups can be tailored for specific contaminants, increasing selectivity and adsorption strength, but may require complex chemical modifications and can affect the polymer's stability or reusability. Optimizing pH can maximize adsorption and enable pH-responsive behavior, but it requires careful control of solution conditions, limiting applications in environments with variable pH. Higher temperatures can increase adsorption rates and facilitate temperature-responsive systems, yet they may negatively impact polymer stability and lead to higher energy costs. Polymer composition can be designed for specific applications, including biodegradable or sustainable options, though this often involves extensive research and higher production costs. A higher degree of cross-linking enhances mechanical stability and reusability, but may reduce polymer flexibility and affect adsorption kinetics. Longer contact time generally increases adsorption and allows equilibrium to be reached, although it can reduce throughput and increase operational costs. Higher initial concentrations of contaminants can drive adsorption, but saturation of adsorption sites may occur at very high concentrations, reducing efficiency. Lastly, the presence of competitive ions can enhance selective adsorption and mimic real-world conditions, yet may reduce overall adsorption capacity and require careful design for mixed contaminant systems.
Table 2 Comparing factors affecting polymer adsorption, along with their advantages and disadvantages
Factor |
Description |
Advantages |
Disadvantages |
Molecular weight |
Higher molecular weight polymers have longer chains, affecting adsorption |
Increased adsorption capacity due to more entanglements and surface area |
Higher molecular weight may lead to slower diffusion rates, affecting adsorption kinetics |
Hydrophobicity |
The tendency of the polymer to repel water |
Hydrophobic polymers can adsorb more readily onto hydrophobic surfaces due to favorable interactions |
Hydrophobic polymers may have limited interaction with polar surfaces, reducing versatility |
Concentration |
The concentration of the polymer in solution influences adsorption rates |
Higher concentrations can lead to increased adsorption rates and capacity |
Excessive concentration may lead to aggregation, reducing effective adsorption |
pH of the solution |
The acidity or basicity of the solution can affect polymer charge and solubility |
Optimal pH can enhance adsorption through better charge interactions |
Extreme pH levels can lead to polymer degradation or altered solubility, negatively impacting adsorption |
Ionic strength |
The presence of salts in the solution can screen electrostatic interactions |
Increased ionic strength can enhance adsorption of charged polymers on charged surfaces |
High ionic strength may reduce the effectiveness of electrostatic interactions, leading to lower adsorption |
Surface roughness |
The texture of the substrate surface affects polymer attachment |
Rough surfaces provide more area for polymer attachment, enhancing adsorption capacity |
Excessive roughness may lead to uneven adsorption, complicating the interpretation of results |
Temperature |
Temperature influences the kinetic energy of polymer chains |
Higher temperatures can increase adsorption rates due to enhanced molecular mobility |
Elevated temperatures may lead to polymer degradation or desorption if interactions weaken |
Fluid movement |
The flow conditions of the solution can impact mass transfer to the surface |
Increased fluid movement can enhance mass transfer, leading to faster adsorption rates |
High flow rates may cause shear forces that disrupt adsorbed layers, reducing overall adsorption |
Functional groups |
The presence of specific functional groups on the polymer affects interactions |
Functional groups can promote specific interactions (e.g., hydrogen bonding) that enhance adsorption |
Limited functional groups may restrict the types of surfaces the polymer can effectively adsorb to |
Temperature |
Temperature influences the kinetic energy of polymer chains |
Higher temperatures can increase adsorption rates due to enhanced molecular mobility |
Elevated temperatures may lead to polymer degradation or desorption if interactions weaken |
5. Types of polymeric adsorbents
Polymeric adsorbents are vital in water remediation and purification, offering versatile and sustainable solutions for removing contaminants from water.84 Bio-based polymeric materials, including synthetic polymers like polystyrene sulfonic acid, have been employed as effective flocculants and adsorbents, showcasing advantages like biodegradability, low cost, and renewable nature.92 Polysaccharides, like chitosan and cyclodextrin, have demonstrated remarkable selectivity for ions and aromatic compounds, making them valuable for removing pollutants from wastewater. Extracellular polymeric substances (EPS) offer binding sites for ions, contributing to the sustainable and efficient removal of contaminants from water.93 Porous aromatic frameworks, polymers of intrinsic microporosity, hyper crosslinked polymers, porous organic polymers, porous coordination polymers, conjugated microporous polymers, aromatic polystyrenic polymers, aromatic halogenated polystyrene, aliphatic methacrylate polymers, biopolymers, and proteins have been utilized as adsorbents for the removal of various pollutants from water. These polymeric adsorbents offer advantages like strong mechanical strength, outstanding hydraulics performance, high stability, tunable surface chemistry, and high surface area for fast hazard removal. They offer a sustainable and efficient solution for removing contaminants from water, addressing the pressing need for clean and safe water.94 The choice of polymeric adsorbent depends on factors like the nature of the pollutants, the desired adsorption capacity, and the specific water remediation application, highlighting the versatility and adaptability of polymeric materials in addressing diverse water purification challenges.
5.1. Nano-magnetic polymers (NMPs)
Nano-magnetic polymers are innovative materials combining polymeric adsorbents' advantages with magnetic properties. These adsorbents typically consist of a magnetic core (often iron oxide nanoparticles) coated with a polymer shell.95 Using an external magnetic field, the magnetic core allows easy separation and recovery of the adsorbent from treated water. At the same time, the polymer shell offers the adsorption capacity for various contaminants. NMPs may be tailored to target specific pollutants via modifying the polymer shell with hydrophilic groups. They have shown outstanding performance in removing heavy ions, organic dyes, and pharmaceuticals from water. The magnetic properties of NMPs not only facilitate their recovery but also enable their reuse in multiple remediation cycles, upgrading their cost-effectiveness. Recent research has been devoted to developing environmentally friendly and sustainable NMPs using biopolymers or waste-derived materials as polymer components.96 A series of tetraethylenepentamine-functionalized core–shell structured nanomagnetic Fe3O4 polymers (TEPA-Fe3O4-NMPs) were synthesized. These NMPs were used to adsorb phosphate ions from water. The results showed that the NMPs had a high adsorption capacity for phosphate ions, with the adsorption efficiency increasing with the amount of TEPA hydrophilic groups. The study also investigated the effect of pH, temperature, and initial concentration on adsorption. The results indicated that the NMPs effectively removed phosphate ions from water, making them a potential material for water remediation applications.97 Magnetic polymer nanocomposites' advantages include their high adsorption capacity, superparamagnetic behavior, good compatibility, low toxicity, high surface energy, and large surface area. These properties make them suitable for eliminating targeted molecules from water.98 Ghobashy et al.99 synthesized and characterized Fe3O4/PE magnetic nanocomposites for water remediation and offered a comprehensive overview of these advanced materials' preparation, analysis, and practical application. The synthesis method involves a solvent-based technique where polyethylene (PE) The preparation of PE wax from LDPE through radiation-thermolysis was previously reported.100 In this study, iron oxide (Fe3O4) nanoparticles were incorporated into PE wax to form a composite, which was then grafted with poly(acrylic acid) (PAAc) using gamma radiation. The process involved mixing the PE/Fe3O4 pellets with acrylic acid monomer and a small amount of FeCl3 to inhibit homopolymer formation. This mixture was exposed to gamma radiation from a Co-60 source. The final product, PE-g-PAAc/Fe3O4, was purified through multiple washings and drying steps. This method allows for the functionalization of PE/Fe3O4 composites, upgrading their properties for specific applications. The detailed preparation procedure ensures a controlled synthesis environment, leading to a reproducible and functional nanocomposite. Fig. 4A outlines the gamma irradiation techniques used in the grafting process to create safe polymer-ion nanocomposites with magnetic properties for effective dye removal. This method ensures an even distribution of nanoparticles, which is critical for the consistent performance of the nanocomposite in water remediation applications. Characterization of the Fe3O4/PE nanocomposite was conducted using several advanced techniques. Fourier Transform Infrared Spectroscopy (FTIR) was employed to confirm the successful incorporation of Fe3O4 nanoparticles into the PE matrix.101 The FTIR spectra showed characteristic peaks corresponding to both PE and Fe3O4, indicating the presence of both components in the nanocomposite. Scanning Electron Microscopy (SEM) offered detailed images of the nanocomposite's morphology, revealing a relatively uniform dispersion of Fe3O4 nanoparticles within the PE matrix. This uniform distribution is essential for maximizing the surface area available for adsorption. Thermogravimetric Analysis (TGA) was used to assess the thermal stability and composition of the nanocomposite, confirming the presence of both PE and Fe3O4 components and providing insights into the material's thermal behavior.101,102 The adsorption studies conducted in this research demonstrated the efficacy of the Fe3O4/PE nanocomposite in removing various pollutants from water. To determine the removal efficiency of 0.05 g PE-g-PAAc as dye adsorbents, 3 mL of a test dye solution (5 × 10−5 M) of toluidine blue (TB) was used. At room temperature, the color change was observed after 20 minutes, with clear differences among PE/Fe3O4 and PE-g-PAAc/Fe3O4. Fig. 4B indicated that grafting PAAc onto PE/Fe3O4 upgraded its adsorption efficiency. The decolorization efficiency reached 78% after 1 hour, demonstrating the improved performance of the grafted nanocomposite in dye removal applications. The difference in adsorption performance is illustrated in Fig. 2B, which compares the sequence of dye removal efficiency among (b) PE-g-PAAc/Fe3O4 and (a) PE/Fe3O4. The upgraded efficiency of the grafted nanocomposite is evident, showcasing the impact of PAAc grafting on adsorption properties. Overall, this research offers valuable insights into the development of Fe3O4/PE magnetic nanocomposites as effective materials for water remediation. The combination of magnetic properties, high adsorption capacity, and the potential for further functionalization make these nanocomposites promising candidates for addressing global water pollution. The detailed synthesis and characterization techniques outlined in the study offer a solid foundation for future research and development, establishing the route for implementing these advanced materials in practical water remediation applications.99
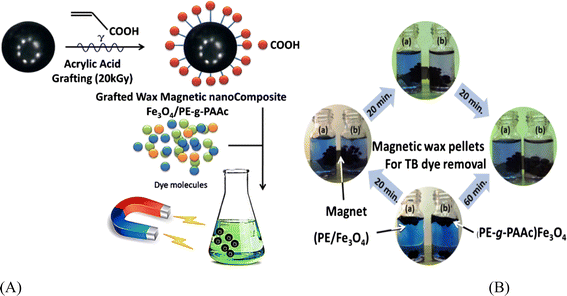 |
| Fig. 4 Schematic representation of the gamma irradiation technique used in grafting to create Fe3O4/PE nanocomposites with magnetic properties for effective dye removal in water remediation applications (A) and comparison of dye removal efficiency among (a) PE/Fe3O4 and (b) PE-g-PAAc/Fe3O4 nanocomposites, illustrating upgraded adsorption capability with PAAc grafting. Adsorption studies show significant improvement in decolorization efficiency, highlighting the impact of grafting on nanocomposite performance (B). Reproduced from ref. 99 with permission from Taylor and Franci, copyright [2016]. | |
Ghobashy et al.103 investigate the synthesis of poly(vinylpyrrolidone)/Fe3O4@SiO2 nanoporous catalyst and evaluate its efficiency for degrading toluidine blue dye using different advanced oxidation processes. The nanoporous catalyst is synthesized using an ultrasonic-assisted gamma irradiation technique and characterized using various methods that confirm its mesoporous nature with a surface area of 43.50 m2 g−1. The catalyst exhibits good stability with negligible iron leaching. The efficiency of the nanoporous catalyst is evaluated for degrading toluidine blue dye using different oxidation processes like photocatalytic degradation, photo-Fenton catalytic degradation, sono-photocatalytic degradation, sono-Fenton degradation, and sono-photo-Fenton degradation. The results show that the degradation rate improves when these processes are combined and further improves via applying a magnetic field. The sono-photo-Fenton degradation under a magnetic field achieves the highest degradation rate of 95.3% after 10 minutes. The generated hydroxyl and superoxide radicals are found to be the main active species responsible for degrading the dye. The nanoporous catalyst exhibits good reusability with stable catalytic activity over three cycles.104 The PVP/Fe3O4@SiO2 nanoporous catalyst integrated with sono-photo-Fenton degradation and magnetic field may be an effective advanced remediation process for wastewater-containing dyes.
Ghobashy et al.100 discuss the development of a magnetic polyethylene wax nanocomposite for selective oil adsorption. Low-density polyethylene pellets were irradiated using gamma rays at 50 kGy and then thermally decomposed to produce polyethylene wax pellets. The wax pellets were then composited with 30% magnetite (Fe3O4) nanoparticles using a melting process. The Fe3O4 nanoparticles dispersed relatively well in the polyethylene wax matrix with 10 and 12 nm sizes. Adding the Fe3O4 nanoparticles increased the melting point of the wax pellets from 107 °C to 111 °C. The irradiated polyethylene wax/Fe3O4 composite showed a rough surface and some cavities, making it suitable for oil/water separation. The magnetic polyethylene wax composite showed a good ability to absorb crude oil, removing up to 98% of the oil after 130 seconds. Overall, the magnetic, durable, superhydrophobic polyethylene wax composite is promising for practical applications in oil absorption and oil/water separation.
5.2. Polysaccharides
Polysaccharides are natural polymers composed of long chains of monosaccharide units. Two prominent polysaccharide-based adsorbents in water remediation are chitosan and cyclodextrin.105 Chitosan, derived from chitin found in crustacean shells, is a versatile adsorbent with a high affinity for heavy ions, dyes, and organic pollutants. Its adsorption capacity stems from the presence of amino and hydroxyl groups that may interact with various contaminants. Chitosan may be easily modified to upgrade its adsorption properties and mechanical strength.106 Cyclodextrins, on the other hand, are cyclic oligosaccharides with a hydrophobic interior cavity and hydrophilic exterior. This unique structure allows cyclodextrins to form inclusion complexes with organic molecules, effectively removing organic pollutants from water. Both chitosan and cyclodextrin-based adsorbents are biodegradable and non-toxic, aligning with the principles of green chemistry. Recent research has been devoted to developing composite materials combining these polysaccharides with other materials to upgrade their adsorption capacity and selectivity for specific contaminants. Ghobashy et al.107 synthesis of phosphorylated chitosan/HEMA interpenetrating polymer networks (IPNs) and their application for ion removal from aqueous solutions. The CS/pHEMA IPNs were prepared using γ-radiation, which induces chitosan and HEMA monomers crosslinking. The gel content and swelling of the IPNs increased with higher HEMA concentration and irradiation dose. The CS/pHEMA IPNs were chemically modified via phosphorylation to introduce phosphate groups, resulting in phos-(CS/pHEMA). The phos-(CS/pHEMA) IPNs were characterized using various techniques to confirm the phosphorylation and structure. The phos-(CS/pHEMA) IPNs showed good adsorption capacity for Ca2+, Cu2+, and Zn2+ ions from aqueous solutions. The adsorption capacity followed the order Cu+ > Zn2+ > Ca2+. The adsorption of ion ions onto phos-(CS/pHEMA) fitted the Langmuir isotherm model and followed pseudo-second-order kinetics. The phosphorylated IPNs show potential for effectively removing ion ions from contaminated water due to the presence of phosphate groups that may chelate with ion ions. Ge et al.108 discussed the synthesis of a novel melamine-grafted chitosan adsorbent for the selective removal of Pb2+ and Hg2+ ions from wastewater. The melamine-grafted chitosan adsorbent showed high selectivity and uptake for Pb2+ and Hg2+ ions. It could be regenerated for reuse, making it an effective adsorbent for removing these heavy ions from wastewater. A melamine-grafted chitosan (GMCS) adsorbent was synthesized using glutaraldehyde as a crosslinker among chitosan and melamine. The GMCS adsorbent contained abundant amine groups that could effectively chelate with ion ions. The GMCS adsorbent showed higher selectivity and uptake for Pb2+ and Hg2+ ions compared to unmodified chitosan. Under optimized conditions, the maximum adsorption capacities were 618.2 mg g−1 for Pb2+ and 490.7 mg g−1 for Hg2+. The adsorption of Pb2+ and Hg2+ on GMCS followed the pseudo-second-order kinetic model and Langmuir isotherm model. The adsorption was an endothermic and spontaneous chemical process. The GMCS adsorbent could be efficiently regenerated through desorption and maintained 80% of its adsorption capacity even after five cycles of adsorption–desorption experiments.108 discusses the synthesis and characterization of cellulose-based adsorbents to remove Ni2+, Cu2+, and Pb2+ ions from aqueous solutions. Cellulose was extracted from rice husk, and graft copolymers were prepared via grafting N-isopropyl acrylamide and acrylic acid onto the cellulose backbone. The grafted copolymers showed a higher uptake of ion ions than the ungrafted cellulose. The ion sorption followed a pseudo-second-order kinetic model and Langmuir adsorption isotherm. Under optimized conditions, almost complete sorption of Pb2+ ions was observed for the cellulose-g-NIPAM-co-AAc copolymer, while 74.5% of Ni2+ and 77.5% of Cu2+ ions were sorbed. The grafted copolymers showed potential as efficient adsorbents for removing toxic ion ions from wastewater due to their low cost, biodegradability, and recyclability. Kumar et al.109 discussed the environmental and health concerns posed by disposing of toxic effluents containing dyes and heavy ion ions from various industries into water bodies. Traditional techniques for removing these hazardous ion ions from water consist of coagulation, reverse osmosis, and biopolymer adsorption. The focus is on low-cost adsorbents derived from agricultural wastes, particularly cellulose, which is abundant in nature and offers outstanding materials for adsorption. The study reports on the grafting of NIPAM and AAc onto cellulose extracted from rice husk to prepare copolymers for the sorption of Ni2+, Cu2+, and Pb2+ ions from aqueous solutions. The grafting parameters, synthesis of binary graft copolymers, and characterization of the pure cellulose and graft copolymers are detailed. The characteristics of the cellulose and its graft copolymers consist of morphological changes, XRD patterns, FTIR analysis, thermal stability, and swelling properties under different pH conditions. Cellulose was extracted from rice husk using a greener, total chlorine-free, and ultrasonically assisted method, exhibiting characteristic XRD peaks at 15.36°, 22.33°, and 34.55°, corresponding to the (101), (002), and (004) diffraction planes, respectively. FTIR analysis revealed peaks at 1163.7 and 1057.8 cm−1 due to grafting at the eOH situated at C2, C3, and C6 atoms of each β-D-glucopyranose unit of the cellulose backbone. Thermogravimetry showed weight loss at ∼200 °C and further weight loss at 368 °C due to glycosidic linkage decomposition, with exothermic peaks at 338.4, 377.6, and 439.5 °C in the DTG thermogram and 390.4 °C and 442 °C in the DTA thermogram. The graft copolymer Cell-g-NIPAM demonstrated thermal stability from 100–200 °C, a 17.0% weight loss from 200 to 300 °C, a sharp 52.8% weight loss up to 400 °C, and an FDT at 561.3 °C with 80% weight loss. Cell-g-NIPAM-co-AAc showed a nominal 6.6% weight loss up to 165.6 °C and a continuous 96.0% weight loss up to 553.8 °C, its FDT, with exothermic peaks in the DTG and DTA thermographs due to the degradation of amide groups and decarboxylation of the COOH group of the AAc moiety. These characteristics offer insights into cellulose's structural and thermal properties and its graft copolymers, aiding in their application for ion sorption.110 The optimized grafting conditions and synthesis of binary graft copolymers show promising results for ion sorption capacities. The copolymers exhibit higher sorption efficiencies than ungrafted cellulose and previously reported sorbents. The study highlights the potential of cellulose-based graft copolymers as effective and low-cost adsorbents for removing heavy ion ions from water, offering a sustainable solution to environmental pollution.111
5.3. Extracellular polymeric substances (EPS)
Extracellular polymeric substances (EPS), biopolymers excreted via microorganisms, have gained considerable attention as adsorbents for removing organic and inorganic pollutants from aqueous solutions.112 EPS's unique composition and structure, which consist of various hydrophilic groups like carboxyl, phosphoric, sulfhydryl, phenolic, and hydroxyl groups, significantly upgrade their application in biosorption processes. EPS consists of a complex mixture of proteins, lipids, carbohydrates, and nucleic acids, each contributing to the substance's ability to bind and remove pollutants. The diverse hydrophilic groups present in EPS are critical in their effectiveness as biosorbents.113 Carboxyl groups, for instance, may form strong ionic bonds with ion ions, while hydroxyl and phenolic groups may engage in hydrogen bonding with organic pollutants. Phosphoric and sulfhydryl groups further expand the range of interactions EPS may participate in, making them highly versatile in pollutant removal applications. One significant advantage of EPS is their high molecular weight, which offers numerous binding sites and upgrades their adsorption capabilities through van der Waals forces. Zhang et al.114 demonstrated this with their research on the adsorption of Basic Blue 54, where the high-molecular-weight EPS exhibited outstanding adsorption properties. The specific structure of EPS, combined with their substantial binding sites, allows for effective interaction with various pollutants, contributing to their superior adsorption performance. In practical applications, EPS has been employed in various biosorption processes to treat wastewater containing complex mixtures of pollutants. A notable study involved the decolorization of a complex Remazol dye effluent using a composite of polyaniline (PANI) and EPS. The effluent contained Remazol Brilliant Blue R, Remazol Black, Remazol Violet, Remazol Orange, sodium chloride, sodium carbonate, sodium hydroxide, and acetic acid.115 The composite material demonstrated upgraded dye removal capabilities, indicating its potential as an effective adsorbent for treating industrial dye effluents. This study115 underscores the adaptability of EPS in composite materials, further broadening their application scope in water remediation. The ability of EPS to bind with both organic and inorganic pollutants make them particularly valuable in addressing a broad range of contaminants. Organic pollutants, like dyes, pesticides, and pharmaceuticals, may be effectively adsorbed via the hydrophilic groups within EPS. In contrast, inorganic pollutants, including heavy ions like lead, cadmium, and mercury, may be sequestered through complexation and ion exchange mechanisms. This versatility is a key factor in the growing interest in EPS for environmental remediation.116 Furthermore, the eco-friendly nature of EPS, naturally produced via microorganisms, presents a sustainable alternative to traditional chemical adsorbents. EPS in water remediation aligns with the principles of green chemistry, emphasizing the importance of using environmentally benign materials and processes. The biodegradability of EPS also ensures that they do not introduce secondary pollution, a significant advantage over synthetic polymers and chemical adsorbents that may persist in the environment. Research into optimizing EPS production and its application in various forms, like composites or immobilized on different substrates, continues to expand.117 Studies are focusing on upgrading the efficiency and specificity of EPS-based adsorbents, exploring their potential in removing arising contaminants and scaling up the technology for industrial applications. Integrating EPS with other biopolymers and nanomaterials also promises to create hybrid adsorbents with superior performance characteristics.118 Oladoja et al.119 investigated the role of extracellular polymeric substances (EPS) in biofilm formation and its impact on wastewater remediation. The authors found that EPS involved a significant role in the aggregation of microorganisms, upgrading their ability to remove pollutants from wastewater. The study highlighted the importance of understanding EPS dynamics in biofilms for effective wastewater remediation strategies. Another study published120 explored using EPS as a flocculant for wastewater remediation. The authors demonstrated that EPS could effectively flocculate and remove suspended solids from wastewater, improving the overall water quality. This study emphasized the potential of EPS as a sustainable and eco-friendly alternative to traditional chemical flocculants. A research article published121 examined the role of EPS in sludge remediation and dewatering. The authors found that EPS is critical in aggregating and dewatering sludge, making it easier to handle and dispose of. This study highlighted the importance of understanding EPS dynamics in remediation for improved sludge management practices. A study112 investigated the use of EPS in bioreactors for wastewater remediation. The authors demonstrated that EPS could upgrade the performance of bioreactors via improving the biofilm formation and activity of microorganisms. This study emphasized the potential of EPS in designing more efficient and sustainable bioreactors for wastewater remediation. The formation of biofilms, which are complex communities of microorganisms embedded in a matrix of extracellular polymeric substances (EPS), is a critical aspect of microbial life. EPS, primarily composed of polysaccharides, proteins, nucleic acids, and phospholipids, serves as a protective array that stabilizes the local environment of bacteria against various stresses. This matrix involves a crucial role in the resistance of biofilm-associated microorganisms to antimicrobial agents and environmental challenges. In the context of defense against the antimicrobial activity of nanoparticles (NPs), particularly silver nanoparticles (AgNPs), certain bacteria, like Bacillus subtilis, have been found to produce EPS as a defense mechanism. B. subtilis has demonstrated the extracellular production of polysaccharides and polygamma-glutamate (PGA) as a defense against AgNPs. The extracellular matrix rich in polysaccharides, constituting a significant portion of the EPS, has been shown to sequester NPs and ion ions, leading to their precipitation and subsequent inactivation of their antimicrobial activity. Additionally, the PGA produced via B. subtilis during its stationary growth phase contains amino and carboxyl groups that interact with AgNPs, binding to their surface and inactivating their bactericidal activity. Table 3 highlights the intricate inter involve among microbial biofilms, EPS, and their defense mechanisms against antimicrobial agents, shedding light on the adaptive strategies employed via microorganisms to thrive in challenging environments. Understanding biofilm formation dynamics and EPS's role in microbial defense mechanisms is crucial for developing effective strategies for controlling biofilm growth and addressing medical, industrial, and environmental challenges. The interaction of nanoparticles (NPs) with extracellular polymeric substances (EPS) and the subsequent formation of a protein corona have been observed to interfere with the antimicrobial activity of NPs.137 In the case of Escherichia coli, the production of protein-like substances, amino acids, and flagellin has been reported as a defense mechanism against the bactericidal action of NPs. However, the presence of these components tends to destabilize the NPs via joining their surface and modifying their zeta potential, leading to their agglomeration and inactivation.
Table 3 Extracellular substances produced via bacterial strains for resistance against nanoparticles
Strain (reference) |
Extracellular substances |
Nanocomposite |
B. subtilis122 |
Polysaccharide |
AgNP |
B. subtilis123 |
PGA |
AgNP |
E. coli CCM 3954 (ref. 124) |
Flagellin |
AgNP |
E. coli125 |
Protein-like substances |
ZnONP/SiO2NP |
E. coli K-12 MG1655 (ref. 126) |
Amino acids |
AgNP |
P. aeruginosa ATCC 19606 (ref. 127) |
Phenazine pigments |
AgNP |
P. aeruginosa PAO1 (ref. 128) |
Pyocin overproduction |
AgNP |
E. faecalis ATCC 29212 (ref. 129) |
VWA domain-containing protein |
AgNP |
Staphylococcus aureus130 |
Exopolysaccharides |
AuNP |
Klebsiella pneumoniae131 |
Lipopolysaccharides |
AgNP |
Pseudomonas putida132 |
Alginate |
AgNP |
Acinetobacter baumannii133 |
Outer membrane vesicles |
AgNP |
Mycobacterium smegmatis134 |
Trehalose dimycolate |
CuNP |
Shewanella oneidensis135 |
Extracellular polymeric substances |
MnO2NP |
Bacillus cereus136 |
Polysaccharides |
TiO2NP |
Table 3 offers an overview of various bacterial strains and the extracellular substances they produce to resist different nanoparticles (NPs). Bacillus subtilis is highlighted with its polysaccharides and poly gamma-glutamate (PGA) production to counteract silver nanoparticles' antimicrobial effects (AgNPs). Escherichia coli strains demonstrate a range of defensive extracellular substances, including flagellin, protein-like substances, and amino acids, against AgNPs and a combination of zinc oxide (ZnONP) and silicon dioxide (SiO2NP) nanoparticles. Pseudomonas aeruginosa employs phenazine pigments and pyocin overproduction as a defense mechanism against AgNPs. Similarly, Enterococcus faecalis utilizes a VWA domain-containing protein for AgNP resistance. Expanding beyond these primary examples, Staphylococcus aureus produces exopolysaccharides to combat gold nanoparticles (AuNPs), while Klebsiella pneumoniae relies on lipopolysaccharides against AgNPs.138 Pseudomonas putida secretes alginate as a protective measure against AgNPs, and Acinetobacter baumannii uses outer membrane vesicles for similar purposes. Mycobacterium smegmatis produces trehalose dimycolate to resist copper nanoparticles (CuNPs), and Shewanella oneidensis employs extracellular polymeric substances to mitigate the effects of manganese dioxide nanoparticles (MnO2NP). Lastly, Bacillus cereus produces polysaccharides to defend against titanium dioxide nanoparticles (TiO2NP). This variety of extracellular substances highlights bacteria's diverse strategies to survive in environments containing antimicrobial nanoparticles, demonstrating the complexity and adaptability of microbial defense mechanisms.
This phenomenon has been observed to interfere with NPs' antimicrobial activity, as the formation of a protein corona around the NPs, even in NPs exposed to culture media, has been shown to diminish their antibacterial activity.139 Proteomic studies have identified common proteins that bind to NPs, including α-1-antiproteinase, α-2-HS-glycoprotein, apolipoprotein A-I, A-II, C-III, keratin, type II cytoskeletal, keratin type II cytoskeletal, and serum albumin. The presence of these proteins in the corona, similar to those found in serum, has been implicated in diminishing the antibacterial activity of NPs. This research137 sheds light on the complex interinvolve among NPs, EPS, and microbial defence mechanisms, highlighting the potential impact of the protein corona on the antimicrobial activity of NPs. Understanding these interactions is crucial for developing effective strategies for applying NPs in antimicrobial and environmental contexts. Fig. 5 offers a comprehensive overview of the complex interactions among nanoparticles (NPs) and biological systems, focusing on forming protein coronas. The protein corona refers to the layer of proteins that adsorbs onto the surface of NPs when they enter a biological environment. This phenomenon has both advantages and disadvantages, clearly delineated in the image. On the disadvantage side, protein coronas may mask the intended functionality of NPs and potentially trigger unwanted immune responses, which could limit their therapeutic efficacy or cause adverse effects. However, the advantages are significant: pre-coating NPs with specific proteins may help them escape immune recognition and prevent aggregation, improving their circulation time and stability.
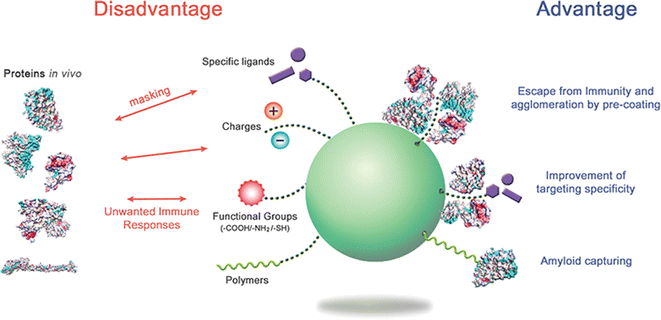 |
| Fig. 5 Advantages and disadvantages of protein corona formation on nanoparticles (NPs) in biological systems. The left side illustrates the disadvantages, including masking NP functionality and potential unwanted immune responses. The right side showcases the advantages, like escape from immunity and agglomeration through pre-coating, improved targeting specificity, and amyloid capturing. The central green sphere represents a nanoparticle surrounded via various elements that may influence protein corona formation, including specific ligands, surface charges, hydrophilic groups, and polymers. By carefully regulating protein coronas through appropriate surface modifications and NP selection, researchers may improve the biological behavior and efficacy of NPs in vivo. Structural studies of corona proteins on NP systems may offer valuable insights for optimizing NP design and functionality in biomedical applications. Reproduced from ref. 140 with permission from [Taylor and Francis], copyright [2020]. | |
Moreover, this coating may upgrade targeting specificity, allowing NPs to reach their intended destinations more effectively. The ability to capture amyloids associated with various neurodegenerative diseases is another notable advantage.141 The central green sphere represents the NP, surrounded via multiple elements that may be manipulated to control protein corona formation, including specific ligands, surface charges, hydrophilic groups, and polymers. This level of control suggests that researchers may fine-tune NP properties to achieve desired biological behaviours. Fig. 5 emphasizes that understanding and regulating protein corona formation is crucial for optimizing nanoparticle-based technologies in biomedical applications, potentially leading to more effective drug delivery systems, diagnostic tools, and therapeutic strategies.
5.4. Porous aromatic frameworks
Porous aromatic frameworks (PAFs) are synthetic polymers characterized by their high surface area, tunable pore size, and chemical stability.142 These materials are typically synthesized through the polymerization of aromatic monomers, resulting in a rigid, three-dimensional network with well-defined pores. The aromatic nature of PAFs offers strong π–π interactions with organic contaminants, making them particularly effective for removing aromatic compounds from water. The high surface area and porosity of PAFs contribute to their outstanding adsorption capacity, while their chemical stability allows for their use in various water remediation conditions. PAFs may be further functionalized with specific groups to upgrade their selectivity towards certain contaminants or improve their hydrophilicity for better performance in aqueous environments.143 Recent research has been devoted to developing PAFs with hierarchical pore structures, incorporating catalytic sites for simultaneous adsorption and degradation of pollutants, and exploring sustainable synthesis methods using bio-based precursors. The versatility and tunability of PAFs make them promising candidates for advanced water purification applications, especially for removing persistent organic pollutants.
Porous aromatic frameworks (PAFs) represent a promising class of materials that have garnered significant interest in recent years for their potential applications in water remediation. These frameworks are characterized via their highly porous structures, which offer large surface areas and tunable pore sizes, making them ideal for adsorption-based processes to remove contaminants from water. One of the key studies, published via,144 offers a comprehensive review of PAFs' capabilities in water purification. This review covers three main areas: adsorption, photocatalysis, and membrane filtration. PAFs exhibit outstanding adsorption capacities for organic pollutants, heavy ions, and microorganisms due to their extensive surface areas and the ability to tailor their pore structures to match specific contaminants. The review underscores the importance of understanding PAF synthesis, modification, and performance under different water remediation conditions, highlighting their potential as versatile adsorbents. In another notable contribution, Zhao et al.145 explore using PAF-based adsorbents to remove organic dyes from wastewater. Their research demonstrates that PAFs exhibit high adsorption capacities, possess rapid adsorption kinetics, and may be easily regenerated for reuse. This study emphasizes the practical applicability of PAFs in industrial settings where efficient and cost-effective removal of dye pollutants is crucial.146
Moving to membrane applications, Fajal et al.147 investigate PAF-based membranes tailored for the selective removal of heavy ion ions from water. These membranes leverage PAFs' inherent properties, like high selectivity and permeability, demonstrating promise in upgrading water purification processes via effectively filtering out toxic ion contaminants while allowing the passage of essential nutrients and ions. Furthermore, it contributes to understanding PAFs' role in tackling arising contaminants like pharmaceuticals and personal care products in water systems. Their research highlights PAFs' ability to adsorb organic micropollutants efficiently, offering a sustainable approach to addressing these increasingly prevalent water quality challenges.148 Overall, these studies underscore the versatility and effectiveness of PAFs in various facets of water remediation. Their tunable pore structures, large surface areas, and robust chemical stability make them highly suitable for applications ranging from adsorption and photocatalysis to membrane filtration. As research continues to advance in this field, further exploration of PAF-based materials holds promise for developing advanced water purification technologies that are both efficient and environmentally sustainable. Based on recent research findings, porous aromatic frameworks (PAFs) have emerged as highly effective materials for removing diverse pollutants from water. PAF-based adsorbents demonstrate exceptional capability in adsorbing organic dyes and micropollutants like pharmaceuticals and personal care products, showcasing high adsorption capacities and rapid kinetics. Moreover, PAF-based membranes exhibit notable selectivity and permeability in removing heavy ion ions, underscoring their potential to upgrade water purification processes.149 The tunable properties of PAFs allow for tailored materials optimized to address specific water remediation challenges across a broad spectrum of organic and inorganic contaminants. While exact details on pollutant removal efficiencies via PAFs vary depending on material composition and environmental conditions, continuing research aims to refine and optimize PAF-based technologies for future applications in sustainable water remediation solutions. Fig. 6 illustrates the preparation of submicron PAF-56 particles and their application in pervaporation for the recovery of n-butanol. PAF-56 particles were incorporated into polydimethylsiloxane (PDMS) membranes to study their effects on hydrophilicity, swelling degree, partition coefficient, and pervaporation performance. The study found that the PAF-56/PDMS mixed matrix membranes (MMMs) disinvolved n-butanol sorption-selectivity, with permeation flux and separation factor increasing as the size of PAF-56 particles decreased at the same loading. The best performance was observed at 2–2.5% loading of PAF-56, with a significant increase in separation factor and permeation flux compared to pristine PDMS membranes. The study demonstrated the size effects of PAF-56 particles and the potential of MMMs to recover n-butanol through pervaporation.150
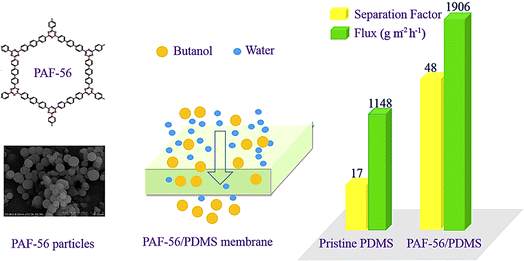 |
| Fig. 6 Demonstrates the improved performance of a PAF-56 (Porous Aromatic Framework-56) incorporated PDMS (polydimethylsiloxane) membrane for butanol–water separation. The image showcases the chemical structure of PAF-56 and an SEM micrograph of PAF-56 particles, illustrating their porous nature and spherical morphology. A schematic in the center depicts the PAF-56/PDMS membrane selectively separating larger butanol molecules from smaller water molecules. The bar graph on the right compares the separation factor and flux of a pristine PDMS membrane to the PAF-56/PDMS composite membrane. The data reveals that the composite membrane significantly outperforms the pristine PDMS, with a higher separation factor (48 vs. 17) and improved flux (1906 vs. 1148 g m−2 h−1), demonstrating upgraded efficiency in butanol–water separation. Reproduced from ref. 150 with permission from [Elsevier], copyright [2019]. | |
5.5. Polymers of intrinsic microporosity
Polymers of intrinsic microporosity (PIMs) are a unique material class that combines high free volume with a rigid and contorted molecular structure. Unlike conventional porous materials, PIMs derive their microporosity from their molecular structure rather than a network of interconnected pores.151 This intrinsic microporosity results in high surface areas and good permeability, making PIMs outstanding candidates for water purification applications. The rigid and contorted structure of PIMs creates a network of interconnected voids that may effectively trap and remove contaminants from water. PIMs may be synthesized using different monomers, allowing for tuning their chemical properties and pore characteristics. These polymers have shown particular promise in removing organic micropollutants, like pharmaceuticals and personal care products, from water. The non-crystalline nature of PIMs also makes them resistant to fouling, a common problem in membrane-based water remediation processes. Recent research has been devoted to upgrading the stability of PIMs in aqueous environments, developing composite materials incorporating PIMs, and exploring their potential in advanced separation processes like forward osmosis.152
This distinctive feature endows PIMs with exceptionally high surface areas and controllable pore structures, making them ideal candidates for adsorption-based and membrane-based water remediation technologies. The development and application of PIMs in water remediation have been extensively studied via numerous recent research articles. One of the seminal works in this field is the review article via McKeown and Budd,153 which offers a comprehensive overview of the development and applications of PIMs, including their potential use in water remediation to remove organic pollutants and heavy ions. This article laid the foundation for subsequent research exploring the specific applications of PIMs in addressing various water hazard issues. Building upon this foundational work, Satilmis et al.154 demonstrated the efficacy of PIM-1, a specific type of PIM, in the adsorptive removal of organic pollutants from water. Their study showcased the high adsorption capacity and selectivity of PIM-1 towards organic contaminants like phenol and dyes. The authors attributed this exceptional performance to the unique pore structure and high surface area of PIM-1, which facilitate the rapid and efficient capture of organic molecules from aqueous solutions. In addition to organic pollutants, PIMs have shown remarkable potential in removing heavy ion ions from water. Another study via Ye et al.155 explored the application of PIMs, specifically PIM-1 and PIM-7, for the adsorptive removal of heavy ion ions like Cu2+, Pb2+, and Cd2+ from aqueous solutions. This research elucidated the adsorption mechanisms and the factors influencing the performance of PIMs in heavy ion removal. The authors found that the high surface area and the presence of hydrophilic groups in PIMs contribute to their strong affinity for ion ions, enabling efficient removal even at low concentrations. The versatility of PIMs in water purification was further highlighted in both membrane-based and adsorption-based water purification processes. The researchers showcased the ability of PIMs to remove a broad range of organic and inorganic contaminants from water, emphasizing their potential as multifunctional materials for comprehensive water remediation solutions. One of the key advantages of PIMs in water remediation applications is their tunable pore structure. Via modifying the molecular design of PIMs, researchers may tailor the pore size distribution and surface chemistry to target specific contaminants.156 This flexibility allows for the development of highly selective adsorbents and membranes that may efficiently remove pollutants while maintaining high water flux rates. Furthermore, the chemical stability of PIMs enables their use in various harsh environments, making them suitable for diverse water remediation scenarios. In membrane-based water purification, PIMs offer several advantages over conventional polymeric membranes. Their intrinsic microporosity facilitates upgraded permeability without compromising selectivity, addressing the primary challenges in membrane technology known as the permeability-selectivity trade-off. PIM-based membranes have shown promising results in nanofiltration, reverse osmosis, and gas separation applications, demonstrating their potential to revolutionize membrane-based water remediation processes.155 The adsorption properties of PIMs have been extensively studied for the removal of various water contaminants. Their high surface area and tunable pore structure enable rapid adsorption kinetics and high adsorption capacities for various pollutants, including organic dyes, pharmaceuticals, and heavy ions. Moreover, the regeneration and reusability of PIM-based adsorbents have been demonstrated, highlighting their potential for sustainable and cost-effective water remediation solutions. Recent research has also been devoted to developing composite materials incorporating PIMs to upgrade their performance in water remediation applications further. For instance, integrating PIMs with other nanomaterials, like graphene oxide, carbon nanotubes, or ion-organic frameworks, has created hybrid materials with synergistic properties. Compared to their components, these composites often exhibit improved adsorption capacities, selectivity, and mechanical stability.17 Despite the promising results obtained in laboratory studies, the large-scale application of PIMs in water remediation still faces several challenges. These consist of PIM synthesis's scalability, PIM-based materials' long-term stability under real-world conditions, and their production and implementation cost-effectiveness. Addressing these challenges requires continued research and development attempts and collaboration among academic institutions and industry partners. The potential environmental impact of PIMs in water remediation applications is significant. Via enabling more efficient and selective removal of contaminants from water, PIMs could improve water quality and increase access to clean water resources worldwide. Furthermore, regenerating and reusing PIM-based materials aligns with sustainability and circular economy principles, potentially reducing the environmental footprint of water remediation processes. In conclusion, polymers of intrinsic microporosity represent a promising class of materials for addressing the global challenge of water pollution.157 Their unique structural properties, high surface areas, and tunable pore structures make them versatile candidates for various water remediation applications, including adsorption-based and membrane-based processes. The growing body of research on PIMs in water remediation demonstrates their potential to remove a broad range of organic and inorganic contaminants efficiently. As research in this field continues to advance, it is anticipated that PIMs will be increasingly important in developing next-generation water purification technologies, contributing to global attempts to ensure access to clean and safe water resources. Fig. 7a illustrates the preparation procedure of PIM-1 and C-PIM-1 membranes. The process begins with the synthesis of PIM-1 through polycondensation of 5,5′,6,6′-tetrahydroxy-3,3,3′,3′-tetramethyl-1,1′-spirobisindane (TTSBI) and 2,3,5,6-tetrafluoroterephthalonitrile (TFTPN). The PIM-1 membrane is then prepared using a simple solution casting method, where a PIM-1 solution in chloroform is poured into a glass dish, and the solvent is allowed to evaporate slowly at room temperature. The resulting PIM-1 membrane is then subjected to controlled thermal remediation under N2/H2 atmosphere to produce the carbonaceous PIM-1 (C-PIM-1) membrane. Fig. 7b shows photographs of the PIM-1 and C-PIM-1 membranes. The PIM-1 membrane is yellow and transparent, while the C-PIM-1 membrane is glittering-grey and opaque after carbonization. Fig. 7c represents the XPS C 1s spectra of the PIM-1 and C-PIM-1 membranes. The spectra show that the carbon content in the C–C bond (284.4 eV) of the C-PIM-1 membrane is much larger than that of the PIM-1 membrane. This indicates an increase in carbon content and a change in the chemical bonding structure after carbonization. Fig. 7d involves the Raman spectra of the PIM-1 and C-PIM-1 membranes. The C-PIM-1 membrane shows clear D (1310 cm−1) and G (1595 cm−1) band peaks, which correspond to graphitic carbon structures. These peaks are not observed in the PIM-1 membrane spectrum, indicating the formation of graphitic structures during the carbonization process. Fig. 7e shows the pore size distributions of the PIM-1 and C-PIM-1 (40% carbonization) membranes. The graph indicates that the PIM-1 membrane has a median pore size of 0.824 nm, while the C-PIM-1 membrane has a slightly smaller median pore size of 0.778 nm. This suggests that the carbonization process slightly reduces the pore size but generally preserves the microporous structure of the original PIM-1 membrane.
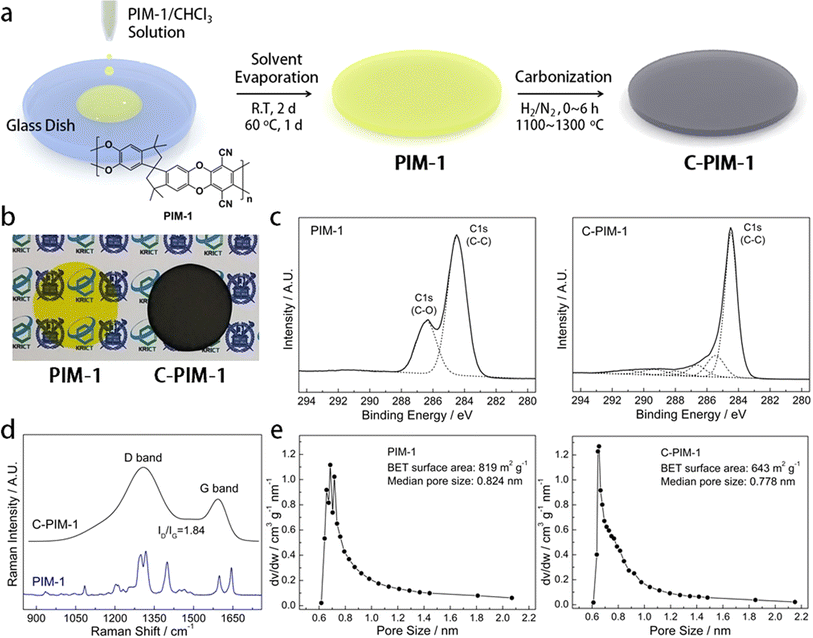 |
| Fig. 7 (a) Preparation of PIM-1 and C-PIM-1 membranes: synthesis via polycondensation, solution casting of PIM-1 in chloroform, and controlled thermal remediation under N2/H2. (b) Photographs: PIM-1 (yellow, transparent) and C-PIM-1 (glittering-grey, opaque post-carbonization). (c) XPS C 1s spectra: increased C–C content in C-PIM-1. (d) Raman spectra: presence of D and G bands in C-PIM-1. (e) Pore size distributions: comparison showing slight reduction post-carbonization, maintaining microporous structure. Reproduced from ref. 158 with permission from [Springer], copyright [2016]. | |
5.6. Hypercrosslinked polymers
Hyper crosslinked polymers (HCPs) are highly porous materials characterized by extensive crosslinking and high specific surface area. These polymers are typically synthesized through post-crosslinking of linear or lightly crosslinked precursor polymers, often using Friedel–Crafts alkylation.159 The extensive crosslinking results in a rigid, three-dimensional network with high microporosity. HCPs exhibit exceptional surface areas, often exceeding 1000 m2 g−1, contributing to their high adsorption capacity for various contaminants. The pore structure of HCPs may be tailored via adjusting the synthesis conditions and choice of monomers, allowing for the optimization of their adsorption properties for specific applications. HCPs have shown outstanding performance in removing organic pollutants, particularly aromatic compounds, from water due to their hydrophobic nature and strong π–π interactions.160 Recent research has been devoted to developing more sustainable synthesis methods for HCPs, incorporating hydrophilic groups to upgrade their selectivity, and exploring their potential in composite materials for advanced water remediation applications. The high surface area, tunable pore structure, and chemical stability make HCPs promising candidates for addressing challenging water purification needs. These polymers, characterized via their interconnected networks of aromatic structures, offer unique advantages due to their high surface area, tunable pore structure, and chemical stability. Yao et al.161 highlight hypercrosslinked polymers' exceptional adsorption capacity and selectivity towards organic contaminants like phenol and dyes. Their study underscores the potential of these materials in addressing water pollution challenges via efficiently trapping organic pollutants through adsorption mechanisms. Further elaborating on the versatility of hyper crosslinked polymers, Wolska et al.162 explore their efficacy in removing a broad spectrum of organic contaminants, including pharmaceuticals and personal care products, from water systems. This research was conducted via Wolska delves into the factors influencing adsorption, shedding light on how these polymers may be optimized for various environmental conditions and contaminant types. These research articles underscore the potential of hypercrosslinked polymers as advanced adsorbents in water remediation. Their ability to effectively remove a broad range of contaminants, from organic pollutants to heavy ions, is supported via their unique structural properties and the continuous refinement of synthesis methods. Moving forward, further research into optimizing their performance and exploring novel applications will upgrade their role in sustainable water management practices globally. Each synthetic strategy for HCPs offers unique advantages in terms of control over pore size, surface area, and chemical stability, crucial for optimizing their performance in water purification processes.163 The choice of method depends on factors like the desired pore structure, target contaminants, and production scalability, ensuring that HCPs effectively meet the diverse challenges posed via water remediation applications. Continued advancements in synthetic methodologies promise to further upgrade the efficiency and versatility of HCPs in addressing environmental concerns related to water pollution. Hypercrosslinked porous polymer materials (HCPs) are synthesized using several distinct methodologies, each tailored to achieve specific structural and functional properties.164 Post-crosslinking: post-crosslinking involves the modification of pre-existing polymer chains through strong covalent bonds to form a three-dimensional network. This method often begins with synthesizing polymer precursors that contain rigid building units capable of forming stable bonds upon cross-linking. The process upgrades the porosity and surface area of the resulting HCPs, making them effective adsorbents for various contaminants in water remediation applications.94 Direct polycondensation or one-step condensation: in this strategy, commercially available polymeric products are precursors for forming HCPs through self-condensation or condensation reactions involving small rigid molecules with bihydrophilic groups. This approach simplifies the synthesis process via directly utilizing available starting materials without requiring extensive modification or additional steps. It enables efficient production of HCPs with tailored properties suited for specific adsorption requirements. External crosslinking: external crosslinking methods encompass various strategies, like self-condensation or co-condensation of monomers containing chloromethyl and hydroxymethyl groups. The Scholl coupling reaction, which involves the oxidative coupling of aromatic compounds, is another technique used to synthesize HCPs.165 These methods facilitate the creation of interconnected networks via linking polymer chains externally, leading to upgraded stability and pore structure in the resulting materials. The knitting strategy is one example of using formaldehyde dimethyl acetal (FDA) in synthesizing hypercrosslinked porous polymer materials (HCPs). In this approach, the FDA is employed as an external crosslinker to combine simple aromatic compounds like benzene or biphenyl with rigid methylene bridges via anhydrous FeCl3 catalysis. This process forms a highly crosslinked polymer network with a tailored porous structure and specific functionalities. Using the FDA in the knitting strategy allows for the creating HCPs with upgraded properties like increased surface area, improved stability, and tunable pore architecture, making them suitable for various applications in gas storage, catalysis, and environmental remediation.166 Fig. 6 illustrates a critical process in synthesizing hyper crosslinked porous polymer materials (HCPs), specifically focusing on using Formaldehyde Dimethyl Acetal (FDA) as an external crosslinker. This synthesis method, known as the “knitting” strategy, is fundamental to creating highly porous polymer networks with tailored properties. The process begins with simple aromatic compounds like benzene or biphenyl. These compounds serve as the basic building blocks for the polymer network. The key to the process is the introduction of the FDA as an external crosslinker. FDA involves a crucial role in bridging these aromatic compounds with rigid methylene links. A critical component of this reaction is the catalyst, anhydrous FeCl3. This Lewis acid catalyst initiates crosslinking via complexing with the FDA molecules. This complexation is a pivotal step as it weakens the bond among the methoxyl group and the central carbon atom of the FDA molecule. This weakening makes the FDA more reactive and prone to further reactions.167 As a result of this catalyst-FDA interaction, intermediate carbocations are generated in the solvent. These highly reactive species are essential for the subsequent steps of polymer formation. The carbocations react with the phenyl rings of the aromatic compounds, leading to the addition of multi-methoxymethyl groups to these molecules. The process continues with these newly formed methoxymethyl groups. These groups are highly active and readily convert to methylene linkages when they encounter other phenyl rings. This step is crucial as it results in the formation of a rigid, highly crosslinked network structure. One of the most significant aspects of this synthesis method is its flexibility. Via adjusting the molar ratio among the crosslinkers (FDA) and the aromatic building blocks, researchers may fine-tune the properties of the resulting HCPs. This ability to control the synthesis allows for the customization of porous structures and surface areas, making these materials highly versatile for various applications. In recent advancements, the synthesis of hyper-crosslinked polymers (HCPs) has been facilitated through innovative methodologies involving crosslinking agents like formaldehyde dimethyl acetal (FDA), sulfur, and divinylbenzene. These agents enable one-pot synthesis, where nucleophilic aromatic monomers are interwoven directly with electrophilic crosslinking agents. This approach is economically efficient and broadly applicable, offering upgraded control over the porous structure and chemical properties of the resulting HCPs.168 In a notable development via Guo et al.,169 halogenated ionic polymers (HIPs) were synthesized using histidine and α,α′-dibromo-p-xylene (DBX) or α,α′-dichloro-p-xylene (DCX) as crosslinking agents under Lewis acid catalysis (Fig. 8a). HIPs represent a class of HCPs incorporating nucleophilic halide ions within their framework. Simultaneous quaternization and Friedel–Crafts alkylation reactions occur during synthesis, resulting in a polymer with hierarchical porosity and abundant ionic sites. These features enable efficient CO2 cycloaddition reactions under ion-free, co-catalyst-free, and solvent-free conditions, showcasing HIPs as versatile and stable materials suitable for diverse applications. Furthermore, Song et al.170 introduced three
novel poly(ionic liquid) materials composed of benzene rings, utilizing 2-phenylimidazole as a building block (Fig. 8b). These materials were synthesized via polymerizing α,α′-dichloro-p-xylene (DCX), and formaldehyde dimethyl acetal (FDA) with designed ionic liquid (IL) monomers. The self-crosslinking of the crosslinking agent contributed to a large specific surface area of the resulting hyper-crosslinked organic frameworks. Additionally, co-crosslinking among the crosslinking agent and the ionic liquid introduced active sites, upgrading the materials' capability for selective CO2 absorption and catalytic activity in CO2 cycloaddition reactions. The reported surface area of 763 m2 g−1 underscores the high performance of these porous materials in CO2 capture and utilization applications.171 Fig. 8b likely depicts this process schematically, showing the progression from individual aromatic compounds and FDA molecules to the final crosslinked network. It may consist of representations of the Lewis acid as catalyst, intermediate stages of the reaction, and the final porous structure of the HCP. This synthesis method is precious in the fields of energy and environmental sciences. The resulting HCPs, with their high porosity and large surface areas, are outstanding candidates for applications like gas storage, catalysis, and water purification. The ability to tailor the properties of these materials makes them adaptable to a broad range of specific requirements in these fields.
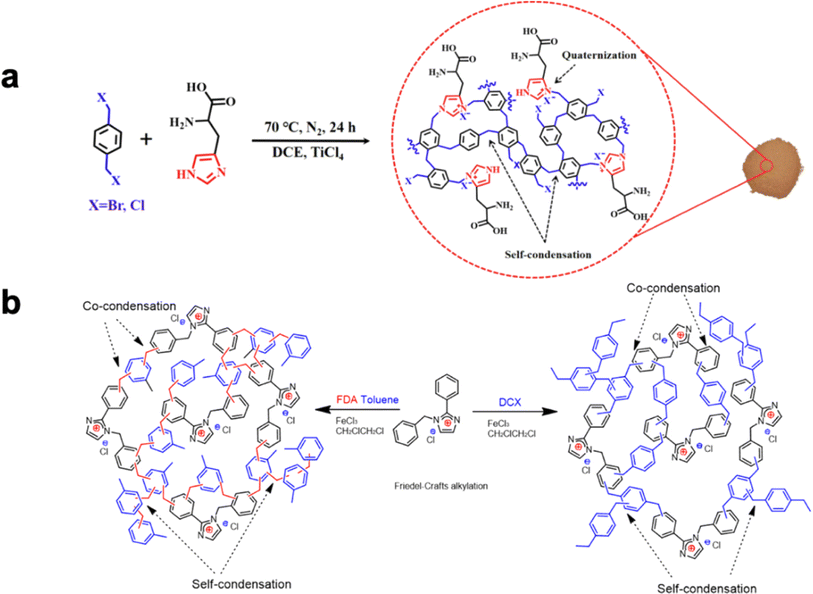 |
| Fig. 8 (a) Illustrates HIP-X-His synthesis (where X is either Br or Cl). The reaction starts with two reactants: a dihalogenated benzene compound and an amino acid derivative containing an imidazole ring. These react under specific conditions (70 °C, N2 atmosphere, for 24 hours) in the presence of DCE (dichloroethane) and TiCl4 (titanium tetrachloride) as catalysts. The product forms through self-condensation and quaternization reactions, resulting in a complex, porous structure represented via the circular diagram on the right copyright and permission. Reproduced from ref. 169 with permission from [Elsevier], copyright [2023] and (b) shows the formation of two different hyper crosslinked polymers: (1) HP-[BZPhIm]Cl-Co-Tols: formed via the reaction of [BZPhIm]Cl with FDA (fluorenone diacetyl) in toluene, catalyzed via FeCl3. (2) HP-[BZPhIm]Cl-DCXs: formed via the reaction of [BZPhIm]Cl with DCX (dichloroxylene) under similar conditions, both reactions involve Friedel–Crafts alkylation, leading to highly crosslinked structures through co-condensation and self-condensation processes. The resulting polymers have complex, three-dimensional structures with multiple aromatic rings and charged imidazolium groups. Reproduced from ref. 170 with permission from [American Chemical Society], copyright [2021]. | |
5.7. Porous organic polymers
Porous organic polymers (POPs) are a broad class of materials that encompass various types of porous polymers, including covalent organic frameworks (COFs), conjugated microporous polymers (CMPs), and porous aromatic frameworks (PAFs).172 POPs are characterized via their high surface area, tunable pore size, and diverse chemical functionalities. These materials are typically synthesized through the polymerization of organic building blocks, resulting in a network structure with well-defined pores. The organic nature of POPs allows for easy functionalization and modification of their surface properties, enabling the tailoring of their adsorption characteristics for specific contaminants. POPs have shown outstanding performance in removing a broad range of pollutants from water, including heavy ions, organic dyes, and micropollutants. Their high stability in various pH conditions and resistance to degradation make them suitable for use in challenging water remediation environments. Recent research has been devoted to developing POPs with hierarchical pore structures, incorporating catalytic sites for simultaneous adsorption and degradation of pollutants, and exploring green synthesis methods using renewable precursors. The versatility and tunability of POPs make them promising candidates for advanced water purification applications.173
This article147 offers a comprehensive overview of the synthesis and application of porous organic polymers (POPs) for water capture and remediation. POPs are characterized via their exceptional properties, including high thermal stability, large specific surface area, and the ability to have their pore functionalization tuned. These characteristics make POPs particularly effective for removing various pollutants from water. The authors delve into the molecular structure of POPs, explaining how their interconnected porous networks contribute to their high adsorption capacities. They also discuss the various synthesis methods, like condensation polymerization and cross-coupling reactions, that may create POPs with specific properties tailored for water remediation applications. The article further explores the use of POPs in both adsorption and photocatalytic processes, detailing how these materials may be modified to target specific contaminants or upgrade their photocatalytic activity for the degradation of organic pollutants in water. This article174 is devoted to a specific class of POPs known as porphyrin porous organic polymers (Py-POPs) and their application in water pollution remediation. Porphyrins are organic compounds known for their unique optical and electronic properties, and when incorporated into POPs, they create materials with exceptional characteristics for water remediation. The authors offer a detailed discussion of the synthesis methods for Py-POPs, including various polymerization techniques and post-synthetic modifications. They highlight the unique properties of Py-POPs, like their large specific surface area, high porosity, and photosensitivity, making them particularly effective for removing pollutants from water. The article175 explores using Py-POPs in adsorption and photocatalytic processes, explaining how the porphyrin units may act as adsorption sites and photocatalytic centers. The authors also discuss the potential of Py-POPs in advanced oxidation processes for degrading recalcitrant organic pollutants in water. This comprehensive review article176 examines the use of polymer-based composites in wastewater remediation, encompassing a broader range of materials beyond just POPs. The authors discuss the removal of various pollutants, including dyes, heavy ion ions, and pharmaceuticals, using different types of polymer-based composites. They explain how these composites may be designed to combine the benefits of polymers (like processability and chemical stability) with the functional properties of other materials (like nanoparticles or activated carbon). The article highlights the advantages of polymer-based composites, like their improved adsorption and removal characteristics, recyclability, and cost-effectiveness. The authors offer detailed discussions of the synthesis methods for these composites, including in situ polymerization, blending, and grafting techniques. They also explore the mechanisms of pollutant removal, discussing how different composite structures may upgrade adsorption capacity, facilitate photocatalytic degradation, or enable selective ion exchange. The review concludes via addressing the challenges and future prospects of polymer-based composites in wastewater remediation, emphasizing their potential for large-scale applications and the need for further research in areas like composite durability and regeneration. Porous aromatic frameworks (PAFs) are a class of highly versatile porous organic polymers that have shown great potential in water remediation applications.177 These materials exhibit exceptional properties, like high surface area, tunable pore structure, and outstanding chemical and thermal stability, making them effective adsorbents for removing a broad range of organic pollutants, including dyes, pharmaceuticals, and micropollutants. Additionally, the conjugated structure and light-harvesting capabilities of PAFs enable their use as photocatalysts for degrading organic contaminants under light irradiation. Furthermore, PAF-based membranes have demonstrated high selectivity and permeability towards removing heavy ions and organic pollutants, highlighting their potential in membrane filtration processes. The ability to incorporate PAFs into composite materials and functionalize them further upgrades their performance and selectivity, making them a promising solution for addressing complex water remediation challenges. While scaling up the production and improving the cost-effectiveness of PAF synthesis remain challenging, the extensive research in this field suggests that these porous organic polymers hold great promise for developing sustainable and efficient water purification technologies. Conjugated microporous polymers (CMPs) are a unique porous organic material class that combines extended π-conjugation with a microporous structure. These polymers are typically synthesized via polymerizing multifunctional monomers, resulting in a rigid, three-dimensional network with interconnected pores. The extended π-conjugation in CMPs offers them interesting optical and electronic properties and adsorption capabilities.178 CMPs exhibit high surface areas and tunable pore sizes, making them effective adsorbents for various water contaminants. The conjugated structure of CMPs allows for strong interactions with aromatic pollutants through π–π stacking, making them particularly effective in removing organic dyes and aromatic compounds from water. CMPs may be easily functionalized to introduce specific binding sites or to upgrade their hydrophilicity for better performance in aqueous environments. Recent research has been devoted to developing CMPs with improved stability in water, exploring their potential in photocatalytic water remediation processes, and creating CMP-based composite materials for upgraded adsorption performance.179 The microporosity and extended π-conjugation make CMPs promising materials for advanced water purification applications, especially for removing recalcitrant organic pollutants.
5.8. Porous coordination polymers
Porous coordination polymers (PCPs), also known as ion-organic frameworks (MOFs), are a class of crystalline materials composed of ion ions or clusters coordinated to organic ligands to form one-, two-, or three-dimensional structures.180 PCPs are characterized via their exceptionally high surface areas, tunable pore sizes, and diverse chemical functionalities. The modular nature of PCPs allows for precise control of their pore structure and surface chemistry by selecting ion nodes and organic linkers. This tunability makes PCPs highly versatile adsorbents for water purification, capable of targeting a broad range of contaminants. PCPs have shown outstanding performance in removing heavy ions, organic pollutants, and gases from water. Their crystalline nature also allows for detailed structural characterization, enabling a better understanding of the adsorption mechanisms. Recent research has been devoted to developing water-stable PCPs, as many early PCPs were moisture-sensitive. Attempts have been made to create hierarchical pore structures in PCPs, combining micro-, meso-, and macropores to upgrade mass transfer and adsorption kinetics. The integration of PCPs into composite materials and membranes has also been explored to leverage their unique properties in advanced water remediation technologies. Porous Coordination Polymers (PCPs) and Ion-Organic Frameworks (MOFs) have demonstrated exceptional adsorption capabilities and chemical stability in water remediation applications. The β-cyclodextrin-based porous polymer was reported via Wang et al.181 showcased remarkable efficiency, removing 83% of organic micropollutants within just one minute of contact time. Its adsorption capacity exceeded 200 mg g−1 for many pollutants and maintained performance through at least five regeneration cycles. This high adsorption capacity and rapid kinetics outperform many traditional adsorbents, highlighting the potential of PCPs in water purification. Similarly, the zirconium-based MOF introduced via Lin et al.182 exhibited outstanding chemical stability, retaining its crystalline structure after 24 hours in boiling water and remaining stable in aqueous solutions with pH ranging from 1 to 11. This stability is crucial for practical applications in diverse water remediation scenarios. The MOF also demonstrated a significant adsorption capacity of 74 mg g−1 for chromate anions, showcasing its potential for removing specific inorganic pollutants. These findings underscore the versatility and robustness of PCPs and MOFs in addressing various water hazard challenges. The ability of PCPs and MOFs to selectively adsorb specific pollutants represents a significant advancement in water remediation technology. Li et al. 183 reported MOFs with high selectivity for particular contaminants, like UiO-66-(COOH)2, which showed a remarkable distribution coefficient (Kd) of 5.3 × 105 mL g−1 for U(VI), and MIL-101-SO3H, with a Kd of 9.0 × 104 mL g−1 for Hg2+. This selectivity allows for targeted removal of hazardous substances, even in complex water matrices. In heavy ion removal,184 Hess et al.185 designed MOFs capable of capturing trace amounts of toxic ions. Their MOF-808 completely removed Cd2+ and Hg2+ to levels below the detection limit of 0.1 ppb, while HKUST-1 removed 99% of Pb2+ from a 1 ppm solution. These results demonstrate the potential of MOFs to address one of the most persistent and dangerous forms of water pollution – heavy ion hazard. The high selectivity and efficiency in heavy ion removal position PCPs and MOFs as promising materials for specialized water remediation applications, particularly in industrial wastewater remediation and remediation of contaminated water sources. Beyond adsorption, some PCPs and MOFs exhibit catalytic properties that enable the degradation of organic pollutants, adding another dimension to their water remediation capabilities. Andrade, Pedro HM, et al.186 reviewed the catalytic degradation potential of various MOFs, revealing impressive results. Ti-MOFs, for instance, demonstrated 90% degradation of rhodamine B under visible light in just 80 minutes, while Fe-MOFs achieved 98% removal of bisphenol A through Fenton-like reactions in 60 minutes. This multifunctionality – combining adsorption with catalytic degradation – offers the potential for more comprehensive and efficient water remediation processes. The ability to remove and break down harmful organic compounds addresses a critical challenge in water purification, particularly for persistent organic pollutants that resist conventional remediation methods. Moreover, the use of visible light in some of these catalytic processes suggests the possibility of developing sustainable, solar-driven water remediation technologies. The multifunctional nature of these materials opens up new avenues for integrated water remediation systems that may tackle a broad range of pollutants simultaneously, potentially reducing the complexity and cost of water purification processes. Despite the promising laboratory results, the transition of PCPs and MOFs to large-scale water remediation applications faces several challenges. Most studies to date have been conducted at a laboratory scale, and significant research is needed to scale up both the synthesis of these materials and their application in real-world water remediation systems. The scalability issue encompasses not only the production of the materials but also the design of appropriate engineering systems for their effective use in water remediation plants. Additionally, while some PCPs and MOFs use relatively inexpensive materials, others rely on costly components, potentially limiting their economic viability for widespread adoption.187 Developing cost-effective synthesis methods and exploring more abundant, less expensive materials are crucial to making these technologies competitive with existing water remediation solutions. Furthermore, PCPs and MOFs must demonstrate clear advantages over current technologies to gain acceptance in the water remediation industry, which often favors well-established, proven methods. Addressing these challenges requires interdisciplinary collaboration among materials scientists, chemical engineers, and water remediation professionals to bridge the gap among laboratory discoveries and practical applications. The long-term stability of PCPs and MOFs under real water remediation conditions remains a critical area for further research. While many of these materials have shown outstanding stability in laboratory tests, their performance over extended periods in complex, real-world water systems needs thorough investigation. Factors like fouling, chemical degradation, and mechanical stress in continuous flow systems could impact their long-term efficiency and durability. Additionally, the potential environmental impact of these materials must be carefully assessed. Studies on the possible release of ion ions or organic linkers from PCPs and MOFs during water remediation are necessary to ensure their safety and prevent unintended consequences. The environmental fate of these materials after their useful life, including disposal or recycling options, also requires consideration. As research progresses, it will be crucial to develop standardized testing protocols and safety guidelines for using PCPs and MOFs in water remediation. This will ensure their effectiveness and safety and build confidence among regulators and end-users, establishing the route for their integration into mainstream water remediation technologies. The continued exploration of these aspects will be vital in realizing the full potential of PCPs and MOFs for sustainable and efficient water purification.
5.9. Aromatic polystyrenic polymers
Aromatic polystyrenic polymers are a class of adsorbents based on the polystyrene backbone, which is modified or crosslinked to upgrade its adsorption properties.188 These polymers are characterized via their aromatic rings, which offer strong π–π interactions with organic contaminants, making them particularly effective in removing aromatic compounds from water. The most common form of aromatic polystyrenic adsorbents is crosslinked polystyrene, often in beads or resins. These materials may be further functionalized with various groups to upgrade their selectivity or adsorption capacity for specific contaminants. Aromatic polystyrenic polymers, including phenols, dyes, and pharmaceutical compounds, have effectively removed organic pollutants from water. Their hydrophobic nature makes them particularly suitable for removing non-polar organic contaminants. These polymers are also broadly used in ion exchange applications when functionalized with appropriate groups.189 Recent research has been devoted to developing more sustainable synthesis methods for aromatic polystyrenic polymers, exploring their use in composite materials, and upgrading their regeneration capabilities for improved reusability in water remediation applications. Combining chemical stability, ease of modification, and strong affinity for organic pollutants makes aromatic polystyrenic polymers valuable tools in water purification. The study on grafted aromatic polystyrenic polymers for wastewater remediation reveals significant variations in performance across different polymers and wastewater types.190 AP-g-PDMA (dimethylacrylamide grafted polystyrene) consistently performs well, achieving the highest removal rates for most wastewater types. For instance, in treating iron ore wastewater, AP-g-PDMA achieves a remarkable 93.5% removal rate at pH 6.5, outperforming other polymers like AP-g-PAM (89.3%) and St-g-PAM (86.4%). This trend continues across various wastewater types, with AP-g-PDMA showing exceptional results for kaolin wastewater (93.3% removal) and municipal wastewater (90% removal). The data indicates that dimethyl acrylamide grafting significantly upgrades the polymer's effectiveness in contaminant removal. However, it's noteworthy that all tested polymers perform strongly in treating coal wastewater, with removal rates ranging from 88.2% to 90.9%. This suggests that while AP-g-PDMA is generally more effective, the choice of the polymer may be optimized based on the specific wastewater characteristics and remediation requirements. The study underscores the critical role of pH in optimizing the performance of grafted aromatic polystyrenic polymers in wastewater remediation. The data reveals that the optimal pH for remediation varies significantly depending on the wastewater type. The optimal pH for iron ore and silica wastewater is 6.5, while kaolin wastewater remediation is most effective at pH 7.6. Coal wastewater shows optimal remediation at pH 7.5, and municipal wastewater at pH 6.8. Interestingly, AP-g-PAA performs outstandingly in treating textile wastewater at a higher pH of 8.2, achieving a remarkable 98.0% removal rate.190 This pH dependency highlights the importance of careful pH adjustment in wastewater remediation processes using these polymers. It suggests a preliminary pH assessment and adjustment step in practical applications could significantly upgrade remediation efficiency. The varying optimal pH levels also indicate that these polymers might have different mechanisms of action or interact differently with contaminants under various pH conditions, opening avenues for further research into the underlying chemical and physical processes involved in contaminant removal.191 The study offers valuable insights into the specialized applications of certain grafted aromatic polystyrenic polymers, particularly AP-g-PAA (acrylic acid grafted polystyrene). This polymer demonstrates exceptional performance in treating specific types of industrial wastewater. AP-g-PAA achieves an impressive 98.0% removal rate for textile wastewater at pH 8.2, significantly outperforming other polymers in this application. It also shows strong performance in treating mining industries' wastewater (87.31% removal), paper mill effluent (82.1% removal), and mine process water (81.8% removal). These results highlight the potential of AP-g-PAA as a specialized remediation solution for certain industrial wastewaters, particularly those from textile and mining industries. On the other hand, AP-g-PDMA emerges as the most versatile polymer, consistently showing high performance across various wastewater types. Its effectiveness in treating a broad range of wastewaters, from iron ore to municipal, suggests that it could be a valuable general-purpose flocculant for diverse wastewater remediation applications. This versatility could be particularly beneficial in remediation plants dealing with multiple wastewater streams or in situations where the wastewater composition varies over time. Despite the overall strong performance of grafted aromatic polystyrenic polymers, the study reveals certain challenges and areas for improvement. One notable observation is the relatively lower effectiveness of all tested polymers in treating paper mill wastewater.192 Removal rates for this type of wastewater range from 69.6% to 72.5%, significantly lower than the rates achieved for other wastewater types. This indicates a potential area for further research and development, possibly exploring new grafting techniques or polymer compositions specifically tailored to the unique contaminants in paper mill effluents. Another challenge is the varying performance of polymers in treating silica wastewater. While AP-g-PDMA and St-g-PDMA show good performance (85.8% and 83.0% removal, respectively), AP-g-PAM and St-g-PAM struggle with this wastewater type, achieving only 43.4% and 42.2% removal rates. This stark difference in performance for silica wastewater remediation suggests that the choice of grafting monomer (dimethylacrylamide vs. acrylamide) involves crucial in determining the polymer's effectiveness for certain contaminants. Further investigation into the interaction among these polymers and silica particles could lead to improvements in polymer design for specific wastewater remediation applications. This study157 offers comprehensive data on grafted aromatic polystyrenic polymers opens up several avenues for future research and practical applications in wastewater remediation. One key area for further investigation is the development of hybrid or composite polymers that combine the strengths of different grafting monomers. For instance, a polymer incorporating dimethyl acrylamide and acrylic acid could offer upgraded performance across a broader range of wastewater types and contaminants. Additionally, research into the molecular-level interactions among these polymers and various contaminants could offer insights for designing even more effective and targeted flocculants. From a practical standpoint, the study's findings underscore the importance of tailoring wastewater remediation solutions to specific effluent characteristics. Remediation plant operators and environmental engineers may use this data to select the most appropriate polymer for their specific wastewater streams, potentially improving remediation efficiency and reducing costs.193 Furthermore, the strong pH dependency observed in the study suggests that implementing advanced pH control systems in remediation plants could significantly upgrade the performance of these polymeric flocculants. As environmental regulations become increasingly stringent, developing and optimizing such high-performance, versatile flocculants will involve crucial in ensuring efficient and cost-effective wastewater remediation across various industries.
5.10. Aromatic halogenated polystyrene
Aromatic halogenated polystyrene is a specialized class of polystyrenic polymers that incorporates halogen atoms (typically chlorine or bromine) into the aromatic rings of the polymer structure. This halogenation process significantly alters the properties of the polymer, upgrading its adsorption capabilities for certain contaminants.194 The presence of halogen atoms increases the electron-withdrawing character of the aromatic rings, which may strengthen π–π interactions with certain organic pollutants. Aromatic halogenated polystyrenes often exhibit improved thermal and chemical stability compared to their non-halogenated counterparts, making them suitable for use in more challenging water remediation conditions. These polymers have shown particular effectiveness in removing halogenated organic compounds from water, like chlorinated pesticides and polychlorinated biphenyls (PCBs). The halogenation also increases the hydrophobicity of the polymer, upgrading its affinity for non-polar organic contaminants. Recent research has been devoted to optimizing the degree of halogenation to balance adsorption performance with environmental considerations, exploring the use of these polymers in composite materials, and developing more sustainable synthesis methods. While effective, the use of halogenated polymers raises some environmental concerns, prompting research into alternative materials with similar performance but reduced environmental impact. Aromatic halogenated polystyrene has emerged as a promising material for water remediation due to its unique chemical properties and high efficiency in removing contaminants. Recent research has been devoted to its application in removing polystyrene nanoplastics from water through various remediation processes. Filtration processes, particularly using sand and granular activated carbon (GAC) filters, have shown significant efficacy in removing these nanoplastics. Without coagulation, these filters achieve an overall nanoplastic removal efficiency of 88.1%, demonstrating their effectiveness even without additional chemical processes. However, introducing coagulation processes greatly upgrades the removal efficiency, increasing the global removal rate to an impressive 99.4%. This improvement is attributed to the ability of coagulants to aggregate nanoplastics, making them easier to filter out. The effectiveness of sand filtration, in particular, is significantly boosted in the presence of coagulants, with removal efficiency increasing from 54.3% to 99.2%. These findings highlight the potential of combining filtration and coagulation techniques to address the growing concern about nanoplastic hazards in water sources.195 Remediating adsorbable organic halogens (AOX) is another critical application of aromatic halogenated polystyrene in water remediation. Activated carbon, known for its high surface area and porous structure, is broadly used for the adsorptive removal of AOX from water. This method is particularly effective in treating chemical wastewater and reducing the presence of harmful halogens. Advanced remediation processes have been developed to further upgrade AOX removal, like the sequential use of Trametes versicolor and UV/TiO2/Ru(x)Se(y). This approach combines biological and photocatalytic methods to achieve higher removal efficiencies, especially in treating wastewater from paper production processes. Sequential remediation showcases a significant reduction in AOX levels, demonstrating the potential of integrating multiple remediation techniques. The effectiveness of these advanced processes in removing AOX highlights the importance of continued research and development in this area to address increasingly complex water hazard issues. Modifying polystyrene to upgrade its adsorption capabilities has opened new avenues for water remediation, particularly in removing phenolic compounds. Sodium lignosulfonate-modified polystyrene has been investigated for its ability to remove phenol from wastewater, and promising results have been shown. This modification significantly upgrades the adsorption capacity of polystyrene, making it more effective in capturing phenolic compounds. Modified polystyrene offers a sustainable and efficient solution for treating phenol-contaminated water, addressing a common industrial pollution problem. Additionally, research has explored activated carbon regeneration using phenolic-degrading fungi, like Scedosporium apiospermum. This approach allows for repeated use of activated carbon, reducing water remediation processes' overall cost and environmental impact. The ability to regenerate and reuse adsorbents is crucial for developing sustainable water remediation practices, making this area of research particularly valuable for long-term environmental solutions.196 Understanding polystyrene's chemical structure and properties is crucial for its effective application in water remediation. Polystyrene is a synthetic aromatic polymer made from monomer styrene with a chemical formula (C8H8)n. Its structure is a long hydrocarbon chain with a phenyl group attached to every other carbon atom. This unique structure contributes to its versatility and effectiveness in various applications, including water remediation. The tacticity of polystyrene, which refers to the relative stereochemistry of adjacent chiral centers in the polymer chain, may significantly influence its properties and behavior in water remediation processes. Atactic polystyrene, where the phenyl groups are randomly distributed along the polymer chain, is the most common form used in water remediation applications due to its amorphous nature and good solubility in organic solvents.197 The ability to modify polystyrene, like through halogenation or the addition of hydrophilic groups, further upgrades its adsorption capabilities and specificity for certain contaminants, making it a highly adaptable material for diverse water remediation needs. The research on aromatic halogenated polystyrene for water remediation reveals promising results and highlights several areas for future investigation. The high removal efficiencies achieved through coagulation and filtration processes, the effective adsorption of AOX via activated carbon, and the upgraded phenol removal using modified polystyrene demonstrate the versatility and effectiveness of this material. Future research should optimize these processes for large-scale applications and explore new modifications to upgrade specificity and efficiency. Additionally, the development of more sustainable and cost-effective regeneration methods for adsorbents will be crucial for the long-term viability of these remediation processes. As water hazards become increasingly complex, with arising pollutants posing new challenges, the continued exploration of aromatic halogenated polystyrene and its derivatives will be vital in advancing water remediation technologies. Via integrating insights from chemical engineering, materials science, and environmental studies, researchers may further improve the effectiveness and sustainability of water remediation solutions, ultimately contributing to better water quality and environmental protection globally.
5.11. Aliphatic methacrylate polymers
Aliphatic methacrylate polymers are a class of synthetic polymers based on methacrylate monomers with aliphatic (non-aromatic) side chains. These polymers are characterized via their flexibility, hydrophobicity, and ability to be easily functionalized.198 In water remediation applications, aliphatic methacrylate polymers are often used as resins or beads, either as homopolymers or copolymers with other monomers. The aliphatic nature of these polymers offers good chemical stability and resistance to degradation in various water remediation conditions. Aliphatic methacrylate polymers may be tailored to target specific contaminants via incorporating hydrophilic groups into their structure or via adjusting the length and branching of their aliphatic side chains. These polymers have effectively removed organic pollutants, particularly non-polar compounds, from water. They are also commonly used as precursors for ion exchange resins when functionalized with appropriate groups. Recent research has been devoted to developing methacrylate-based polymers with improved hydrophilicity for better performance in aqueous environments, exploring their use in composite materials, and upgrading their regeneration capabilities. The versatility and ease of modification of aliphatic methacrylate polymers make them valuable materials for a broad range of water purification applications.199
5.12. Polymers based hydrogel
Polymers with porous structures encompass a broad range of materials designed to have a high internal surface area and interconnected pore network. These polymers may be synthetic or natural and are characterized via their ability to remove contaminants efficiently through adsorption and size exclusion mechanisms. The porous structure of these polymers may be tailored to target specific pollutants via controlling the pore size distribution, ranging from micropores (<2 nm) to mesopores (2–50 nm) and macropores (>50 nm). Common examples consist of polyurethane foams, porous polyethylene, and various hydrogels. These materials are particularly effective in removing organic contaminants like alcohols, aldehydes, ketones, phenols, furans, and acids from aqueous solutions. The high internal surface area offered via the porous structure allows for efficient adsorption, while the interconnected pore network facilitates the rapid mass transfer of contaminants. Recent research has been devoted to developing hierarchical porous structures combining different pore sizes to optimize adsorption capacity and kinetics.200 Attempts have been made to incorporate hydrophilic groups within the porous structure to upgrade selectivity for specific contaminants. The versatility and tunability of porous polymers make them valuable materials for addressing a broad range of water purification challenges. Fig. 9 in the document illustrates the adsorbent–adsorbate interaction mechanisms for the hazard removal of wastewater via cellulose-based hydrogels (CBHs). The figure shows the various interactions among the hydrogel's hydrophilic groups and the contaminants present in the wastewater. (1) Electrostatic interactions: electrostatic interactions are crucial in the adsorption of ions onto cellulose-based hydrogels (CBHs). When the pH of the solution is higher than the point of zero charge (pH > pHPZC) of the CBH, the surface becomes negatively charged. This leads to the attraction and adsorption of positively charged cations from the aqueous solution to the negatively charged hydrophilic groups, like carboxyl (–COO−) or hydroxyl (–OH) groups, on the CBH surface. Conversely, at lower pH values (pH < pHPZC), the surface may become positively charged, facilitating the adsorption of negatively charged anions through electrostatic interactions. (2) Ion exchange: ion exchange involves the substitution of ions adsorbed on the CBH surface with ions of similar charge from the surrounding aqueous solution. In CBHs, cation exchange typically occurs when the pH is lower than the pHPZC, allowing positively charged ion ions to exchange with other cations in the solution. Anion exchange, on the other hand, happens when the pH is higher than the pHPZC, enabling CBHs to adsorb and exchange anions like nitrate (NO3−) or phosphate (PO43−). (3) Hydrogen bonding: hydrogen bonding occurs among polar hydrophilic groups on CBHs, like –OH and –COOH, and electronegative atoms (e.g., oxygen or nitrogen) in the adsorbate molecules. These interactions significantly remove polar pollutants from wastewater via CBHs, as they upgrade adsorption through specific and directional bonding. (4) Hydrophobic interactions: hydrophobic interactions involve non-polar regions of CBHs interacting with hydrophobic pollutants in the aqueous solution. This interaction is driven via the tendency of hydrophobic groups to minimize contact with water molecules via associating with similar non-polar regions on adsorbates. CBHs with hydrophobic domains may effectively remove organic compounds like oils, dyes, and certain pesticides from wastewater. (5) Coordination interactions: coordination interactions occur when ion ions in the wastewater form coordination bonds with lone pair electrons on oxygen or nitrogen atoms within the hydrophilic groups of CBHs, like carboxyl (–COOH) or amino (–NH2) groups. These interactions are specific and selective, allowing CBHs to effectively adsorb heavy ion ions like copper (Cu2+), lead (Pb2+), and cadmium (Cd2+) from contaminated water sources. These mechanisms collectively illustrate the complex nature of pollutant removal via CBHs, highlighting their versatility and effectiveness in wastewater remediation applications.
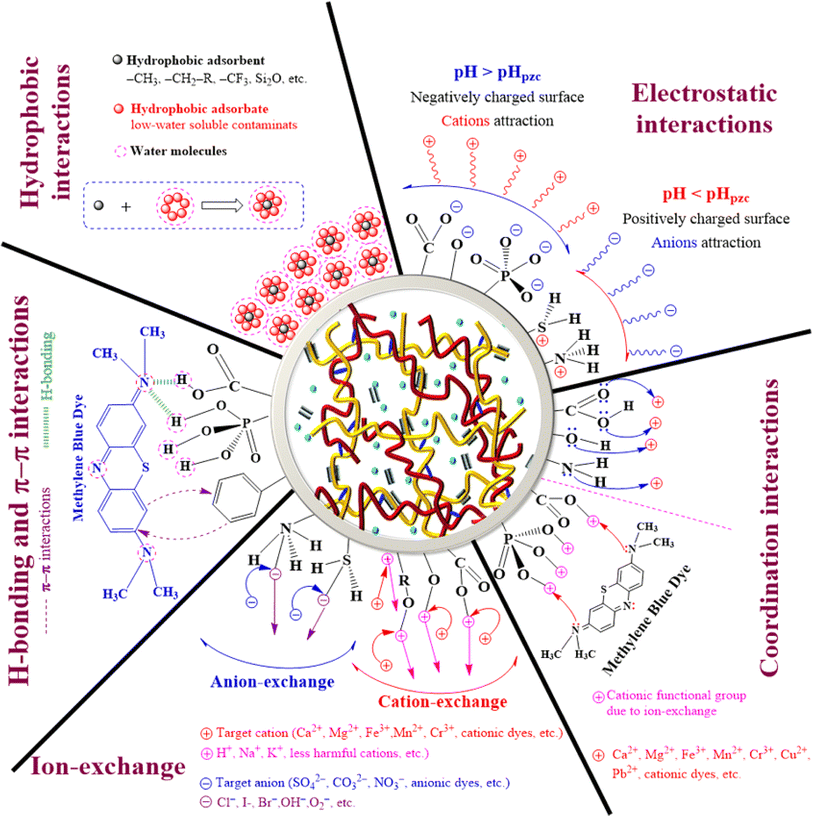 |
| Fig. 9 Adsorbent–adsorbate interaction mechanisms for the hazard removal of wastewater via cellulose-based hydrogels (CBHs). Reproduced from ref. 201 with permission from [MDPI], copyright [2021]. | |
5.13. Molecularly imprinted polymers
Molecularly imprinted polymers (MIPs) have emerged as a promising technology for water remediation, offering high specificity and efficiency in removing trace pollutants. The core of this technology lies in creating polymer structures that may selectively recognize and bind to specific target molecules.202 This process, known as molecular imprinting, involves pre-arranging functional monomers around a template molecule, polymerizing the monomer–template complex with cross-linkers, and removing the template to leave behind complementary binding sites. The resulting structure is analogous to the lock-and-key or antibody-to-antigen principles, ensuring high specificity for target molecules. This approach allows for developing tailored solutions to address specific water hazard issues, making MIPs particularly valuable in scenarios where traditional water remediation methods may fall short. Creating these highly specific binding sites within a polymer matrix opens new possibilities for targeted pollutant removal, potentially revolutionizing water remediation processes across various industries and environmental applications. The effectiveness of MIPs in water remediation is largely attributed to the non-covalent interactions that drive their formation and function. These interactions, including electrostatic forces, ion chelation, hydrogen bonding, and van der Waals forces, involve a crucial role in the self-assembly of functional monomers and templates. These interactions not only guide the formation of the polymer matrix but also facilitate the subsequent binding of target molecules, ensuring the high specificity of MIPs.203 To upgrade the performance of MIPs in aqueous environments, researchers have developed advanced fabrication techniques like Pickering emulsion polymerization. This method produces water-compatible imprinted polymers with greater stability than those made with conventional organic surfactants. The Pickering emulsion technique improves the mass transfer of template molecules during removal and rebinding processes, resulting in more efficient and robust MIPs for water remediation applications. This advancement addresses one of the key challenges in applying MIPs to water remediation: ensuring their effectiveness in purely aqueous environments. MIPs have demonstrated remarkable performance in water remediation, particularly in the selective removal of trace pollutants. The specific binding sites within the polymer matrix allow for targeted removal of contaminants, significantly improving the overall effectiveness of water remediation processes. This selective removal is especially beneficial for detecting and eliminating low-concentration pollutants that are often challenging to address with conventional methods. One of the most successful applications of MIPs in water remediation has been in solid-phase extraction (SPE). When used in SPE, MIPs effectively isolate and remove specific compounds from aqueous samples, upgrading the purification process via selectively binding to target analytes. This selectivity reduces the presence of unwanted substances in the treated water, resulting in a higher-quality output.204 The application of MIPs in SPE has shown particular promise in analytical separations, allowing for more precise control over water remediation processes and potentially enabling the recovery of valuable substances from wastewater streams. To maximize the effectiveness of MIPs in water remediation, researchers have devoted themselves to optimizing these polymers for purely aqueous environments. This optimization involves the synthesis of specialized functional monomers that facilitate the efficient formation of the template-functional monomer matrix in water-based applications. These tailored monomers ensure that MIPs maintain their high performance and specificity even when fully immersed in water, addressing a critical requirement for practical water remediation applications. The versatility of MIPs further upgrades their value in water remediation scenarios. Beyond their use in analytical separations and pollutant removal, MIPs have shown potential in applications like drug delivery and chemical sensors. This versatility makes them a valuable tool in addressing a broad range of water pollution issues, from industrial wastewater remediation to the purification of drinking water supplies. The ability to adapt MIP technology to various water remediation challenges demonstrates its potential as a flexible and powerful solution in the continuing attempt to improve water quality globally. The development and application of molecularly imprinted polymers in water remediation represent a significant advancement in environmental technology.205 Their high specificity and efficiency in removing trace pollutants and the ability to tailor these polymers for specific contaminants offer a promising approach to addressing complex water pollution issues. As research in this field progresses, future directions may consist of further optimization of MIP fabrication techniques to upgrade their performance in diverse aqueous environments, exploration of new template molecules to target arising pollutants, and the development of large-scale, cost-effective production methods for widespread implementation. Integrating MIP technology with other water remediation processes could also lead to more comprehensive and efficient water purification systems. The potential for MIPs to selectively remove and potentially recover valuable substances from wastewater also opens up possibilities for resource recovery in water remediation processes. In conclusion, molecularly imprinted polymers offer a highly specific and efficient approach to water remediation, with the potential to significantly improve water quality across various applications. As research continues to advance this technology, MIPs are poised to involve an increasingly important role in addressing global water pollution challenges and contributing to sustainable water management practices.
6. Synthesis and modification of polymeric adsorbents
Polymeric adsorbents are synthesized using various polymerization techniques, each offering distinct advantages in control over molecular structure, functionality, and physical properties. The primary polymerization methods include:
6.1. Free radical polymerization
This is the most common method for synthesizing polymeric adsorbents. It involves the polymerization of vinyl monomers initiated via free radicals. Free radical polymerization may be initiated thermally, photochemically, or using chemical initiators like benzoyl peroxide.206 This technique allows for the synthesizing of various polymers with different hydrophilic groups via copolymerizing different monomers. However, controlling the molecular weight distribution and polymer architecture may be challenging.
6.2. Emulsion polymerization
This technique produces polymers with high molecular weights and uniform particle sizes. It involves the polymerization of monomers in an aqueous emulsion containing surfactants. Emulsion polymerization is beneficial for creating polymeric adsorbents with high surface areas and porosity, which are essential for adsorption applications. Additionally, the process may be easily scaled up, making it suitable for industrial production.207
6.3. Suspension polymerization
In this method, the monomer is dispersed in a continuous phase (usually water) and polymerized to form spherical polymer beads. Suspension polymerization is advantageous for producing bead-shaped polymeric adsorbents with controlled particle sizes, particularly useful in packed bed adsorption columns.208 The process also allows for the incorporation of functional monomers to tailor the adsorbent properties.
6.4. Solution polymerization
This method involves the polymerization of monomers in a solvent, producing polymers dissolved in the solvent. Solution polymerization is suitable for synthesizing high-purity polymers and easily incorporates hydrophilic groups. However, subsequent solvent removal may be costly and environmentally challenging.209
7. Chemical and physical modifications
Modifying polymeric adsorbents is essential to upgrade their adsorption properties, like selectivity, capacity, and stability. These modifications may be chemical or physical:
7.1. Chemical modifications
Introducing hydrophilic groups like amines, carboxyls, or sulfonic acids onto the polymer backbone upgrades the adsorbent's affinity for specific pollutants. For example, aminated polymers may effectively capture heavy ions through chelation. The study210 carried different chemical modifications of the p(AAm-co-AAc) hydrogel, which have varying effects on the adsorption capacity of the hydrogels for ion ions. The study conducted chemical modifications to functionalize the bare hydrogel with thiol (–SH), amino (–NH2), or carboxyl (–COOH) groups, resulting in the development of six functional hydrogels (compound 1 to compound 6). Fig. 10 illustrates a reaction involving modifying a specific weight of p(AAm-co-AAc) hydrogel sample (compound 0). The process begins by allowing the hydrogel sample to swell in water and heating at 90 °C in a 0.5 M NaOH solution. Subsequently, the hydrogel sample reacts with 1 M HCl to produce a modified product labeled as compound (2). This reaction scheme demonstrates the alkaline hydrolysis of the amino groups in the hydrogel, leading to the formation of the modified product. These hydrophilic groups selectively bind and remove target ions from the solution. Among the modified hydrogels, the NaOH-treated hydrogel (compound 2) outperformed all other modified ones in the removal of Cu2+, Ba2+, and Sr2+ ions, with maximum capacities of 13.67, 36.4, and 27.31 mg g−1, respectively. The chemical modification with sodium hydroxide significantly upgraded the adsorption capacity of the hydrogel for the targeted ion ions. Conversely, the modification strategy of the hydrogel with thionyl chloride (compound 3) reduced the adsorption capability for Cu2+ ion removal. Additionally, the chemically modified compound 6 hydrogel exhibited slightly lower adsorption uptake for Ba2+ and Sr2+ ions than other modified hydrogels.
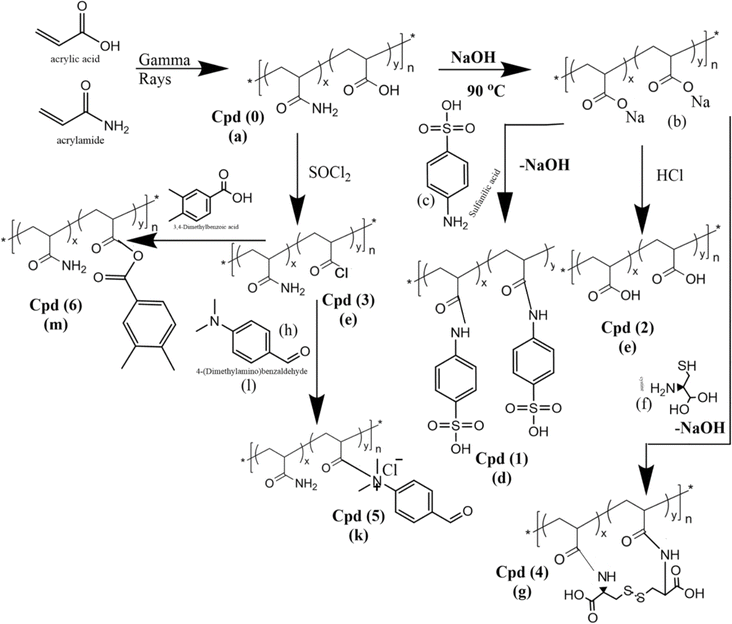 |
| Fig. 10 Proposed mechanism and reaction process involves modifying a p(AAm-co-AAc) hydrogel sample (Cpd 0) to yield the modified products. Reproduced from ref. 210 with permission from [Springer], copyright [2024]. | |
7.2. Physical modifications
Physical methods like templating and phase inversion may increase the porosity of polymeric adsorbents. High porosity increases the surface area for adsorption and improves mass transfer rates. Polymers may also be used to modify adsorbents like clay and minerals. For example, Cu2+-exchanged hectorite films showed vertical shifts in the position of the platelets on the surface.211 This was believed to be due to intercalated aniline polymerizing in the interlayer region, with no evidence of benzene polymerization on the surface. After exposure to aniline vapor, the structure on the hectorite surface consists of small polymer bundles. The diameter of these bundles ranged from 300–3000 Å, similar to the structure seen on electropolymerized polyaniline films. Aniline polymerized on the surface of hectorite films at 180 °C revealed a structure identical to undoped N-methylpyrrolidinone (NMP) cast polyaniline films, with polymer bundles averaging 300 Å in dimension. Preparing PANI/bentonite involves several key steps to ensure the effective synthesis of polyaniline (PANI) on the bentonite surface through plasma-induced polymerization. Initially, 2.0 g of bentonite undergoes pre-remediation via air plasma for 30 minutes. During this step, the plasma is generated with a power of 100 W, and air is flowed at a rate of 10 mL min−1 over the bentonite material. This plasma remediation is crucial as it modifies the surface properties of the bentonite, upgrading its reactivity and promoting the subsequent polymerization process. After the plasma remediation, the activated bentonite is immersed in a solution containing 50 mL of aniline. The reaction occurs at a controlled temperature of 60 °C for 3 hours. During this period, the aniline monomer undergoes polymerization initiated via the activated sites on the bentonite surface. The polymerization process results in the deposition and growth of PANI chains directly onto the bentonite particles, forming a composite material known as PANI/bentonite. This synthesis method is advantageous because it leverages plasma remediation to activate the bentonite surface, facilitating better adhesion and polymerization of PANI. The resulting PANI/bentonite composite material exhibits upgraded properties suitable for various applications, including adsorption, catalysis, and sensor technologies.212 The modification of bentonite with polymerized aniline upgrades the efficiency of the bentonite adsorbent via altering its exchange sites, as evidenced via changes in the 2θ angle of the X-ray diffraction (XRD) pattern, specifically the d-spacing of the (001) plane. Bentonite, a natural clay mineral, typically exhibits a characteristic XRD peak at around 2θ = 6°, corresponding to a d-spacing of approximately 1.5 nm for the (001) plane in its pristine state. Several notable changes occur in the XRD pattern upon modification with polymerized aniline (PANI). Firstly, there is a shift in the position of the (001) peak towards higher or lower 2θ angles, indicating an increase or decrease in the d-spacing. This shift suggests intercalation or partial exfoliation of the bentonite layers due to the incorporation of PANI chains among the clay layers. The intercalation of PANI may expand the interlayer spacing of bentonite, allowing for easier access of adsorbates to the exchange sites within the clay structure. Moreover, the intensity and broadening of the (001) peak may also change, reflecting variations in the crystallinity and stacking of the bentonite layers post-modification also upgrades the adsorbent efficiency via modifying the exchange sites within the bentonite structure. These changes indicate structural alterations induced via the interaction among PANI and bentonite, potentially upgrading the availability and accessibility of exchange sites on the modified adsorbent material. Fig. 11 illustrates the structure of bentonite clay and the effect of aniline polymerization induced via gamma irradiation within its layers. The structure of bentonite consists of repeating layers, each composed of two tetrahedral sheets sandwiching an octahedral sheet. The layers are separated via interlayers, which may contain adsorbed cations and water molecules. The atomic compositions consist of silicon and oxygen atoms from the tetrahedral sheets, aluminum, magnesium, and hydroxide groups form the octahedral sheet, and various planes of oxygen and hydroxyl groups are present throughout the structure. The aniline polymerization is effective in the d-spacing (d001) of the bentonite structure, which increases from 15.7 Å to 16.1 Å after aniline polymerization. This increase suggests that the polyaniline (PANI) forms within the interlayer spaces of the bentonite. In challenge, Cs ion has a relatively large hydrated radius, which limits its interaction with conventional adsorbents. Traditional methods for Cs removal consist of ion exchange, precipitation, and membrane filtration, but these approaches often have limitations like high cost, secondary waste generation, and efficiency issues. Recent advancements have been devoted to developing novel sorbents like zeolites, layered double hydroxides (LDHs), ion-organic frameworks (MOFs), and composites tailored to upgrade Cs adsorption capacity and selectivity. These materials typically feature high surface areas, tunable pore structures, and specific hydrophilic groups optimized for Cs binding through ion exchange or chemisorption mechanisms. For example, certain zeolites exhibit outstanding Cs selectivity due to their negatively charged frameworks and cage-like structures, which facilitate Cs ion capture. Additionally, MOFs offer precise control over pore size and ion node chemistry, enabling tailored interactions with Cs ions. Such advancements highlight the potential of advanced sorbents in addressing the challenges of Cs removal from contaminated water sources, establishing the route for more effective and sustainable water remediation solutions. In this context, Maksoud et al.213 investigated the sorption of cesium ions using two prepared organoclay adsorbents synthesized via gamma rays, namely bentonite/polyaniline (OC1) and bentonite/polyaniline/ilmenite (OC2). The process included the penetration of polyaniline molecules and ilmenite into the bentonite host material at montmorillonite sites. This modification aimed to upgrade the adsorption capacity of the bentonite-based sorbents for cesium ions in aqueous solutions. The modification process altered the interlayer structure of the bentonite, making it more suitable for ion adsorption in wastewater remediation applications. The study is devoted to exploring the parameters affecting the adsorption process, like initial Cs concentration, pH, contact time, temperature, and sorbent dosage, to understand the efficiency of the synthesized sorbents in hazard removal and cesium removal from aqueous solutions. The optimum results of the study showed that the synthesized organoclay adsorbents, OC1 and OC2, exhibited high stability toward removing cesium ions from aqueous solutions. The maximum adsorption capacity of Cs+ was found to be approximately 24.7 mg g−1 for OC1 and 34.4 mg g−1 for OC2. The adsorption process followed the Langmuir model, indicating a favorable adsorption mechanism. Additionally, the desorption process using 0.1 M NaOH and 0.1 M HCl showed efficient desorption rates, reaching up to 97% for OC1 and 50% for OC2 after multiple adsorption stages. Due to the superior selectivity, stability, and compatibility of the synthesized organoclay adsorbents OC1 and OC2, the study successfully achieved high efficiency in removing cesium ions from aqueous solutions. Modifying bentonite using gamma radiation and incorporating polyaniline and ilmenite improved adsorption capacities, making the sorbents suitable for hazard-removal applications. The optimum results highlighted the effectiveness of the sorbents in cesium removal, showcasing their potential for addressing challenges associated with hazardous ion hazards in water sources. Fig. 11 illustrates the structure of bentonite clay and the effects of aniline polymerization within its layers.213 The bentonite structure consists of repeating layers, each composed of two tetrahedral sheets (silicon and oxygen) sandwiching an octahedral sheet (aluminum, magnesium, and hydroxide). The layers are separated via interlayers containing adsorbed cations and water. The image highlights the bentonite structure's acid sites (Si plane and O plane) and base sites (Al, Mg plane and O, OH plane). Importantly, the exchange sites in bentonite, also where aniline polymerization occurs, are indicated. The polymerization of aniline inside the bentonite structure leads to an increase in the d-spacing (d001) from 15.7 Å to 16.1 Å, suggesting successful intercalation of polyaniline into the interlayer spaces while maintaining the overall layered structure. This composite combines the properties of bentonite (high surface area, ion exchange capacity).
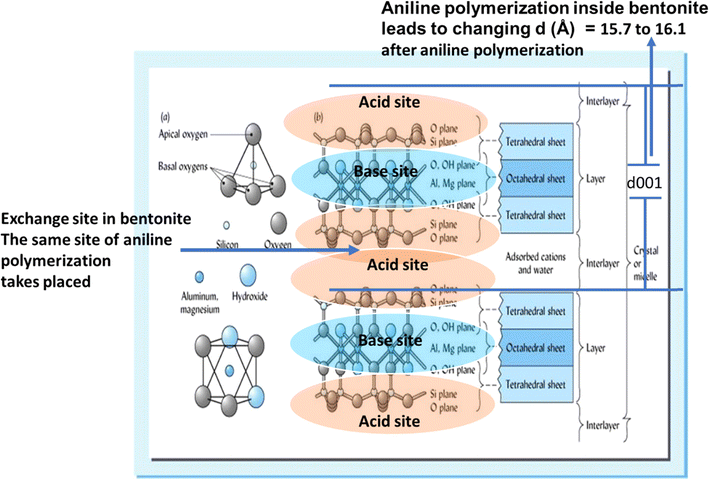 |
| Fig. 11 Aniline polymerization inside bentonite: structural changes and reaction sites. Effect on d-spacing and identification of acid–base sites in the clay layers. | |
8. Adsorption characteristics of polymeric adsorbents
Polymeric adsorbents, including porous organic polymers (POPs), ion-organic frameworks (MOFs), and synthetic polymers, exhibit diverse and crucial adsorption characteristics that underpin their efficacy in water remediation applications.214 Understanding these characteristics is essential for optimizing their use and harnessing their full potential in addressing water hazard challenges. Raman spectroscopy is a powerful analytical technique that may offer valuable insights into the adsorption characteristics of polymeric adsorbents. It is used for surface analysis, identifying adsorption mechanisms, monitoring adsorption kinetics, characterizing polymer structure and composition, evaluating thermal stability, and conducting complementary analysis with other techniques. For instance, Raman spectroscopy may analyze surface properties, like the presence and distribution of hydrophilic groups, cross-linking degree, and adsorbent particle morphology, which are crucial for understanding adsorption mechanisms and optimizing adsorbent performance. Researchers may identify specific interactions among the adsorbent and target pollutants via comparing Raman spectra before and after adsorption, like hydrogen bonds and π–π interactions. Additionally, Raman spectroscopy helps monitor the adsorption process over time, providing insights into kinetics, equilibrium capacity, and desorption behavior. It also offers detailed information about polymer chemical structure and composition, including hydrophilic groups and polymerization degree. Raman spectroscopy is essential for evaluating the thermal stability of polymeric adsorbents and is important for water remediation applications under varying temperatures. Combining Raman spectroscopy with other techniques, like IR spectroscopy, X-ray diffraction, and scanning electron microscopy, offers a comprehensive understanding of adsorption characteristics. For example, the article215 used Raman spectroscopy and gravimetric sorption measurements to determine the adsorption capacity and selectivity of translucent functionalized porous materials, specifically silica ionogels. Raman spectroscopy was employed to characterize the adsorption capacity and selectivity of the materials at high pressures. The method involved conducting line measurements in the ionogels and bulk phase using a confocal Raman microscope. The density of the fluids in the adsorbent was compared to their density in the vapor phase, and substance-specific peaks were analyzed to determine adsorption capacity. On the other hand, gravimetric sorption measurements were conducted independently using a magnetic-suspension balance to validate the results obtained from Raman spectroscopy. The measurements were carried out at various pressures with an equimolar (hydrogen + carbon dioxide) gas mixture and pure constituents. The net adsorption capacity was calculated based on the mass of the sample and the adsorbed fluid, and the results were compared with the Raman spectroscopy data to ensure consistency and accuracy in determining the sorption properties of the ionogels. Fig. 12 depicts a schematic of the experimental setup used for adsorption measurements of an equimolar gas mixture on an ionogel utilizing a confocal Raman microscope. The figure shows how spectra were recorded along a single axis at equidistant intervals of 5 μm in both the bulk phase and inside the ionogel particle. The measurements were conducted at a specific height (z = 200 μm), corresponding to approximately half the height of the investigated particles. The structure of a mesopore is highlighted in the inset of the figure, although it is noted that the details in the schematic are not true to scale. The experimental setup allowed for the visualization and analysis of the adsorption process within the ionogel at high pressures, providing valuable insights into the adsorption capacity and selectivity of the material. Fig. 12 presents a schematic of an innovative experimental setup for measuring gas adsorption on ionogels (IGs) using confocal Raman microscopy. The custom-made high-pressure optical cell contains an IG particle on the left and a bulk gas phase on the right, filled with an equimolar hydrogen and carbon dioxide mixture. The confocal Raman microscope, positioned above the cell, conducts line measurements at 5 μm intervals along a single axis from inside the IG particle to the bulk gas phase. All measurements are taken at z = 200 μm, approximately half the height of the IG particles. This setup allows for a direct comparison of gas density within the adsorbent to that in the vapour phase, enabling the determination of adsorption capacity as a function of pressure. The inset highlights the mesoporous structure of the IG. While not drawn to scale, the schematic illustrates a method that combines the chemical specificity of Raman spectroscopy with high spatial resolution, providing detailed insights into adsorption phenomena in complex materials. The system operates at a constant temperature of 293.15 K and may handle pressures from 1 to 50 bar, though these control mechanisms are not explicitly shown in the Fig. 12.
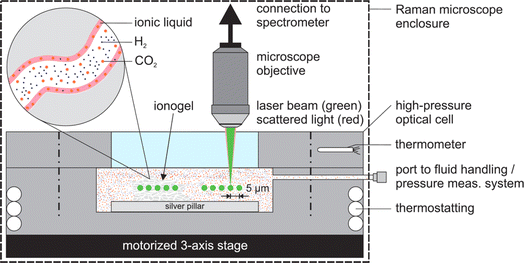 |
| Fig. 12 Schematic of the experimental setup for adsorption measurements of an equimolar gas mixture on an IG utilizing a confocal Raman microscope. Spectra are recorded at 5 μm intervals along a single axis in the bulk phase (right) and inside the IG particle (left) at z = 200 μm, approximately half the particle height. The inset highlights the mesoporous structure, noting the schematic is not to scale. Reproduced from ref. 215 with permission from [Wiley], copyright [2022]. | |
Molecularly Imprinted Polymers (MIPs) and Raman spectroscopy efficiently separate and enrich melamine from whole milk samples.216 MIPs act as a sorbent in Solid Phase Extraction (SPE) to clean up the samples rapidly, allowing for the accurate detection and quantification of melamine using Surface-Upgraded Raman Spectroscopy (SERS). The MIPs are designed to selectively recognize and bind melamine, upgrading the sensitivity and specificity of the detection method. Surface-Upgraded Raman Spectroscopy (SERS) improves the detection of melamine in milk by significantly increasing the detection method's sensitivity and specificity.217 Here's how SERS upgrades the detection of melamine in milk: (1) Signal upgradement: SERS significantly upgrades the Raman scattering signals of molecules adsorbed on the surface of noble ion nanostructures, like silver or gold. This upgrade may amplify the Raman signals via several orders of magnitude, making it possible to detect trace levels of analytes like melamine. (2) Increased sensitivity: the upgraded Raman signals in SERS allow for the detection of analytes at very low concentrations, even down to single molecule levels. This high sensitivity is crucial for detecting contaminants like melamine in food samples at regulatory levels. (3) Selective detection: the specific interaction among the analyte (melamine) and the SERS-active substrate (silver dendrite in this study) leads to selective detection. This specificity ensures that the detected signal is primarily from the target analyte, reducing interference from other components in the sample. (4) Fast analysis: SERS enables rapid analysis of samples, as demonstrated in the study where the full analysis time to determine melamine in whole milk was less than 20 minutes. This speed is essential for high-throughput detection required in food industry settings. (5) Quantitative analysis: SERS may offer quantitative information about the concentration of melamine in milk samples. The study showed a good linear relationship among the intensity of the melamine SERS band and melamine concentration, allowing for accurate quantification. In detecting melamine in whole milk samples, Raman spectroscopy demonstrated its capability through efficient separation and enrichment of melamine using Molecularly Imprinted Polymers (MIPs).218 It achieved accurate detection and quantification within a specific range, showcasing high sensitivity with a limit of detection (LOD) of 0.012 mmol L−1 and a rapid analysis time of less than 20 minutes, highlighting its effectiveness for sensitive and swift melamine detection in food samples.
8.1. Adsorption capacity and kinetics
The adsorption capacity of polymeric adsorbents refers to their ability to capture and retain contaminants from water. The polymer matrix influences. This capacity via surface area, pore size distribution, and hydrophilic groups. Hydrophobic interactions are pivotal in influencing the adsorption capacity of polymeric adsorbents due to their nature as weak, non-covalent forces that occur among non-polar molecules or surfaces in aqueous environments. These interactions arise from water molecules' tendency to minimize contact with hydrophobic surfaces, resulting in the aggregation of hydrophobic groups and the exclusion of water molecules. Hydrophobic interactions play a critical role in polymeric adsorbents by upgrading their adsorption capacity, particularly for non-polar organic pollutants. Hydrophilic groups or aromatic rings within the polymeric structure attract and bind hydrophobic adsorbates through these interactions.219 The strength of hydrophobic interactions is modulated via factors like ionic strength and temperature, where higher ionic strength increases hydrophobic interactions but diminishes electrostatic interactions, optimizing adsorption capacity. Temperature also affects these interactions, with lower temperatures favoring enthalpic contributions that drive the adsorption process. Thus, understanding and controlling hydrophobic interactions are crucial for optimizing the performance of polymeric adsorbents in diverse water remediation applications.
Adsorption kinetics is pivotal in comprehending the temporal aspects and mechanisms governing adsorption processes, which is crucial for optimizing various industrial and environmental applications. It encompasses the study of reaction rates, sorption mechanisms, mass transfer phenomena, diffusion processes, and surface reactions occurring during the adsorption of substances from solution onto solid surfaces.220 The process typically unfolds in three distinct phases: external mass transfer, where molecules move across the boundary layer surrounding the adsorbent; internal diffusion, involving the penetration of molecules into the porous structure of the adsorbent material (Fig. 13); and finally, the establishment of physical or chemical bonds at active sites within the adsorbent's pores. Key kinetic models employed in adsorption studies consist of Langergren's pseudo-first-order model, which assumes adsorption occurs via surface sites and follows an exponential decrease in the number of vacant sites with time. Conversely, Ho's pseudo-second-order model suggests adsorption proceeds through chemisorption, involving the sharing or exchange of electrons among adsorbent and adsorbate, reflecting a more realistic adsorption mechanism over time. Mechanism determination models like the interfacial diffusion and particle diffusion models further elucidate how external and internal mass transport processes influence overall adsorption kinetics, aiding in developing efficient adsorption processes tailored to specific applications.
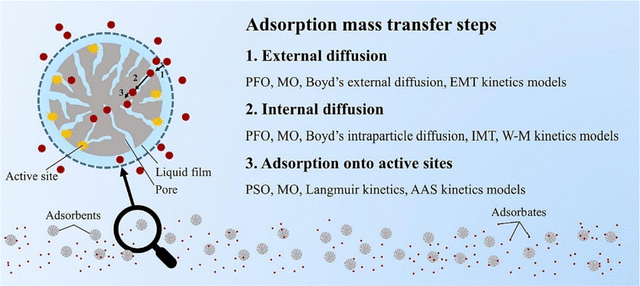 |
| Fig. 13 The general steps of mass transfer in adsorption kinetics models. | |
On the other hand, the kinetics of adsorption describe the rate at which contaminants are adsorbed onto the polymeric surface.221 This parameter is critical for assessing the efficiency and practicality of using polymeric adsorbents in water remediation processes. By understanding the adsorption capacity and kinetics, researchers and engineers can tailor the design and operational parameters of water remediation systems to achieve optimal contaminant removal while maximizing resource utilization.
For example, the article222 discusses gallic acid's adsorption capacity and kinetics onto polymeric adsorbents with amine hydrophilic groups. The polymeric adsorbents studied in the research are characterized via their weight and volume exchange capacities, which determine their ability to adsorb gallic acid. The adsorption capacity and kinetics analysis of the polymeric adsorbents used in the study222 are as follows: (1) sample P115, hydrophilic groups: xCH2CHNHCOCH2CH2CH2CH2y, volume weight: 0.03256 g mL−1, exchange capacity: 0.187 meq mL−1/5.735 meq g−1. (2) Sample P-BA-2, hydrophilic groups: CH2CH2CH2CH2CHCHC
OCHCH2xNCHCH3CH3NNHy, volume weight: 0.0534 g mL−1, exchange capacity: 0.510 meq mL−1/9.55 meq g−1. (3) Sample P-HH, hydrophilic groups: yCH2CH2CHCHNH2C
ONHCHCH2xCHCH2CNHNHNHz, volume weight: 0.2284 g mL−1, exchange capacity: 0.523 meq mL−1/1.304 meq g−1. (4) Sample P1, hydrophilic groups: N + CH2CHCH2Cl–CONH2, volume weight: 0.4814 g mL−1, exchange capacity: 2.70 meq mL−1/1.30 meq g−1. The adsorption capacities of the polymeric adsorbents were found to vary, with P115 showing the highest efficiency, followed via P1, P-Ba-2, and PHH.
Regarding kinetics, the study observed that the sorption capacity of gallic acid increased with time, reaching equilibrium within 2 hours for both concentrations tested. The adsorption kinetics were modeled using the Lagergren pseudo-first-order equation to understand the sorption rate.223 The results indicated that the sorption rate increased with the concentration of gallic acid. The polymeric adsorbents with amine hydrophilic groups may be called ion exchangers. These ion exchangers are designed to have specific hydrophilic groups that may interact with and adsorb target molecules, like gallic acid in this study. The hydrophilic groups, like primary, secondary, and tertiary amine groups, modify the chemical composition of the adsorbent, upgrading its adsorption behavior towards organic compounds like polyphenols. In the context of characterizing ion exchangers, it is important to understand the difference among milliequivalents per milliliter (meq mL−1) and milligrams per milliliter (mg mL−1) as these units are used to describe different properties of the materials. The choice among meq mL−1 and mg mL−1 should be guided via the specific requirements of the application and the type of information that is most useful for the purpose at hand. For ion exchange and detailed chemical analysis, meq mL−1 is more informative, while mg mL−1 is typically more practical and simpler for general concentration measurements. Here's a compares milliequivalents per milliliter (meq mL−1) and milligrams per milliliter (mg mL−1) in Table 4. Milliequivalents per milliliter (meq mL−1) is preferred in contexts where understanding ion exchange capacity, chemical reactions, and ion balance is crucial, like in ion exchange applications, clinical and medical settings, and environmental science. Conversely, milligrams per milliliter (mg mL−1) is preferred for general concentration measurements, ease of use, and situations where straightforward mass per volume information is needed, like in food science, pharmaceuticals, and certain regulatory contexts.224
Table 4 Illustrates the differences in measurement units and their practical applications in various scientific and industrial contexts, highlighting their respective strengths in ion exchange studies and general concentration measurements
Attribute |
meq mL−1 (milliequivalents per milliliter) |
mg mL−1 (milligrams per milliliter) |
Definition |
A measure of the amount of a substance based on its chemical equivalent |
A measure of the mass of a substance in a given volume of solution |
Usage |
They are used to describe the exchange capacity of ion exchangers |
They were used to describe the concentration of a substance in a solution |
Considers valence |
Yes, it takes into account the valence (charge) of ions |
No, it only considers the mass of the substance |
Relevant for |
Determining ion exchange capacity |
Quantifying the amount of substance present or adsorbed |
Calculation basis |
Amount of ion exchange sites available per unit volume |
Mass of the substance per unit volume |
Direct application |
Performance and efficiency of ion exchangers |
Concentration of a solute in a solution |
Conversion requirement |
Requires ion valence and molar mass knowledge for conversion to mg mL−1 |
Direct measurement of mass per volume; conversion to meq mL−1 requires molar mass and valence information |
Ion exchange applications |
Preferred: it accounts for the valence of ions and measures ion exchange capacity |
Less preferred: it does not offer direct information on ion exchange capacity |
Chemical reactions |
Preferred: when dealing with reactions, ion exchange or chemical equivalence is important |
Less preferred: unless the mass concentration is specifically required |
General concentration measurement |
Less preferred: more complex to interpret for general solute concentration |
Preferred: straightforward for measuring the amount of a substance in a solution |
Environmental science |
Preferred: useful for understanding pollutant ion exchange and remediation processes |
Less preferred: offers mass concentration but not ion exchange capacity |
Ease of use |
Less preferred: requires additional information (valence, molar mass) for interpretation |
Preferred: easier to measure and understand directly as mass per volume |
Regulatory and standardization |
Preferred: in fields where ion exchange capacity is a standard measure (e.g., water remediation) |
Less preferred: unless specified for contaminant mass concentration regulations |
8.2. Selectivity and specificity
The selectivity and specificity of polymeric adsorbents are pivotal in their ability to target and capture specific contaminants from water. Selectivity refers to the preferential adsorption of certain contaminants over others, while specificity relates to the ability of the adsorbent to recognize and selectively bind to particular molecules or ions.225 The polymeric materials' chemical composition, surface functionalization, and pore structure often govern these characteristics. Via upgrading the selectivity and specificity of adsorbents, it becomes possible to tailor their performance to remove specific pollutants, thereby improving the overall efficiency and cost-effectiveness of water remediation processes. In the context of adsorption mechanisms in polymers, selectivity refers to the ability of the hydrogel to preferentially adsorb certain ions or molecules over others based on specific properties. Factors like ionic radius, electronegativity, ionization potential, and hydration radius are crucial in determining a hydrogel's selectivity for particular ions. For example, hydrophilic groups like –COOH in nanocomposite polymers may exhibit higher adsorption selectivity for Pb2+ ions compared to other ion ions like Ni2+, Cd2+, Cu2+, and Zn2+ due to differences in their ionic properties.
Additionally, the size of the ionic radius may influence the binding capacity, with larger ions having a superior binding capacity to the polymers. Specificity, on the other hand, refers to the ability of the polymers to accurately and precisely target and adsorb a particular ion or molecule of interest while minimizing interactions with other ions or molecules present in the solution. This specificity may be achieved via designing the polymers hydrophilic groups and structure to upgrade the affinity for the desired ion or molecule. Modifying the polymer structure may increase specificity towards certain contaminants, making the polymers more effective in selective adsorption processes.
The ability of polymeric adsorbents to be regenerated and reused is a critical aspect of their sustainability and economic viability in water remediation applications. Regeneration involves removing adsorbed contaminants from the polymeric matrix, restoring its adsorption capacity, and preparing it for subsequent use. Reusability refers to the ability of the adsorbent to maintain its adsorption performance over multiple cycles of operation.226 Both regeneration and reusability are influenced via factors like the stability of the polymeric matrix, the nature of the adsorption–desorption process, and the potential for structural and chemical degradation. Maximizing the regeneration and reusability of polymeric adsorbents is essential for minimizing waste generation, reducing operational costs, and promoting sustainable use in water remediation systems.
Regeneration and reusability are critical aspects of the practical application of polymers in wastewater remediation processes. Regeneration refers to restoring a polymer's adsorption capacity after it has been saturated with contaminants. This process is essential to ensure the long-term effectiveness and sustainability of the polymer as an adsorbent. Regeneration methods may vary depending on the type of contaminants and the specific properties of the polymer. Some common regeneration techniques for polymers consist of desorption using appropriate solvents or solutions, like acids, bases, or complexing agents, to release the adsorbed contaminants from the polymer. Thermal remediation, washing, and other physical or chemical methods may also regenerate the hydrogel for further use. Reusability, on the other hand, refers to the ability of the polymer to be used multiple times for adsorption without significant loss of adsorption capacity or structural integrity. The reusability of a polymer is closely related to its regeneration efficiency. A polymer that may be regenerated effectively and retains its adsorption capacity over multiple cycles is considered highly reusable.227
Attempts are being made to develop polymers with improved regeneration and reusability properties to upgrade their practical applicability in large-scale wastewater remediation processes. Researchers aim to create sustainable and cost-effective solutions for removing contaminants from wastewater using polymer-based adsorbents by optimizing regeneration methods and designing polymers with durable structures. Understanding and optimizing the adsorption capacity and kinetics, selectivity and specificity, and regeneration and reusability of polymeric adsorbents are pivotal for advancing their application in water remediation.228 These characteristics not only define the performance of polymeric adsorbents but also inform the design of efficient and sustainable water remediation processes.
9. Sustainable polymeric adsorbents for biological contamination
Sustainable polymeric adsorbents have gained attention in the field of water remediation, particularly for addressing biological contamination. These materials are derived from renewable resources and exhibit properties that make them effective in capturing and removing biological pollutants, such as bacteria, viruses, and organic contaminants. Polymeric adsorbents have shown effectiveness in capturing various pathogens, including bacteria (e.g., Escherichia coli, Salmonella), viruses, and protozoa. Their ability to selectively adsorb these contaminants can lead to significant reductions in microbial load in treated water. These adsorbents can be combined with other water treatment methods, such as biological filtration and chemical disinfection, to enhance overall efficacy. For instance, they can be used as a pre-treatment step to reduce the burden of contaminants before applying more intensive treatment processes. Sustainable polymeric adsorbents can be deployed in situ, allowing for real-time treatment of contaminated water sources. This approach is particularly beneficial in remote areas where traditional treatment facilities are unavailable. Sharma et al.229,230 conducted extensive research on Guar gum-based hydrogels, demonstrating their effectiveness in both dye adsorption and antibacterial applications. Their work on Guar gum/acrylic acid and Guar gum/itaconic acid hydrogels showed impressive dye removal rates of 70% and 87%, respectively, along with 100% antibacterial killing effectiveness. These studies highlight the versatility of natural gum-based hydrogels in environmental remediation and disinfection processes. Expanding on the use of natural gums, Sahraei and Ghaemy et al.231 developed a gum tragacanth-graphene oxide (GT-GO) hydrogel. This composite material showed remarkable adsorption capacities for heavy metal ions, specifically 132.12, 112.50, and 142.05 mg g−1 for Ag(I), Cd(II), and Pb(II), respectively. The incorporation of graphene oxide into the hydrogel structure likely contributed to its enhanced adsorption performance. Additionally, the GT-GO hydrogel exhibited antibacterial properties against S. aureus, demonstrating a multifunctional approach to water treatment. Dil and Sadeghi et al.232 took a different approach by focusing on gelatin-based hydrogels. Their porous gelatin/acrylic acid (PGE-AcA) and silver nanoparticle-doped porous gelatin-silver/acrylic acid (PGESNC-AcA) hydrogels showed promising results for copper ion removal, with adsorption rates of 65.8% and 78.7% respectively. The incorporation of silver nanoparticles not only improved adsorption performance but also enhanced antibacterial activity against both S. aureus and E. coli, showcasing the potential for synergistic effects in hydrogel design. Mohamed et al.233 explored the potential of N-quaternized chitosan/poly(acrylic acid) (NQC/PAA) hydrogels for heavy metal removal and antibacterial applications. Their material demonstrated high adsorption capacities for various metal ions, including Cd(II), Fe(III), Cu(II), Cr(III), and Ni(II), with removal percentages ranging from 44.0% to 93.3%. The NQC/PAA hydrogel also exhibited strong antibacterial properties against both Gram-positive and Gram-negative bacteria, further emphasizing the multifunctional nature of these materials. These studies collectively demonstrate the versatility and effectiveness of synthetic polymeric hydrogels as adsorbents and antibacterial agents. The ability to fine-tune properties through material selection and preparation methods allows for targeted applications in water treatment and environmental remediation. Future research in this field may focus on improving adsorption capacities, enhancing selectivity for specific pollutants, and developing more sustainable and cost-effective hydrogel formulations. Fig. 14 provides a comprehensive visual representation of several hydrogel-based adsorbents and their performance characteristics. Fig. 14 highlights different aspects of the hydrogels studied by various research groups. Sharma et al.229 presented their work on Guar gum-based hydrogels in the first part of Fig. 14a–c. The SEM images in Fig. 14a reveal the morphological changes in the hydrogel structure as it progresses from pure Guar gum to the doped Ggum-cl-poly(AcA-ipn-aniline) form. Fig. 14b displays a bar graph comparing the adsorption percentages of these different formulations, clearly showing the enhanced performance of the doped hydrogel. The antibacterial efficacy of the Guar gum/acrylic acid hydrogel is demonstrated in Fig. 14c through images of bacterial growth inhibition. The work of Sahraei and Ghaemy et al.231 on the GT-GO hydrogel is illustrated in sections Fig. 14d–f. A schematic representation of the GT-GO hydrogel preparation process is shown in Fig. 14d, providing insight into its synthesis. The removal efficiency of the hydrogel over multiple cycles is presented in Fig. 14e, demonstrating its reusability. Fig. 14f showcases the antibacterial properties of the GT-GO hydrogel through images of bacterial growth inhibition zones. Returning to the work of Sharma et al.,230 Fig. 14g–i focuses on their Guar gum/itaconic acid hydrogel. SEM images in Fig. 14g display the morphological differences between natural Guar gum and the modified hydrogel forms. The adsorption rates of these different formulations are compared in a bar graph in section Fig. 14h, while their antibacterial activity is visually represented in Fig. 14i.
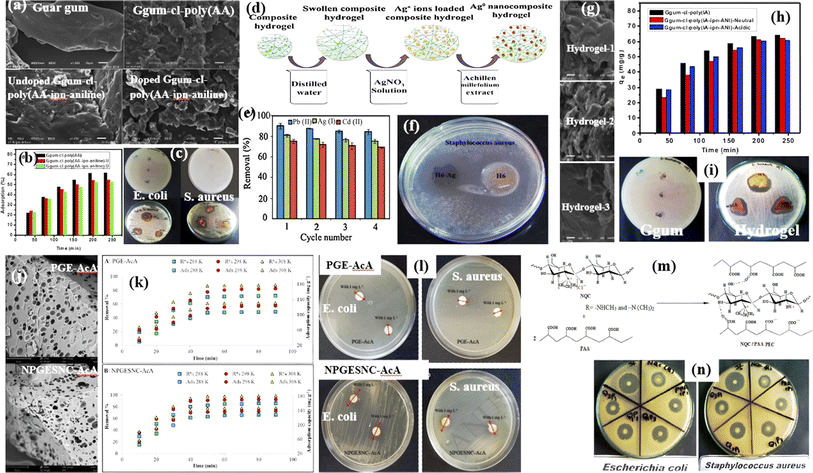 |
| Fig. 14 Comparative analysis of various hydrogel-based adsorbents. (a) SEM images and (b) adsorption percentages of Guar gum, Ggum-cl-poly(AcA), undoped Ggum-cl-poly(AcA-ipn-aniline) and doped Ggum-cl-poly(AcA-ipn-aniline). (c) Antibacterial study of Guar gum/acrylic acid hydrogel (Sharma et al., 2015).229 (d) Schematic illustration of GT-GO hydrogel preparation route. (e) Removal efficiency of GT-GO hydrogel over multiple cycles. (f) Antibacterial study of GT-GO hydrogel (Sahraei and Ghaemy et al.,231). (g) SEM images, (h) adsorption rates, and (i) antibacterial activity of Guar gum natural, Guar gum neutral, and Guar gum/itaconic acid hydrogel (Sharma et al., 2017).229 (j) Surface morphology, (k) adsorption capacity, and (l) antibacterial study of PGE-AcA and PGESNC-AcA hydrogel (Dil and Sadeghi et al.,232). (m) Adopted fabrication method of NQC/PAA hydrogel. (n) Antibacterial study of NQC/PAA hydrogel against S. aureus and E. coli (Mohamed et al.,233). Reproduced from ref. 234 with permission from [Elsevier], copyright [2021]. | |
Dil and Sadeghi et al.232 contributed the PGE-AcA and PGESNC-AcA hydrogels, which are featured in sections Fig. 14j–l. The surface morphology of these hydrogels is shown through SEM images in (j). Their adsorption capacities are compared in a bar graph in Fig. 14k, and their antibacterial efficacy is demonstrated through growth inhibition images in Fig. 14l.
Finally, Mohamed et al.233 is work on the NQC/PAA hydrogel is presented in sections Fig. 14m and n. Fig. 14m provides a schematic illustration of the NQC/PAA hydrogel fabrication method, offering insight into its preparation process. Fig. 14n visually represents the antibacterial properties of this hydrogel against S. aureus and E. coli through images of bacterial growth inhibition.
Various polymer-based adsorbents have been developed to remove water-borne pathogens effectively. For instance, Khan et al.235 on chitosan-based adsorbents further exemplifies the effectiveness of polymeric materials in removing water-borne pathogens. The study focused on the synthesis of chitosan-copper oxide (CS-CuO) composite spheres, which were evaluated for their ability to adsorb methyl orange dye while simultaneously exhibiting antibacterial properties. The CS-CuO composite demonstrated a remarkable adsorption rate of approximately 95.9% for methyl orange, alongside significant antibacterial activity against various pathogens, including Pseudomonas aeruginosa. This dual functionality highlights the potential of chitosan-based materials in addressing both dye pollution and microbial contamination in water.
Moreover, the work by Sanmugam et al.236 introduced a chitosan–ZnO–graphene oxide hybrid composite that showcased enhanced dye adsorption and antibacterial properties. The study reported that this composite could effectively remove model pollutants such as methylene blue and crystal violet while achieving high antibacterial efficacy against both Gram-positive and Gram-negative bacteria. The incorporation of zinc oxide and graphene oxide not only improved the adsorption capacity but also provided a synergistic effect that enhanced the antibacterial performance of the composite, making it a promising candidate for water purification applications.
Additionally, the research by Taghipour et al.237 on zinc metal-organic polymers (Zn-MOP) demonstrated the potential of coordination polymers in water treatment. The Zn-MOP exhibited an impressive adsorption capacity of 70.923 mg g−1 for bromocresol purple, along with effective antibacterial activity against Proteus vulgaris. This study illustrates the versatility of polymer-based materials in targeting specific pollutants while simultaneously addressing microbial threats in water sources.
These examples collectively emphasize the ongoing advancements in the field of polymeric adsorbents, showcasing their multifaceted capabilities in removing toxic pollutants and deactivating water-borne pathogens. The integration of antibacterial properties into these materials not only enhances their effectiveness in water treatment but also contributes to the development of sustainable and efficient solutions for ensuring safe drinking water. As research continues to evolve, the potential for these multifunctional adsorbents to play a critical role in addressing global water quality challenges becomes increasingly evident. Haldorai and Shim et al.238 investigated the synthesis of chitosan/MgO composites through a chemical precipitation method. The resulting composite exhibited significant antibacterial properties, particularly against common pathogens such as E. coli. The authors highlighted the synergistic effect of chitosan's biocompatibility and MgO's antibacterial characteristics, making this composite a promising candidate for water treatment applications. The study emphasized the potential of chitosan-based materials in effectively removing contaminants while simultaneously providing antimicrobial action, thus addressing both pollutant removal and pathogen deactivation in water. Kamal et al.239 focused on the development of a ZnO/chitosan coating layer for the removal of dyes and its antibacterial properties. The study demonstrated that the ZnO/Chi-MCM composite exhibited a notable adsorption capacity for methylene blue (MO) dye, with a maximum removal rate of 42.8 mg g−1. Additionally, the antibacterial activity was evaluated against E. coli, showing a significant zone of inhibition. The findings suggest that the incorporation of ZnO into chitosan not only enhances dye removal efficiency but also provides effective antibacterial action, making it suitable for wastewater treatment applications. Ahmad et al.240 explored the preparation and characterization of thiosemicarbazide-modified chitosan as an efficient adsorbent for heavy metal ions and its antibacterial properties. The modified chitosan demonstrated a remarkable 99% effectiveness in disinfecting water contaminated with various pathogens. The authors noted that the functional groups introduced during the modification process enhanced the adsorption capacity for metal ions while also providing significant antibacterial activity against Gram-positive and Gram-negative bacteria. This dual functionality positions thiosemicarbazide-modified chitosan as a valuable material for both pollutant removal and pathogen control in water treatment. Kumar et al.241 presented the synthesis of crosslinked chitosan for effective dye removal and antibacterial activity. The crosslinked chitosan exhibited a maximum removal capacity of 95% for methylene blue (MO) dye under optimized conditions. Furthermore, the antibacterial performance was assessed, revealing a strong inhibitory effect against E. coli. The study emphasized the importance of crosslinking in enhancing the structural integrity and adsorption properties of chitosan, making it a versatile material for addressing both organic dye pollution and microbial contamination in water sources. Sahraei and Ghaemy et al.231 focused on the synthesis of a gum tragacanth/graphene oxide composite hydrogel for heavy metal ion removal and antibacterial applications. The composite exhibited excellent adsorption capacity for heavy metals and demonstrated significant antibacterial activity against S. aureus and E. coli. The authors highlighted the advantages of using natural polysaccharides like gum tragacanth in combination with graphene oxide, which not only enhances the adsorption properties but also provides a biocompatible and environmentally friendly approach to water purification. This composite shows promise for practical applications in wastewater treatment. Kamal et al.239 investigated the performance of ZnO/Chi-MCM composites for the removal of organic dyes and their antibacterial properties. The results indicated that the composite achieved a maximum adsorption capacity of 42.8 mg g−1 for methylene blue (MO) dye. Additionally, the antibacterial activity was evaluated against E. coli, showing a significant zone of inhibition. The authors concluded that the incorporation of ZnO into chitosan enhances both the adsorption efficiency for dyes and the antibacterial action, making it a suitable candidate for water treatment applications targeting both organic pollutants and microbial contaminants. Saeid et al.242 developed a polyurethane foam-cadmium sulfide nanocomposite for the removal of reactive orange 122 dye and disinfection of waterborne pathogens. The study reported a removal rate of 22.7 mg g−1 for the dye, along with 100% disinfection efficiency against S. aureus and E. coli. The authors emphasized the potential of using cadmium sulfide nanoparticles within a polyurethane matrix to achieve effective pollutant removal while simultaneously providing antibacterial properties. This composite demonstrates the feasibility of integrating adsorbent materials with antimicrobial capabilities for enhanced water treatment solutions. Dhillon et al.243 focused on the synthesis of a hydrous hybrid Fe–Ca–Zr oxide nanomaterial for the removal of fluoride from water and its antibacterial activity against E. coli. The research demonstrated a fluoride removal rate of 250 mg g−1, along with a significant zone of inhibition against the bacteria. The authors highlighted the effectiveness of the hybrid nanomaterial in addressing both fluoride contamination and microbial threats, showcasing its potential for application in water purification systems that require dual functionality in pollutant removal and pathogen deactivation. Hosseinzadeh et al.244 explored the synthesis of kappa-carrageenan beads containing silver nanoparticles for dye adsorption and antibacterial applications. The composite exhibited a maximum adsorption rate of 269 mg g−1 for cationic dyes, along with strong antibacterial activity against E. coli and S. aureus. The authors noted that the release of silver ions from the nanoparticles contributed to the antibacterial properties, making this composite an effective material for both dye removal and microbial disinfection in wastewater treatment processes. Zhang et al.245 investigated silver-dispersed carbon aerogels for their adsorption and antibacterial properties. The study reported a maximum adsorption capacity of 240 mg g−1 for various organic pollutants, along with significant antibacterial activity against E. coli. The authors emphasized the advantages of using silver nanoparticles within the carbon aerogel matrix, which not only enhanced the adsorption efficiency but also provided effective microbial control. This dual functionality positions silver-dispersed carbon aerogels as promising materials for water treatment applications targeting both organic contaminants and pathogens. Fig. 15 presents a comprehensive overview of various chitosan-based composite materials and their applications in dye removal and antibacterial activities. Fig. 15a–d focuses on the chitosan-magnesium oxide (CS-MgO) composite, as studied by Haldorai and Shim et al.238 Fig. 15a provides a schematic illustration of the fabrication route for the CS-MgO composite, giving insight into its synthesis process. A transmission electron microscopy (TEM) image in Fig. 15b offers a long view of the CS-MgO composite, revealing its structural characteristics. The dye removal efficiency of the composite is presented in a graph in Fig. 15c, while its antibacterial activity is demonstrated in Fig. 15d. Fig. 15e–g showcases the work of S. B. Khan et al.235 on copper oxide-embedded chitosan (CS-CuO) composite spheres. Fig. 15e shows a graphical representation of the composite's removal of methyl orange (MO). Fig. 15f compares the removal results for different dyes using CS-CuO, highlighting its versatility in dye adsorption. Fig. 15g illustrates the antibacterial properties of CS-CuO.
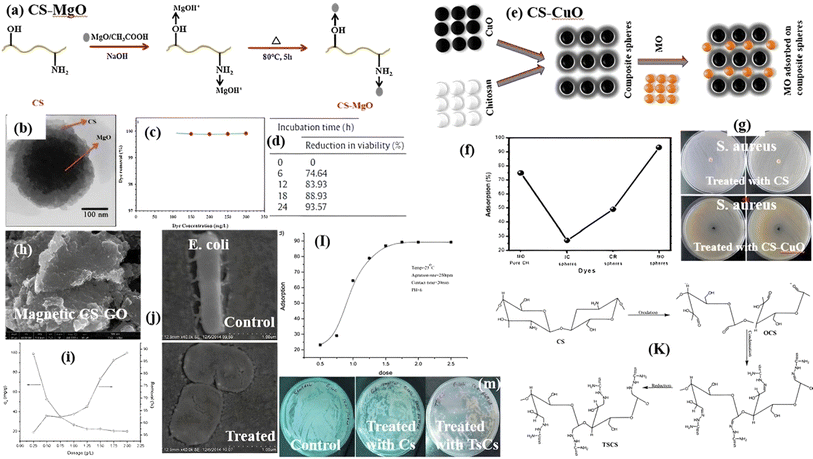 |
| Fig. 15 Chitosan-based composite materials for dye removal and antibacterial applications. (a) Schematic illustration of CS-MgO composite fabrication route. (b) TEM image showing long view of CS-MgO composite. (c) Percentage of dye removal. (d) Antibacterial activity of CS-MgO composite (Haldorai and Shim et al.,238). (e) Graphical representation of MO removal on CS-CuO composite. (f) Removal results of different dyes using CS-CuO. (g) Antibacterial study of CS-CuO (S. B. Khan et al.,235). (h) SEM images of MCGO. (i) SEM images of E. coli before and after treatment with MCGO. (j) Variation of adsorption capacity and removal percentage versus different adsorption dosages of MCGO (Jiang et al.,246). (k) Preparation route of TSCS. (l) Adsorption capacity versus dosage of TSCS. (m) Antibacterial study of TSCS (Ahmad et al.,240). Reproduced from ref. 234 with permission from [Elsevier], copyright [2021]. | |
Fig. 15h–j presents the findings of Jiang et al.246 on the magnetic chitosan-graphene oxide (MCGO) composite. Fig. 15h shows SEM images of MCGO, while Fig. 15i displays SEM images of E. coli before and after treatment with MCGO, visually demonstrating its antibacterial effect. A graph in Fig. 15j illustrates the variation of adsorption capacity and removal percentage versus different MCGO adsorption dosages Fig. 15k–m focuses on the work of Ahmad et al.240 on tripolyphosphate cross-linked chitosan (TSCS). The preparation route for TSCS is schematically represented in Fig. 15k. A graph showing the adsorption capacity versus dosage of TSCS is presented in Fig. 15l, and its antibacterial properties are demonstrated in Fig. 15m.
Polyaniline (PANI) based adsorbents have garnered attention due to their unique conductive properties, which make them suitable for environmental and antibacterial applications. PANI composites, such as PANI-Ti[IV] and PANI-ZrO2, have shown remarkable efficiency in adsorbing pollutants and combating bacterial growth. In studies by Bushra et al.247 and Masim et al.,248 these composites exhibited significant adsorption capacities and demonstrated excellent antibacterial activities against a range of bacterial strains. These materials are particularly advantageous for their dual functionality in environmental remediation and disinfection, offering an integrated approach to pollutant removal and bacterial control. Polyurethane foam-cadmium sulfide nanocomposites represent another polymeric material designed for adsorptive applications. Saeid et al.242 developed this nanocomposite specifically for the removal of reactive orange 122 dye, a common industrial pollutant. The material not only exhibited high adsorption performance but also demonstrated complete (100%) disinfection efficiency against bacteria, making it highly effective for environmental cleaning. The combination of polyurethane foam with cadmium sulfide nanoparticles enhances the material's surface area and reactivity, allowing it to function as both an adsorbent and antibacterial agent.
10. Research gaps, limitations, and challenges of polymeric adsorbents
Sustainable polymeric adsorbents are emerging as a pivotal solution in water remediation technologies. They offer the potential to significantly improve the removal of contaminants and deactivation of pathogens. Their effectiveness in addressing these critical issues is essential for safeguarding public health and maintaining environmental safety. Despite their promising capabilities, several research gaps, limitations, and challenges must be addressed to harness their potential fully. One significant research gap lies in the development of multifunctional polymeric materials. Current research primarily focuses on the adsorption properties of these materials, but there is a growing need for adsorbents that combine multiple functionalities. Integrating additional capabilities, such as catalytic activity or antimicrobial properties, could greatly enhance their performance in diverse water treatment applications. For example, polymeric adsorbents with catalytic properties could facilitate the breakdown of contaminants in situ, while those with antimicrobial properties could address microbial pathogens more effectively.249
Another important research area is the integration of polymeric adsorbents with advanced remediation technologies.250 Combining these adsorbents with techniques like membrane filtration and advanced oxidation processes could lead to more efficient and comprehensive water treatment solutions.251 This integration can address complex water quality challenges by leveraging the strengths of each technology, potentially leading to enhanced overall remediation performance.
Enhanced characterization techniques are also crucial for advancing the field. Improved methods for understanding the structure–property relationships of polymeric adsorbents are needed. Advanced modeling techniques, such as molecular dynamics simulations, could provide valuable insights into how these materials interact with contaminants at a molecular level. This knowledge could facilitate the design of more effective adsorbents tailored to specific contaminants and applications.
Despite their advantages, polymeric adsorbents face several limitations. Scalability is a major challenge, as many promising materials are developed in controlled laboratory settings but struggle to be produced on an industrial scale.252 The transition from laboratory to large-scale production often encounters obstacles related to synthesis processes and material consistency. Additionally, the high production costs associated with some advanced polymeric materials can limit their practical application in large-scale water treatment facilities, making cost-effectiveness a key concern.
Environmental stability is another limitation. Some polymeric adsorbents may degrade when exposed to harsh environmental conditions over extended periods, potentially affecting their long-term performance and sustainability. Research into improving the durability of these materials under varying environmental conditions is necessary to ensure their effectiveness over time. Moreover, the ability to regenerate and reuse polymeric adsorbents efficiently is often limited, and enhancing their reusability is crucial for developing sustainable water treatment solutions.
The challenges facing polymeric adsorbents are multifaceted. The complexity of water contaminants requires adsorbents to be designed with specificity for target pollutants while maintaining broad-spectrum efficacy. Compliance with stringent regulatory standards for water quality can also complicate the deployment of new materials. Research must align with these regulations to ensure that polymeric adsorbents meet the required standards and gain acceptance from regulatory bodies. Additionally, public acceptance and awareness play a vital role in the successful implementation of new technologies. Educating stakeholders about the benefits and safety of polymeric adsorbents is essential for their adoption in water treatment systems.
11. Conclusions
Polymeric adsorbents, particularly those based on hydrogels, biopolymers, and nanocomposites, have proven to be highly effective in adsorption-based water remediation technologies. Their ability to remove a wide range of pollutants, including heavy metals and industrial dyes, along with their capacity to deactivate water-borne pathogens, makes them critical components in sustainable water treatment solutions. By incorporating antimicrobial agents like metal nanoparticles, these materials offer dual functionality, addressing both chemical contaminants and biological hazards. The development of multifunctional polymeric adsorbents provides a promising approach for safer water purification and long-term environmental sustainability. Continued research into enhancing their adsorption efficiency and antibacterial properties will further strengthen their role in global water remediation efforts. Integrating polymeric adsorbents with other remediation technologies, like membrane filtration and advanced oxidation processes, offers synergistic benefits and improves overall remediation efficiency. As research and development in polymer chemistry, nanotechnology, and material science continue to advance, polymeric adsorbents are poised to contribute significantly to sustainable water purification technologies. The future of polymeric adsorbents in water remediation looks promising, with continuing innovations devoted to upgrading performance, selectivity, and sustainability to ensure the protection and preservation of vital water resources for future generations.
Abbreviation
MIPs | Molecularly imprinted polymers |
PANI | Polyaniline |
PAM | Polyacrylamide |
PVA | Poly(vinyl alcohol) |
EPS | Extracellular polymeric substances |
SPE | Solid-phase extraction |
NMPs | Nano-magnetic polymers |
POPs | Porous organic polymers |
FDA | Formaldehyde dimethyl acetal |
DCX | Dichloroxylene |
COFs | Covalent organic frameworks |
CMPs | Conjugated microporous polymers |
PAFs | Porous aromatic frameworks |
HCP | Hyper-crosslinked polymers |
HIP | Halogenated ionic polymers |
PE | Polyethylene |
PAAc | Polyacrylic acid |
FTIR | Fourier transform infrared spectroscopy |
SEM | Scanning electron microscopy |
TGA | Thermogravimetric analysis |
PGA | Polyglutamic acid |
meq/mL | Milliequivalents per milliliter |
mg/mL | Milligrams per milliliter |
IL | Ionic liquid |
MOFs | Metal-organic frameworks |
PIM | Polymer of intrinsic microporosity |
MWCNT | Multi-walled carbon nanotubes |
HEMA | Hydroxyethyl methacrylate |
VOCs | Volatile organic compounds |
PEG | Polyethylene glycol |
PVP | Polyvinylpyrrolidone |
SAW | Surface acoustic wave |
HPSW | Horizontally polarized shear wave |
STW | Surface transverse wave |
QCM | Quartz crystal microbalance |
CMUT | Capacitive micromachined ultrasound transducer |
IRT | Infrared thermography |
DPN | Dip-pen nanolithography |
BSA | Bovine serum albumin |
SAM | Self-assembled monolayer |
DNA | Deoxyribonucleic acid |
RNA | Ribonucleic acid |
IgG | Immunoglobulin G |
E. coli | Escherichia coli |
ECL | Electrochemiluminescence |
ATP | Adenosine triphosphate |
SPR | Surface plasmon resonance |
NPs | Nanoparticles |
MI | Magnetoimpedance |
HPLC | High-performance liquid chromatography |
LC-MS | Liquid chromatography-mass spectrometry |
ICP-MS | Inductively coupled plasma mass spectrometry |
XRF | X-ray fluorescence |
HRTEM | High-resolution transmission electron microscopy |
QD | Quantum dot |
FRET | Förster resonance energy transfer |
EDX | Energy dispersive X-ray spectroscopy |
HPLC-MS | High-performance liquid chromatography mass spectrometry |
Raman | Raman spectroscopy |
EPR | Electron paramagnetic resonance |
CE | Capillary electrophoresis |
NTA | Nanoparticle tracking analysis |
SPM | Scanning probe microscopy |
IR | Infrared spectroscopy |
UV-Vis | Ultraviolet-visible spectroscopy |
GC-MS | Gas chromatography-mass spectrometry |
LDI | Laser desorption/ionization |
SAXS | Small-angle X-ray scattering |
DCS | Differential scanning calorimetry |
PALS | Photon correlation spectroscopy |
TIRF | Total internal reflection fluorescence |
HNMR | High-resolution nuclear magnetic resonance |
PXRD | Powder X-ray diffraction |
VCD | Vibrational circular dichroism |
RBS | Rutherford backscattering spectrometry |
XANES | X-ray absorption near edge structure |
EXAFS | Extended X-ray absorption fine structure |
Data availability
No primary research results, software or code have been included and no new data were generated or analysed as part of this review.
Conflicts of interest
The authors declare no conflict of interest.
Acknowledgements
The authors extend their appreciation to the Deanship of Scientific Research at Northern Border University, Arar, KSA for funding this research work through the project number: “NBU-FFR-2024-2618-07”.
References
- Y. Zhang, An integrated diagnostic framework for water resource spatial equilibrium considering water-economy-ecology nexus, J. Cleaner Prod., 2023, 414, 137592 CrossRef.
- A. O. Iyiola, et al., Sustainable water use and management for agricultural transformation in Africa, in Water Resources Management for Rural Development, Elsevier, 2024, pp. 287–300 Search PubMed.
- V. Singh, et al., Toxic heavy metal ions contamination in water and their sustainable reduction by eco-friendly methods: isotherms, thermodynamics and kinetics study, Sci. Rep., 2024, 14(1), 7595 CrossRef CAS PubMed.
- S. Sundararaman, et al., Noteworthy synthesis strategies and applications of metal-organic frameworks for the removal of emerging water pollutants from aqueous environment, Chemosphere, 2024, 362, 142729 CrossRef CAS.
- S. Sehgal, et al., Impact of Water Contamination on Food Safety and Related Health Risks, in Microbial Biotechnology in the Food Industry: Advances, Challenges, and Potential Solutions, Springer, 2024, pp. 337–363 Search PubMed.
- S. Sarkar, A. Jaswal and A. Singh, Sources of inorganic nonmetallic contaminants (synthetic fertilizers, pesticides) in agricultural soil and their impacts on the adjacent ecosystems, in Bioremediation of Emerging Contaminants from Soils, Elsevier, 2024, pp. 135–161 Search PubMed.
- K. E. Ukhurebor, et al., Applications and contemporary issues with adsorption for water monitoring and remediation: a facile review, Top. Catal., 2024, 67(1), 140–155 CrossRef CAS.
- B. I. Nwadike, O. I. Falodun and A. A. Ogunjobi, Bacterial and viral contaminants in drinking water: Why do they really matter to us, in Environmental Pollution and Public Health, Elsevier, 2024, pp. 3–28 Search PubMed.
- N. Bono, et al., Effect of UV irradiation and TiO2-photocatalysis on airborne bacteria and viruses: an overview, Materials, 2021, 14(5), 1075 CrossRef CAS PubMed.
- A. Faanu, et al., Radiological landscape of natural resources and mining: unveiling the environmental impact of naturally occurring radioactive materials in Ghana’s mining areas, Heliyon, 2024, 10(3), e24959 CrossRef CAS.
- E. M. Cuerda-Correa, M. F. Alexandre-Franco and C. Fernández-González, Advanced oxidation processes for the removal of antibiotics from water. An overview, Water, 2019, 12(1), 102 CrossRef.
- M. H. Dehghani, et al., Sustainable remediation technologies for removal of pesticides as organic micro-pollutants from water environments: a review, Appl. Surf. Sci., 2024, 19, 100558 CrossRef.
- A. Maftouh, et al., Comparative review of different adsorption techniques used in heavy metals removal in water, Biointerface Res. Appl. Chem., 2023, 13, 397 CAS.
- V. Mozhiarasi and T. S. Natarajan, Bael fruit shell-derived activated carbon adsorbent: effect of surface charge of activated carbon and type of pollutants for improved adsorption capacity, Biomass Convers. Biorefin., 2024, 14(7), 8761–8774 CrossRef CAS.
- R. Ghanbari and E. N. Zare, Engineered MXene-polymer composites for water remediation: promises, challenges and future perspective, Coord. Chem. Rev., 2024, 518, 216089 CrossRef CAS.
- B. M. Thamer, et al., Modified electrospun polymeric nanofibers and their nanocomposites as nanoadsorbents for toxic dye removal from contaminated waters: a review, Polymers, 2020, 13(1), 20 CrossRef.
- H. Han, et al., A critical review of clay-based composites with enhanced adsorption performance for metal and organic pollutants, J. Hazard. Mater., 2019, 369, 780–796 CrossRef CAS PubMed.
- P. V. Mane, et al., Unveiling cutting-edge advances in high surface area porous materials for the efficient removal of toxic metal ions from water, Prog. Mater. Sci., 2024, 101314 CrossRef CAS.
- S. Shabaka, et al., Prevalence and risk assessment of microplastics in the Nile Delta estuaries: “The Plastic Nile” revisited, Sci. Total Environ., 2022, 852, 158446 CrossRef CAS PubMed.
- S. A. A. Ghani, et al., Characterization and distribution of plastic particles along Alexandria beaches, Mediterranean Coast of Egypt, using microscopy and thermal analysis techniques, Sci. Total Environ., 2022, 834, 155363 CrossRef.
- A. A. M. El-Sayed, et al., Microplastics contamination in commercial fish from Alexandria City, the Mediterranean Coast of Egypt, Environ. Pollut., 2022, 313, 120044 CrossRef CAS.
- M. M. Ghobashy, et al., Radiation synthesis of pH-sensitive 2-(dimethylamino) ethyl methacrylate/polyethylene oxide/ZnS nanocomposite hydrogel membrane for wound dressing application, J. Drug Delivery Sci. Technol., 2022, 73, 103399 CrossRef CAS.
- N. E. A. Abd El-Sattar, et al., Nanogel-mediated drug delivery system for anticancer agent: pH stimuli responsive poly(ethylene glycol/acrylic acid) nanogel prepared by gamma irradiation, Bioorg. Chem., 2022, 127, 105972 CrossRef.
- S. A. Alkhursani, et al., Application of nano-inspired scaffolds-based biopolymer hydrogel for bone
and periodontal tissue regeneration, Polymers, 2022, 14(18), 3791 CrossRef CAS.
- M. M. Ghobashy, I. M. Mousaa and G. S. El-Sayyad, Radiation synthesis of urea/hydrogel core shells coated with three different natural oils via a layer-by-layer approach: an investigation of their slow release and effects on plant growth-promoting rhizobacteria, Prog. Org. Coat., 2021, 151, 106022 CrossRef CAS.
- S. Mashhadikhan, et al., Breaking temperature barrier: highly thermally heat resistant polymeric membranes for sustainable water and wastewater treatment, Renewable Sustainable Energy Rev., 2024, 189, 113902 CrossRef CAS.
- I. G. B. Kaya, et al., Development of polymeric and polymer-based hybrid adsorbents for chromium removal from aqueous solution, Clean: Soil, Air, Water, 2011, 39(11), 980–988 CAS.
- S. M. Shaikh, et al., Impact of hybrid natural deep eutectic solvent and polyacrylamide flocculant systems on the flocculation of highly stable graphene oxide suspensions, Colloids Surf., A, 2024, 683, 133065 CrossRef CAS.
- M. Zhang, et al., Adsorptive behaviors and mechanisms for removing three organic pollutants from aqueous solutions by polyvinyl alcohol/porous carbon composite hydrogels, J. Environ. Chem. Eng., 2023, 11(5), 111095 CrossRef CAS.
- O. Moradi and G. Sharma, Emerging novel polymeric adsorbents for removing dyes from wastewater: a comprehensive review and comparison with other adsorbents, Environ. Res., 2021, 201, 111534 CrossRef CAS.
- C. K. Pooi and H. Y. Ng, Review of low-cost point-of-use water treatment systems for developing communities, npj Clean Water, 2018, 1(1), 11 CrossRef.
- P. Yaashikaa, P. S. Kumar and S. Karishma, Review on biopolymers and composites-evolving material as adsorbents in removal of environmental pollutants, Environ. Res., 2022, 212, 113114 CrossRef CAS.
- H. Song, et al., One-step construction of silver–polyaniline nanocomposite modified multifunctional sponges for wastewater remediation: adsorption, catalysis and antimicrobial applications, J. Mater. Chem. A, 2024, 12(11), 6747–6767 RSC.
- S. D. Richardson and S. Y. Kimura, Emerging environmental contaminants: challenges facing our next generation and potential engineering solutions, Environ. Technol. Innovation, 2017, 8, 40–56 CrossRef.
- J. O. Gonçalves, et al., Advanced technologies in water treatment: chitosan and its modifications as effective agents in the adsorption of contaminants, Int. J. Biol. Macromol., 2024, 132307 CrossRef.
- S. E. Sanders, H. Vanselous and P. B. Petersen, Water at surfaces with tunable surface chemistries, J. Phys.: Condens. Matter, 2018, 30(11), 113001 CrossRef.
- O. Agboola, et al., A review on polymer nanocomposites and their effective applications in membranes and adsorbents for water treatment and gas separation, Membranes, 2021, 11(2), 139 CrossRef CAS.
- S. B. Pillai, Adsorption in water and used water purification, in Handbook of Water and Used Water Purification, Springer, 2024, pp. 99–120 Search PubMed.
- G. Verma, et al., Recent Advances in Advanced Micro and Nanomanufacturing for Wastewater Purification, ACS Appl. Eng. Mater., 2024, 2(2), 262–285 CrossRef CAS.
- P. Xia, et al., Polymethylhydrosiloxane-modified gas-diffusion cathode for more efficient and durable H2O2 electrosynthesis in the context of water treatment, Appl. Catal., B, 2024, 343, 123467 CrossRef CAS.
- S. E. I. Lebouachera, et al., Understanding the factors affecting the adsorption of surface-active agents onto reservoir rock in chemical enhanced oil recovery applications: a comprehensive review, Chem. Afr., 2024, 1–24 Search PubMed.
- S. Ullah, et al., Activated carbon derived from biomass for wastewater treatment: synthesis, application and future challenges, J. Anal. Appl. Pyrolysis, 2024, 106480 CrossRef CAS.
- G. A. Al-Hazmi, et al., Superior adsorption and removal of doxorubicin from aqueous solution using activated carbon via thermally treated green adsorbent: isothermal, kinetic, and thermodynamic studies, Environ. Technol., 2024, 45(10), 1969–1988 CrossRef CAS.
- N. Takeda, et al., Solid–liquid partitioning and speciation of Pb(II) and Cd(II) on goethite under high pH conditions, as examined by subnanomolar heavy metal analysis, X-ray absorption spectroscopy, and surface complexation modeling, Chemosphere, 2024, 142766 CrossRef CAS.
- M. C. Holliday, D. R. Parsons and S. H. Zein, Agricultural pea waste as a low-cost pollutant biosorbent for methylene blue removal: adsorption kinetics, isotherm and thermodynamic studies, Biomass Convers. Biorefin., 2024, 14(5), 6671–6685 CrossRef CAS.
- R. Bhoje and A. K. Ghosh, Overview of water treatment technologies for preparation of drinking water, in Sustainable Technologies for Remediation of Emerging Pollutants from Aqueous Environment, Elsevier, 2024, pp. 431–453 Search PubMed.
- R. Adão, M. Beraja and N. Pandalai-Nayar, Fast and slow technological transitions, Int. J. Political Econ., 2024, 2(2), 183–227 Search PubMed.
- S. Mohamed Nasser, M. Abbas and M. Trari, Understanding the rate-limiting step adsorption kinetics onto biomaterials for mechanism adsorption control, Prog. React. Kinet. Mech., 2024, 49, 14686783241226858 CrossRef.
- A. Nayak, B. Bhushan and S. Kotnala, Fundamentals and mechanism of adsorption, in Sustainable Technologies for Remediation of Emerging Pollutants from Aqueous Environment, Elsevier, 2024, pp. 29–62 Search PubMed.
- E. W. Wambu, The Graphene Surface Chemistry and Adsorption Science, 2024 Search PubMed.
- V. Šubr, et al., Highly Effective Synthetic Polymer-Based Blockers of Non-Specific Interactions in Immunochemical Analyses, Polymers, 2024, 16(6), 758 CrossRef.
- G. C. Sedenho, et al., Exploring electron transfer: bioinspired, biomimetics, and bioelectrochemical systems for sustainable energy and Value-Added compound synthesis, Appl. Phys. Rev., 2024, 11(2), 1341 Search PubMed.
- S. Kainth, P. Sharma and O. Pandey, Green sorbents from agricultural wastes: a review of sustainable adsorption materials, Appl. Surf. Sci., 2024, 19, 100562 CrossRef.
- M. Chen, et al., CxNy-based materials as gas sensors: structure, performance, mechanism and perspective, Coord. Chem. Rev., 2024, 503, 215653 CrossRef CAS.
- A. Gupta, et al., A review of adsorbents for heavy metal decontamination: growing approach to wastewater treatment, Materials, 2021, 14(16), 4702 CrossRef CAS.
- A. Benettayeb, et al., Natural adsorbents for the removal of emerging pollutants and its adsorption mechanisms, in Sustainable Technologies for Remediation of Emerging Pollutants from Aqueous Environment, Elsevier, 2024, pp. 63–78 Search PubMed.
- M. A. Islam, et al., Adsorptive removal of paracetamol from aqueous media: a comprehensive review of adsorbent materials, adsorption mechanisms, recent advancements, and future perspectives, J. Mol. Liq., 2024, 123976 CrossRef.
- R. C. Muduli, et al., Investigating reversible hydrogen storage and performance of porous Si by kinetic study and pressure composition isotherms at up to 20 bar, Int. J. Hydrogen Energy, 2024, 59, 447–456 CrossRef CAS.
- C. Biz, J. Gracia and M. Fianchini, Experimental Evidences on Magnetism-Covalent Bonding Interplay in Structural Properties of Solids and during Chemisorption, Int. J. Mol. Sci., 2024, 25(3), 1793 CrossRef CAS PubMed.
- D. A. Knopf, et al., Desorption lifetimes and activation energies influencing gas–surface interactions and multiphase chemical kinetics, Atmos. Chem. Phys., 2024, 24(6), 3445–3528 CrossRef CAS.
- A. A. Abd, et al., Carbon dioxide removal through physical adsorption using carbonaceous and non-carbonaceous adsorbents: a review, J. Environ. Chem. Eng., 2020, 8(5), 104142 CrossRef CAS.
- X. Liu, et al., Adsorption selectivity of heavy metals by Na-clinoptilolite in aqueous solutions, Adsorption, 2019, 25, 747–755 CrossRef CAS.
- N. A. M. Sobri, N. Harun and M. Y. M. Yunus, A Review of the Ion Exchange Leaching Method for Extracting Rare Earth Elements from Ion Adsorption Clay, Chem. Eng. Res. Des., 2024, 208, 94–114 CrossRef CAS.
- M. A. N. Azman, et al., Forward Osmosis as a Contemporary Treatment Solution for Mitigating Radionuclide Pollution in Water Bodies, J. Environ. Chem. Eng., 2024, 112542 CrossRef.
- E. Flórez, C. Jimenez-Orozco and N. Acelas, Unravelling the influence of surface functional groups and surface charge on heavy metal adsorption onto carbonaceous materials: an in-depth DFT study, Mater. Today Commun., 2024, 39, 108647 CrossRef.
- B. Clark, et al., Ligand Exchange Adsorbents for Selective Phosphate and Total Ammonia Nitrogen Recovery from Wastewaters, Acc. Mater. Res., 2024, 5(4), 492–504 CrossRef CAS.
- C. Zhang, et al., Removing copper ion from wastewater by surface precipitation using novel hierarchical hydrated magnesium carbonate crystal, Chem. Eng. Sci., 2024, 286, 119665 CrossRef CAS.
- B. S. Rathi and P. S. Kumar, Application of adsorption process for effective removal of emerging contaminants from water and wastewater, Environ. Pollut., 2021, 280, 116995 CrossRef CAS.
- A. Ali Redha, Removal of heavy metals from aqueous media by biosorption, Arab J. Basic Appl. Sci., 2020, 27(1), 183–193 Search PubMed.
- I. Fenoglio, et al., Multiple aspects of the interaction of biomacromolecules with inorganic surfaces, Adv. Drug Delivery Rev., 2011, 63(13), 1186–1209 CrossRef CAS.
- A. M. Elgarahy, et al., A critical review of biosorption of dyes, heavy metals and metalloids from wastewater as an efficient and green process, Clean. Eng. Technol., 2021, 4, 100209 CrossRef.
- S. K. Parida, et al., Adsorption of organic molecules on silica surface, Adv. Colloid Interface Sci., 2006, 121(1–3), 77–110 CrossRef CAS PubMed.
- C. J. van Oss and R. F. Giese, Role of the polar properties of water in separation methods, Sep. Purif. Rev., 2011, 40(3), 163–208 CrossRef CAS.
- C. Zheng, et al., Treatment technologies for organic wastewater, Water Treatment, 2013, 11, 250–286 Search PubMed.
- P. Szabelski, Extended surface chirality for enantiospecific adsorption, Chem.–Eur. J., 2008, 14(27), 8312–8321 CrossRef CAS.
- M. Chafiq, et al., Beyond conventional: role of chiral metal-organic frameworks in asymmetric scenarios, Nano Today, 2024, 56, 102227 CrossRef CAS.
- W. Sun, et al., External electric field effects on asphaltene adsorption on Kaolinite-water interface: a molecular dynamics study, Appl. Clay Sci., 2024, 258, 107481 CrossRef CAS.
- Y. Luo, et al., Selective adsorption performance and mechanism of magnetic cationic resin for emerging contaminants, Sep. Purif. Technol., 2024, 333, 125858 CrossRef CAS.
- A. Nastasović, et al., Methacrylate-based polymeric sorbents for recovery of metals from aqueous solutions, Metals, 2022, 12(5), 814 CrossRef.
- T. Bouzid, et al., Optimizing Eriochrome Black T adsorption through In Situ polymerization of Poly(aniline-co-formaldehyde) on biochar: Multivariate approach using full factorial Design, Box-Behnken, AI, and DFT, Sep. Purif. Technol., 2024, 128107 CrossRef CAS.
- X. Wang, et al., Adsorption characteristics and mechanisms of water-soluble polymers (PVP and PEG) on kaolin and montmorillonite minerals, J. Hazard. Mater., 2024, 466, 133592 CrossRef CAS.
- S. Satyam and S. Patra, Innovations and challenges in adsorption-based wastewater remediation: a comprehensive review, Heliyon, 2024, 10(9), e29573 CrossRef CAS.
- B. Gao, et al., Graphene-based aerogels in water and air treatment: a review, Chem. Eng. J., 2024, 149604 CrossRef CAS.
- W. Al-Gethami, et al., Emerging environmentally friendly bio-based nanocomposites for the efficient removal of dyes and micropollutants from wastewater by adsorption: a comprehensive review, RSC Adv., 2024, 14(4), 2804–2834 RSC.
- H. R. Penchah, Functionalized and improved polymeric adsorbents, in Polymeric Adsorbents, Elsevier, 2024, pp. 159–176 Search PubMed.
- S. Vashista, A. Arora and M. K. Sah, Catalysing Sustainability with Keratin-Derived Adsorbent Materials for Enhanced Heavy Metal Remediation, Korean J. Chem. Eng., 2024, 1–21 Search PubMed.
- N. El Messaoudi, et al., Regeneration and reusability of non-conventional low-cost adsorbents to remove dyes from wastewaters in multiple consecutive adsorption–desorption cycles: a review, Biomass Convers. Biorefin., 2024, 14(11), 11739–11756 CrossRef CAS.
- M. A. Dada, et al., Innovative approaches to waste resource management: implications for environmental sustainability and policy, Eng. Sci. Technol. J., 2024, 5(1), 115–127 CrossRef.
- M. S. Khosrowshahi, et al., Recent Progress on Advanced Solid Adsorbents for CO2 Capture: From Mechanism to Machine Learning, Mater. Today Sustain., 2024, 100900 Search PubMed.
- A. Saini and S. K. Saini, Polymer Composites for Environmental Pollution and Remediation, in Polymer Composites: from Computational to Experimental Aspects, Springer, 2024 pp. 151–180 Search PubMed.
- K. Shanu, et al., Downstream Processing for Bio-product Recovery and Purification, in Recent Advances in Bioprocess Engineering and Bioreactor Design, Springer, 2024, pp. 139–169 Search PubMed.
- L. Jing, et al., Cellulose-based materials in environmental protection: a scientometrics and visual analysis review, Sci. Total Environ., 2024, 172576 CrossRef CAS.
- S. C. Momin, et al., Metal sequestration by Microcystis extracellular polymers: a promising path to greener water treatment, Environ. Sci. Pollut. Res., 2024, 31(7), 11192–11213 CrossRef CAS PubMed.
- T. A. Saleh, Materials, nanomaterials, nanocomposites, and methods used for the treatment and removal of hazardous pollutants from wastewater: treatment technologies for water recycling and sustainability, Nano-Struct. Nano-Objects, 2024, 39, 101231 CrossRef CAS.
- L. Doan, et al., Surface Modifications of Superparamagnetic Iron Oxide Nanoparticles with Chitosan, Polyethylene Glycol, Polyvinyl Alcohol, and Polyvinylpyrrolidone as Methylene Blue Adsorbent Beads, Polymers, 2024, 16(13), 1839 CrossRef CAS.
- N. K. Khanzada, et al., Sustainability in membrane technology: membrane recycling and fabrication using recycled waste, Membranes, 2024, 14(2), 52 CrossRef CAS.
- H. Shen, et al., Adsorption of phosphate onto amine functionalized nano-sized magnetic polymer adsorbents: mechanism and magnetic effects, RSC Adv., 2015, 5(28), 22080–22090 RSC.
- S. Kalia, et al., Magnetic polymer nanocomposites for environmental and biomedical applications, Colloid Polym. Sci., 2014, 292, 2025–2052 CrossRef CAS.
- M. Mohamady Ghobashy, Functionalized of wax-magnetic nanocomposite (Fe3O4/PE) pellets by radiation grafting of PAAc for safe dye removal, Cogent Chem., 2017, 3(1), 1363341 CrossRef.
- M. M. Ghobashy and M. A. Elhady, Radiation crosslinked magnetized wax (PE/Fe3O4) nano composite for selective oil adsorption, Compos. Commun., 2017, 3, 18–22 CrossRef.
- Y. Gao, et al., Nanocomposites of polyethylene with Fe3O4 nanoparticles via surface-initiated ROMP: thermomechanical, shape memory and photothermal properties, Composites, Part A, 2024, 177, 107923 CrossRef CAS.
- A. Katheria, et al., Fe3O4@g-C3N4 and MWCNT embedded highly flexible polymeric hybrid composite for simultaneous thermal control and suppressing microwave radiation, J. Alloys Compd., 2024, 988, 174287 CrossRef CAS.
- M. M. Ghobashy, A. M. Elbarbary and D. E. Hegazy, Synthesis of poly (vinylpyrrolidone)/Fe3O4@SiO2 nanoporous catalyst by γ-rays and evaluation their sono-photo-Fenton degradation of toluidine blue under magnetic field, Appl. Organomet. Chem., 2021, 35(11), e6388 CrossRef CAS.
- Y. Du, C. Ding and S. Agarwal, Sustainable Hierarchically Porous Reusable Metal-Organic Framework Sponge as a Heterogeneous Catalyst and Catalytic Filter for Degradation of Organic Dyes, Adv. Energy Sustainability Res., 2024, 5(4), 2300218 CrossRef CAS.
- R. Soman, Polysaccharide-based hydrogels for environmental applications, in Polysaccharides-Based Hydrogels, Elsevier, 2024, pp. 457–475 Search PubMed.
- M. S. Rostami, M. M. Khodaei and E. Benassi, Surface modified of chitosan by TiO2@MWCNT nanohybrid for the efficient removal of organic dyes and antibiotics, Int. J. Biol. Macromol., 2024, 133382 CrossRef CAS PubMed.
- A. M. Elbarbary and M. M. Ghobashy, Phosphorylation of chitosan/HEMA interpenetrating polymer network prepared by γ-radiation for metal ions removal from aqueous solutions, Carbohydr. Polym., 2017, 162, 16–27 CrossRef CAS PubMed.
- H. Ge and J. Du, Selective adsorption of Pb(II) and Hg(II) on melamine-grafted chitosan, Int. J. Biol. Macromol., 2020, 162, 1880–1887 CrossRef CAS.
- R. Kumar and R. K. Sharma, Synthesis and characterization of cellulose based adsorbents for removal of Ni(II), Cu(II) and Pb(II) ions from aqueous solutions, React. Funct. Polym., 2019, 140, 82–92 CrossRef CAS.
- B. Kaur, et al., Unveiling new horizons of progress on manipulating the structure and characterization of phosphate-modified polymer for selective uranium adsorption, Coord. Chem. Rev., 2024, 518, 216057 CrossRef CAS.
- J. Kaur, P. Sengupta and S. Mukhopadhyay, Critical review of bioadsorption on modified cellulose and removal of divalent heavy metals (Cd, Pb, and Cu), Ind. Eng. Chem. Res., 2022, 61(5), 1921–1954 CrossRef CAS.
- Y. Shi, et al., Exploiting extracellular polymeric substances (EPS) controlling strategies for performance enhancement of biological wastewater treatments: an overview, Chemosphere, 2017, 180, 396–411 CrossRef CAS PubMed.
- D. Cui, et al., Biosorption mechanism of aqueous Pb2+, Cd2+, and Ni2+ ions on extracellular polymeric substances (EPS), Archaea, 2020, 2020(1), 8891543 Search PubMed.
- Z. Zhang, et al., A novel biosorbent for dye removal: extracellular polymeric substance (EPS) of Proteus mirabilis TJ-1, J. Hazard. Mater., 2009, 163(1), 279–284 CrossRef CAS PubMed.
- S. Thambidurai and K. Pandiselvi, Polyaniline/natural polymer composites and nanocomposites, in Polyaniline Blends, Composites, and Nanocomposites, Elsevier, 2018, pp. 235–256 Search PubMed.
- A. Singh and J. G. Vijayan, Biosorbents for Wastewater Treatment, in Application of Nanotechnology for Resource Recovery from Wastewater, CRC Press, 2024, pp. 254–294 Search PubMed.
- T. T. More, et al., Extracellular polymeric substances of bacteria and their potential environmental applications, J. Environ. Manage., 2014, 144, 1–25 CrossRef CAS.
- P. K. Gautam, et al., Synthesis and applications of biogenic nanomaterials in drinking and wastewater treatment, J. Environ. Manage., 2019, 231, 734–748 CrossRef CAS.
- N. A. Oladoja, et al., Polysaccharides as a Green and Sustainable Resources for Water and Wastewater Treatment, Springer, 2017 Search PubMed.
- G. Crini, Recent developments in polysaccharide-based materials used as adsorbents in wastewater treatment, Prog. Polym. Sci., 2005, 30(1), 38–70 CrossRef CAS.
- M. Nasrollahzadeh, et al., Starch, cellulose, pectin, gum, alginate, chitin and chitosan derived (nano) materials for sustainable water treatment: a review, Carbohydr. Polym., 2021, 251, 116986 CrossRef CAS.
- A. M. Galvez, et al., Bacterial exopolysaccharide-mediated synthesis of silver nanoparticles and their application on bacterial biofilms, J. Microbiol., Biotechnol. Food Sci., 2019, 8(4), 970 CrossRef CAS.
- K. Rafińska, P. Pomastowski and B. Buszewski, Study of Bacillus subtilis response to different forms of silver, Sci. Total Environ., 2019, 661, 120–129 CrossRef.
- E. M. Mateo and M. Jiménez, Silver nanoparticle-based therapy: can it be useful to combat multi-drug resistant bacteria?, Antibiotics, 2022, 11(9), 1205 CrossRef CAS PubMed.
- Q. Wang, et al., Sequestration of nanoparticles by an EPS matrix reduces the particle-specific bactericidal activity, Sci. Rep., 2016, 6(1), 21379 CrossRef CAS.
- M. F. Salas-Orozco, et al., Mechanism of escape from the antibacterial activity of metal-based nanoparticles in clinically relevant bacteria: a systematic review, Nanomed. Nanotechnol. Biol. Med., 2024, 55, 102715 CrossRef CAS.
- D. H. Ellis, Silver Nanoparticles: the Immediate Benefits of Low Bacterial Resistance and the Long-Term Risk of Persistent Stress in Mammalian Cells, Wright State University, 2015 Search PubMed.
- J. Lu, et al., New insights of the bacterial response to exposure of differently sized silver nanomaterials, Water Res., 2020, 169, 115205 CrossRef CAS PubMed.
- M. F. Salas-Orozco, et al., Proteomic analysis of an Enterococcus faecalis mutant generated against the exposure to silver nanoparticles, J. Appl. Microbiol., 2022, 132(1), 244 CrossRef CAS.
- S. Vidya, et al., Preparation of gold nanoparticles by novel bacterial exopolysaccharide for antibiotic delivery, Life Sci., 2016, 153, 171–179 CrossRef.
- M. H. Siddique, et al., Effect of silver nanoparticles on biofilm formation and EPS production of multidrug-resistant Klebsiella pneumoniae, BioMed Res. Int., 2020,(1), 6398165 CAS.
- P. Thuptimdang, et al., Effect of silver nanoparticles on Pseudomonas putida biofilms at different stages of maturity, J. Hazard. Mater., 2015, 290, 127–133 CrossRef CAS.
- E. Terzioğlu, et al., Microbial silver resistance mechanisms: recent developments, World J. Microbiol. Biotechnol., 2022, 38(9), 158 CrossRef.
- A.-F. Tăbăran, et al., Silver nanoparticles for the therapy of tuberculosis, Int. J. Nanomed., 2020, 2231–2258 CrossRef.
- L. Gao, et al., Mediation of extracellular polymeric substances in microbial reduction of hematite by Shewanella oneidensis MR-1, Front. Microbiol., 2019, 10, 575 CrossRef.
- V. Slusarski-Santana, et al., Synthesis of inorganic nanomaterials using microorganisms, in Green Sustainable Process for Chemical and Environmental Engineering and Science, Elsevier, 2021, pp. 69–91 Search PubMed.
- Y. Yang, et al., Self-defense mechanisms of microorganisms from the antimicrobial effect of silver nanoparticles: highlight the role of extracellular polymeric substances, Water Res., 2022, 218, 118452 CrossRef CAS.
- N. Karaky, The Use of Metal Ions and Graphene-Based Compounds as Novel Antimicrobials Against Multidrug Resistant Pseudomonas aeruginosa, Klebsiella pneumoniae and Staphylococcus aureus, Manchester Metropolitan University, 2020 Search PubMed.
- B. Janani, et al., Impact of bovine serum albumin – a protein corona on toxicity of ZnO NPs in environmental model systems of plant, bacteria, algae and crustaceans, Chemosphere, 2021, 270, 128629 CrossRef CAS PubMed.
- S. J. Park, Protein-nanoparticle interaction: corona formation and conformational changes in proteins on nanoparticles, Int. J. Nanomed., 2020, 5783–5802 CrossRef CAS.
- V. Rachakonda, T. H. Pan and W. D. Le, Biomarkers of neurodegenerative disorders: how good are they?, Cell Research, 2004, 14(5), 349–358 CrossRef PubMed.
- W. Yan, et al., Frontiers in applications of porous materials in CO2 gas separation membranes: mechanisms, membrane properties, and future perspectives of porous aromatic frameworks (PAFs), J. Environ. Chem. Eng., 2024, 113509 CrossRef CAS.
- Q. Sun, et al., Tailored porous organic polymers for task-specific water purification, Acc. Chem. Res., 2020, 53(4), 812–821 CrossRef CAS.
- N.-Y. Huang, et al., An exceptionally stable octacobalt-cluster-based metal-organic framework for enhanced water oxidation catalysis, Chem. Sci., 2019, 10(42), 9859–9864 RSC.
- R. Zhao, et al., Porous aromatic framework modified
electrospun fiber membrane as a highly efficient and reusable adsorbent for pharmaceuticals and personal care products removal, ACS Appl. Mater. Interfaces, 2019, 11(18), 16662–16673 CrossRef CAS PubMed.
- M.-u.-d. Naik, Adsorbents for the Uranium Capture from Seawater for a Clean Energy Source and Environmental Safety: A Review, ACS Omega, 2024, 9(11), 12380–12402 CAS.
- S. Fajal, S. Dutta and S. K. Ghosh, Porous organic polymers (POPs) for environmental remediation, Mater. Horiz., 2023,(10), 4083–4138 RSC.
- A. V. Baskar, et al., Recovery, regeneration and sustainable management of spent adsorbents from wastewater treatment streams: a review, Sci. Total Environ., 2022, 822, 153555 CrossRef CAS.
- N. A. S. Feisal, et al., A comprehensive review of nanomaterials for efficient heavy metal ions removal in water treatment, J. Water Process Eng., 2024, 64, 105566 CrossRef.
- G. Wu, et al., Preparation of submicron PAF-56 particles and application in pervaporation, Microporous Mesoporous Mater., 2019, 279, 19–25 CrossRef CAS.
- L. Wang, et al., Polymer of intrinsic microporosity (PIM) films and membranes in electrochemical energy storage and conversion: a mini-review, Electrochem. Commun., 2020, 118, 106798 CrossRef CAS.
- D. Ren, et al., Recent developments of organic solvent resistant materials for membrane separations, Chemosphere, 2021, 271, 129425 CrossRef CAS PubMed.
- N. B. McKeown and P. M. Budd, Exploitation of intrinsic microporosity in polymer-based materials, Macromolecules, 2010, 43(12), 5163–5176 CrossRef CAS.
- B. Satilmis, Amidoxime modified polymers of intrinsic microporosity (PIM-1); a versatile adsorbent for efficient removal of charged dyes; equilibrium, kinetic and thermodynamic studies, J. Polym. Environ., 2020, 28, 995–1009 CrossRef CAS.
- H. Ye, et al., Advances in the application of polymers of intrinsic microporosity in liquid separation and purification: membrane separation and adsorption separation, Polym. Rev., 2021, 61(2), 239–279 CrossRef CAS.
- A. R. Antonangelo, N. Hawkins and M. Carta, Polymers of intrinsic microporosity (PIMs) for catalysis: a perspective, Curr. Opin. Chem. Eng., 2022, 35, 100766 CrossRef.
- N. Chaukura, B. B. Mamba and S. B. Mishra, Porous materials for the sorption of emerging organic pollutants from aqueous systems: the case for conjugated microporous polymers, J. Water Process Eng., 2017, 16, 223–232 CrossRef.
- H. J. Kim, et al., A carbonaceous membrane based on a polymer of intrinsic microporosity (PIM-1) for water treatment, Sci. Rep., 2016, 6(1), 36078 CrossRef CAS.
- S. Xu, Y. Luo and B. Tan, Recent development of hypercrosslinked microporous organic polymers, Macromol. Rapid Commun., 2013, 34(6), 471–484 CrossRef CAS.
- X.-Q. Huang, et al., Hypercrosslinked triazine-phloroglucinol hierarchical porous polymers for the effective removal of organic micropollutants, Chem. Eng. J., 2022, 435, 134990 CrossRef CAS.
- L. Yao, et al., N-heterocyclic hyper-cross-linked polymers for rapid and efficient adsorption of organic pollutants from aqueous solution, J. Mol. Liq., 2021, 325, 115002 CrossRef CAS.
- J. Wolska, et al., The influence of cross-linking density on the efficiency of post-synthetic sulfonation of hyper-cross-linked polymers and their adsorption capacity for antibiotic pollutants, J. Environ. Chem. Eng., 2023, 11(5), 110429 CrossRef CAS.
- W. Song, et al., Green synthesis of hypercrosslinked polymers for CO2 capture and conversion: recent advances, opportunities, and challenges, Green Chem., 2024, 26(5), 2476–2504 RSC.
- Q. Liao, et al., Rational design of hyper-crosslinked polymers for biomedical applications, J. Polym. Sci., 2024, 62(8), 1517–1535 CrossRef CAS.
- A. M. Borrero-López, A. Celzard and V. Fierro, Eco-Friendly Production of Hyper-Cross-Linked Polymers Using Mechanosynthesis and Bioresources: A Critical Review, ACS Sustain. Chem. Eng., 2022, 10(49), 16090–16112 CrossRef.
- S. Raza, et al., Electrochemistry of 2D-materials for the remediation of environmental pollutants and alternative energy storage/conversion materials and devices, a comprehensive review, Sustainable Mater. Technol., 2024, e00963 CrossRef CAS.
- C. M. C. Andrés, et al., Anticancer Activity of Metallodrugs and Metallizing Host Defense Peptides—Current Developments in Structure-Activity Relationship, Int. J. Mol. Sci., 2024, 25(13), 7314 CrossRef.
- A. Varyambath, et al., Tunable construction of biphenyl-based porous polymeric nanostructures and their synergistically enhanced performance in pollutant adsorption and energy storage, Microporous Mesoporous Mater., 2021, 312, 110800 CrossRef CAS.
- C. Guo, et al., Construction of multifunctional histidine-based hypercrosslinked hierarchical porous ionic polymers for efficient CO2 capture and conversion, Sep. Purif. Technol., 2023, 312, 123375 CrossRef CAS.
- H. Song, et al., Conferring poly (ionic liquid)s with high surface areas for enhanced catalytic activity, ACS Sustain. Chem. Eng., 2021, 9(5), 2115–2128 CrossRef CAS.
- W. Wu, et al., Nitrogen-doped porous carbon through K2CO3-activated bamboo shoot shell for an efficient CO2 adsorption, Fuel, 2024, 363, 130937 CrossRef CAS.
- S. K. Rajput and V. S. Mothika, Powders to Thin Films: Advances in Conjugated Microporous Polymer Chemical Sensors, Macromol. Rapid Commun., 2024, 45(10), 2300730 CrossRef CAS PubMed.
- K. Patra and H. Pal, Recent Advances in Porous Organic Polymers (POPs): The Emerging Sorbent materials with Promises towards Toxic and Radionuclides Metal Ions Separations, Mater. Today Sustain., 2024, 100799 Search PubMed.
- A. H. Zare, et al., A New Porphyrin-Porous Organic Polymer for Effective Adsorption of Mercury Ions, J. Polym. Environ., 2024, 1–12 Search PubMed.
- R. Zhang, et al., Novel porphyrin-based donor–acceptor conjugated organic polymers for efficient photocatalytic production of hydrogen peroxide in pure water, New J. Chem., 2024, 48(7), 3316–3324 RSC.
- A. Intisar, et al., Adsorptive and photocatalytic degradation potential of porous polymeric materials for removal of pesticides, pharmaceuticals, and dyes-based emerging contaminants from water, Chemosphere, 2023, 336, 139203 CrossRef CAS PubMed.
- Y. Yu, et al., A 1D porphyrin-based rigid conjugated polymer as efficient and recyclable visible-light driven photocatalyst, React. Funct. Polym., 2019, 143, 104340 CrossRef CAS.
- R. Liang, et al., Molecular level design of conjugated polymers based on a synergistic adsorption-photocatalytic strategy for efficient uranium capture, Chem. Eng. J., 2024, 153493 CrossRef CAS.
- Y. Jia, et al., Control of Microporous Structure in Conjugated Microporous Polymer Membranes for Post-Combustion Carbon Capture, Adv. Funct. Mater., 2024, 2407499 CrossRef.
- T. Tsukamoto, Recent advances in atomic cluster synthesis: a perspective from chemical elements, Nanoscale, 2024, 16, 10533–10550 RSC.
- P. Wang, et al., Simultaneous removal of organic micropollutants and metals from water by a multifunctional β-cyclodextrin polymer-supported-polyaniline composite, Chem. Eng. J., 2024, 482, 148826 CrossRef CAS.
- Z.-J. Lin, et al., Efficient capture and effective sensing of Cr2O72-from water using a zirconium metal-organic framework, Inorg. Chem., 2017, 56(22), 14178–14188 CrossRef CAS.
- J. Li, W. Ye and C. Chen, Removal of toxic/radioactive metal ions by metal-organic framework-based materials, in Interface Science and Technology, Elsevier, 2019, pp. 217–279 Search PubMed.
- P. Yang, et al., A robust MOF-based trap with high-density active alkyl thiol for the super-efficient capture of mercury, Chem. Commun., 2019, 55(86), 12972–12975 RSC.
- S. S. Hess, et al., Noncovalent peptide assembly enables crystalline, permutable, and reactive thiol frameworks, J. Am. Chem. Soc., 2023, 145(36), 19588–19600 CrossRef CAS PubMed.
- P. H. M. Andrade, et al., State of the art in visible-light photocatalysis of aqueous pollutants using metal-organic frameworks, J. Photochem. Photobiol., C, 2023, 100635 CrossRef CAS.
- S. Mingolla, et al., Effects of emissions caps on the costs and feasibility of low-carbon hydrogen in the European ammonia industry, Nat. Commun., 2024, 15(1), 3753 CrossRef CAS PubMed.
- H. Masoumi, Types of polymeric adsorbents, in Polymeric Adsorbents, Elsevier, 2024, pp. 1–46 Search PubMed.
- E. Sgreccia, et al., Heavy metal decontamination by ion exchange polymers for water purification: counterintuitive cation removal by an anion exchange polymer, J. Mater. Sci., 2024, 59(7), 2776–2787 CrossRef CAS.
- H. Kolya and C.-W. Kang, Bio-based polymeric flocculants and adsorbents for wastewater treatment, Sustainability, 2023, 15(12), 9844 CrossRef CAS.
- A. Priya, et al., Recent advances in microbial-assisted degradation and remediation of xenobiotic contaminants; challenges and future prospects, J. Water Process Eng., 2024, 60, 105106 CrossRef.
- Y. Wang, et al., Dual lignin-derived polymeric system for peptone removal from simulated wastewater, Environ. Pollut., 2024, 343, 123142 CrossRef CAS.
- G. Howard, et al., Evaluating the tradeoff between cost effectiveness and participation in agricultural conservation programs, Am. J. Agric. Econ., 2024, 106(2), 712–738 CrossRef.
- L. Hao, et al., Coagulation-centered three-step approach
for removing by-product organic pollutants from tetrabromobisphenol A industrial wastewater: experimental and theoretic investigations, Environ. Res., 2024, 247, 118113 CrossRef CAS.
- Y. Xu, et al., Mass Concentration and Removal Characteristics of Microplastics and Nanoplastics in a Drinking Water Treatment Plant, ACS ES&T Water, 2024, 4(8), 3348–3358 Search PubMed.
- O. U. Onwuka and A. Adu, Eco-efficient well planning: engineering solutions for reduced environmental impact in hydrocarbon extraction, Int. J. Appl. Res., 2024, 4(1), 33–43 Search PubMed.
- W. Zhang, et al., Stöber method to amorphous metal-organic frameworks and coordination polymers, Nat. Commun., 2024, 15(1), 5463 CrossRef CAS.
- J. Zhang, et al., Surface functionalization of polyurethanes: a critical review, Adv. Colloid Interface Sci., 2024, 103100 CrossRef CAS PubMed.
- K. Kuperkar, et al., Degradable polymeric bio (nano) materials and their biomedical applications: a comprehensive overview and recent updates, Polymers, 2024, 16(2), 206 CrossRef CAS.
- H. Li, et al., Sustainable Dual-Template Fabrication of Hierarchical Pore Structure for Enhanced and Stable CO2 Capture, ACS Sustain. Chem. Eng., 2024, 12(7), 2911–2920 CrossRef CAS.
- M. Akter, et al., Cellulose-based hydrogels for wastewater treatment: a concise review, Gels, 2021, 7(1), 30 CrossRef CAS PubMed.
- P. Li and Z. Liu, Glycan-specific molecularly imprinted polymers towards cancer diagnostics: merits, applications, and future perspectives, Chem. Soc. Rev., 2024, 53(4), 1870–1891 RSC.
- M. Gamal, et al., Current Advances in the Implementation of Magnetic Molecularly Imprinted Polymers Tailored for Enrichment of Target Analytes in Different Environmental Samples: An Overview from a Comprehensive Perspective, Trends Environ. Anal. Chem., 2024, e00236 CrossRef CAS.
- P. O. Isibor, D. O. Agbontaen and O. O. Adebayo, Nanochitosan-Based Water-Quality Enhancement, in Nanochitosan-Based Enhancement of Fisheries and Aquaculture: Aligning with Sustainable Development Goal 14-Life below Water, Springer, 2024, pp. 159–179 Search PubMed.
- M. S. Sengar, et al., Molecularly imprinted polymer technology for the advancement of its health surveillances and environmental monitoring, ACS Appl. Polym. Mater., 2024, 6(2), 1086–1105 CrossRef CAS.
- H. Feng, X. Shao and Z. Wang, Mechanically Controlled Radical Polymerization: From Harsh to Mild, ChemPlusChem, 2024, e202400287 CrossRef CAS PubMed.
- Y. Xue, et al., Naturally derived materials to enhance the membrane properties in (waste) water treatment applications-mechanisms, scale-up challenges and economic considerations, J. Water Process Eng., 2024, 57, 104647 CrossRef.
- A. Ansari, et al., Optimization of batch and packed-bed column Cr(VI) adsorption of an amine-rich chitosan/polyethyleneimine composite: application in electroplating wastewater treatment, Environ. Sci.: Water Res. Technol., 2024, 10(7), 1572–1585 RSC.
- J. Wang, et al., Green recycling of spent Li-ion battery cathodes via deep-eutectic solvents, Energy Environ. Sci., 2024, 17, 867–884 RSC.
- I. K. Abdel Maksoud, et al., Radiation synthesis and chemical modifications of p (AAm-co-AAc) hydrogel for improving their adsorptive removal of metal ions from polluted water, Sci. Rep., 2023, 13(1), 21879 CrossRef CAS PubMed.
- M. P. Eastman, et al., Polymerization of benzene and aniline on Cu(II)-exchanged hectorite clay films: a scanning force microscope study, Clays Clay Miner., 1996, 44, 769–773 CrossRef CAS.
- N.-C. Godja and F.-D. Munteanu, Hybrid Nanomaterials: A Brief Overview of Versatile Solutions for Sensor Technology in Healthcare and Environmental Applications, Biosensors, 2024, 14(2), 67 CrossRef CAS.
- M. I. A. A. Maksoud, et al., Gamma radiation-induced synthesis of organoclays based polyaniline and ilmenite clay minerals for cesium ions removal from aqueous solutions, Sep. Purif. Technol., 2023, 305, 122434 CrossRef.
- P. Kumar, et al., Highlights in Interface of Wastewater Treatment by Utilizing Metal Organic Frameworks: Purification and Adsorption Kinetics, Langmuir, 2024, 40(10), 5040–5059 CrossRef CAS PubMed.
- G. Lipinski, et al., Application of Raman Spectroscopy for Sorption Analysis of Functionalized Porous Materials, Adv. Sci., 2022, 9(9), 2105477 CrossRef PubMed.
- Y. Hu, et al., Detection of melamine in milk using molecularly imprinted polymers–surface enhanced Raman spectroscopy, Food Chem., 2015, 176, 123–129 CrossRef CAS PubMed.
- Y. Ma, et al., Rapid detection of melamine by DNA Walker mediated SERS sensing technique based on signal amplification function, Microchim. Acta, 2024, 191(5), 283 CrossRef CAS.
- L. Geng, et al., Recent progress of the research of metal-organic frameworks-molecularly imprinted
polymers (MOFs-MIPs) in food safety detection field, Food Chem., 2024, 140330 CrossRef CAS.
- Z.-W. Lin, et al., Structural Features of Styrene-Functionalized Cyclodextrin Polymers That Promote the Adsorption of Perfluoroalkyl Acids in Water, ACS Appl. Mater. Interfaces, 2024, 16(22), 28409–28422 CrossRef CAS.
- X. Du, et al., Preparation of electrospun cellulose acetate/chitosan membranes for efficient sorption of heavy metals from aqueous solutions, Colloids Surf., A, 2024, 134698 CrossRef CAS.
- P. G. Mileo, et al., Nanoscale Simulation of Plastic Contaminants Migration in Packaging Materials and Potential Leaching into Model Food Fluids, Langmuir, 2024, 40(24), 12475–12487 CrossRef CAS PubMed.
- I. Ignat, et al., A comparative study on adsorption of gallic acid onto polymeric adsorbents with amine functional groups, Cellul. Chem. Technol., 2011, 45(3), 251 CAS.
- R. Ezzati, M. Azizi and S. Ezzati, A theoretical approach for evaluating the contributions of pseudo-first-order and pseudo-second-order kinetics models in the Langmuir rate equation, Vacuum, 2024, 222, 113018 CrossRef CAS.
- F. Ronchetti, L. Springer and K. Purnhagen, The regulatory Landscape in the EU for Dairy products derived from precision fermentation, SpringerBriefs in Law, 2024 Search PubMed.
- M. S. Hossain, et al., Benign separation, adsorption, and recovery of rare-earth Yb(III) ions with specific ligand-based composite adsorbent, Process Saf. Environ. Prot., 2024, 185, 367–374 CrossRef CAS.
- J. Bayuo, et al., Adsorption and desorption processes of toxic heavy metals, regeneration and reusability of spent adsorbents: economic and environmental sustainability approach, Adv. Colloid Interface Sci., 2024, 103196 CrossRef CAS.
- A. Nasiri, et al., Facile and green synthesis of recyclable, environmentally friendly, chemically stable, and cost-effective magnetic nanohybrid adsorbent for tetracycline adsorption, Heliyon, 2024, 10(2), e24179 CrossRef CAS PubMed.
- D. Suresh, et al., Waste Reutilization in Pollution Remediation: Paving New Paths for Wastewater Treatment, J. Environ. Chem. Eng., 2024, 113570 CrossRef CAS.
- R. Sharma, et al., Biodegradable and conducting hydrogels based on Guar gum polysaccharide for antibacterial and dye removal applications, J. Environ. Manage., 2015, 162, 37–45 CrossRef CAS.
- R. Sharma, et al., Ggum-poly (itaconic acid) based superabsorbents via two-step free-radical aqueous polymerization for environmental and antibacterial applications, J. Polym. Environ., 2017, 25, 176–191 CrossRef CAS.
- R. Sahraei and M. Ghaemy, Synthesis of modified gum tragacanth/graphene oxide composite hydrogel for heavy metal ions removal and preparation of silver nanocomposite for antibacterial activity, Carbohydr. Polym., 2017, 157, 823–833 CrossRef CAS.
- N. N. Dil and M. Sadeghi, Free radical synthesis of nanosilver/gelatin-poly (acrylic acid) nanocomposite hydrogels employed for antibacterial activity and removal of Cu(II) metal ions, J. Hazard. Mater., 2018, 351, 38–53 CrossRef CAS PubMed.
- R. R. Mohamed, M. H. A. Elella and M. W. Sabaa, Cytotoxicity and metal ions removal using antibacterial biodegradable hydrogels based on N-quaternized chitosan/poly(acrylic acid), Int. J. Biol. Macromol., 2017, 98, 302–313 CrossRef CAS.
- U. Baig, M. Faizan and M. Sajid, Effective removal of hazardous pollutants from water and deactivation of water-borne pathogens using multifunctional synthetic adsorbent materials: a review, J. Cleaner Prod., 2021, 302, 126735 CrossRef.
- S. A. Khan, et al., Antibacterial nanocomposites based on chitosan/Co-MCM as a selective and efficient adsorbent for organic dyes, Int. J. Biol. Macromol., 2016, 91, 744–751 CrossRef CAS PubMed.
- A. Sanmugam, et al., One-pot facile methodology to synthesize chitosan-ZnO-graphene oxide hybrid composites for better dye adsorption and antibacterial activity, Nanomaterials, 2017, 7(11), 363 CrossRef.
- T. Taghipour, et al., Mild synthesis of a Zn(II) metal organic polymer and its hybrid with activated carbon: application as antibacterial agent and in water treatment by using sonochemistry: optimization, kinetic and isotherm study, Ultrason. Sonochem., 2018, 41, 389–396 CrossRef CAS.
- Y. Haldorai and J.-J. Shim, An efficient removal of methyl orange dye from aqueous solution by adsorption onto chitosan/MgO composite: a novel reusable adsorbent, Appl. Surf. Sci., 2014, 292, 447–453 CrossRef CAS.
- T. Kamal, et al., Adsorption and photocatalyst assisted dye removal and bactericidal performance of ZnO/chitosan coating layer, Int. J. Biol. Macromol., 2015, 81, 584–590 CrossRef CAS PubMed.
- M. Ahmad, et al., Preparation and characterization of antibacterial thiosemicarbazide chitosan as efficient Cu(II) adsorbent, Carbohydr. Polym., 2015, 132, 164–172 CrossRef CAS.
- P. Kumar, et al., Synthesis and characterization of crosslinked chitosan for effective dye removal antibacterial activity, Int. J. Biol. Macromol., 2019, 139, 752–759 CrossRef CAS PubMed.
- M. S. S. Dorraji, et al., Polyurethane foam-cadmium sulfide nanocomposite with open cell structure: dye removal and antibacterial applications, Korean J. Chem. Eng., 2017, 34, 547–554 CrossRef CAS.
- A. Dhillon, et al., Excellent fluoride decontamination and antibacterial efficacy of Fe–Ca–Zr hybrid metal oxide nanomaterial, J. Colloid Interface Sci., 2015, 457, 289–297 CrossRef CAS.
- H. Hosseinzadeh, Microwave-assisted synthesis of kappa-carrageenan beads containing silver nanoparticles with dye adsorption and antibacterial properties, J. Nanostruct., 2016, 6(2), 132–139 CAS.
- S. Zhang, et al., Adsorption and antibacterial activity of silver-dispersed carbon aerogels, J. Appl. Polym. Sci., 2006, 102(2), 1030–1037 CrossRef CAS.
- Y. Jiang, et al., Magnetic chitosan–graphene oxide composite for anti-microbial and dye removal applications, Int. J. Biol. Macromol., 2016, 82, 702–710 CrossRef CAS.
- R. Bushra, et al., Synthesis, characterization, antimicrobial activity and applications of polyaniline Ti(IV) arsenophosphate adsorbent for the analysis of organic and inorganic pollutants, J. Hazard. Mater., 2014, 264, 481–489 CrossRef CAS.
- F. C. P. Masim, et al., Synergistic effect of PANI–ZrO2 composite as antibacterial, anti-corrosion, and phosphate adsorbent material: synthesis, characterization and applications, Environ. Technol., 2019, 40(2), 226–238 CrossRef CAS.
- A. A. Aryee, et al., A review on adsorbents for the remediation of wastewater: antibacterial and adsorption study, J. Environ. Chem. Eng., 2021, 9(6), 106907 CrossRef CAS.
- A. Amari, et al., Clay-polymer nanocomposites: preparations and utilization for pollutants removal, Materials, 2021, 14(6), 1365 CrossRef CAS PubMed.
- A. Giwa, et al., Recent advances in advanced oxidation processes for removal of contaminants from water: a comprehensive review, Process Saf. Environ. Prot., 2021, 146, 220–256 CrossRef CAS.
- I. R. Baxendale, et al., Achieving continuous manufacturing: technologies and approaches for synthesis, workup, and isolation of drug substance. May 20–21, 2014 Continuous Manufacturing Symposium, J. Pharm. Sci., 2015, 104(3), 781–791 CrossRef CAS.
|
This journal is © The Royal Society of Chemistry 2024 |
Click here to see how this site uses Cookies. View our privacy policy here.