DOI:
10.1039/D4RA05796A
(Paper)
RSC Adv., 2024,
14, 28340-28344
Rational construction of luminescent Eu-doped Y-MOF for ratiometric temperature sensing†
Received
10th August 2024
, Accepted 30th August 2024
First published on 5th September 2024
Abstract
Introducing lanthanide(III) ions into a MOF structure is one of the most effective strategies to construct luminescent MOFs with multiple emission centers for fluorescent applications. In this work, a functionalized Eu3+-doped Y-MOF (Eu@SNNU-325) was constructed by using a cation exchange strategy. The photoluminescence result shows that Eu@SNNU-325 exhibits a unique emission spectrum, namely, the absence of the organic ligand peak and the very strong Y3+/Eu3+ characteristic peaks. Interestingly, the smart luminescent Eu@SNNU-325 as a ratiometric thermometer for temperature sensing has good self-calibrated ability and a high maximum relative sensitivity (Sm) value (1.2% K−1 at 260 K). This work presents the construction of a smart Eu3+-functionalized Y-MOF thermometer through a cation exchange strategy, providing a good idea for the future development and design of Y-MOF thermometers.
Introduction
Luminescent metal–organic frameworks (LMOFs), as an important class of materials, have advantages for application in light-emitting diodes (LEDs), fluorescent sensors, and bioimaging, etc.1–15 Generally, most luminescent MOFs with single emission centers exhibit many disadvantages, such as low sensitivity, poor accuracy, and low responsiveness, which limits their widespread application.16–20 It is well known that the luminescent MOFs with lanthanide ions centers have attracted much research interest owing to their designability, high sensitivity, fast response, and high accuracy.21–23 Therefore, the rational design of lanthanide ions based-MOFs (Ln-MOFs) is still a great challenge.
In recent years, the luminescent Ln-MOF thermometers have been widely studied due to their distinct characteristic emissions, wide temperature detection range and strong anti-interference abilities.24–30 On the other hand, when constructing Ln-MOFs, selecting appropriate organic ligands as antenna chromophores plays a key role in sensitizing Ln3+ ion emissions. However, MOF materials based on rare earth Y3+ ions are still rare.
To our knowledge, a highly ultra-stable yttrium-MOF (SNNU-325, SNNU = Shaanxi Normal University) consists of the Y3+ ions, π-conjugated ligands, large octahedral cages, and quadrilateral channels, which can be potentially applied in the field of fluorescence sensing.31 This yttrium-MOF (Y-MOF) has been reported by Zhai's group, and its crystal structure, characterization, and gas adsorption/separation performance have been studied. However, the fluorescence performance of this Y-MOF has not yet been studied. Based on the structural characteristics of SNNU-325, we speculate that this MOF constructed from Y3+ ions and rich π-conjugated aromatic ligands should exhibit excellent unique fluorescence properties.
Herein, we have successfully synthesized this Y-MOF (SNNU-325) and further studied its structural characteristics. The result of elemental mappings demonstrates that Sc3+ ions are uniformly dispersed on the MOF. The presence of Sc3+ ions in the pores provides technical support for introducing lanthanide(III) ions with strong luminescent properties via a cation exchange strategy (Scheme 1). More significantly, the luminescent Eu@SNNU-325 as a ratiometric thermometer not only exhibits the Y3+/Eu3+ characteristic peaks but also shows good fluorescence temperature sensing over a wide range of temperature from 80 K to 260 K.
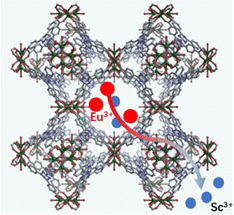 |
| Scheme 1 Schematics illustrating the cation exchange strategy to construct a functionalized Eu-doped Y-MOF. | |
Experimental
Synthesis of SNNU-325
A mixture of the ligand H3TATB (2,4,6-tris(4-carboxyphenyl)-1,3,5-triazine) (12 mg, 0.027 mmol), Y(NO3)3·6H2O (12 mg, 0.03 mmol), Sc(NO3)3·6H2O (16 mg, 0.07 mmol) and 2-FBA (2-fluorobenzoic acid) (173 mg, 1.23 mmol) was ultrasonically dissolved in DMF (N,N-dimethylformamide) (6 mL)/H2O (0.9 mL) solvent system in a glass vial (20 mL). The mixed solution was placed in an oven preheated at 90 °C for 5 days and then cooled to room temperature. After that, the colorless cubic block crystals were obtained by filtering and washing the fresh DMF and EtOH, and then dried at 50 °C.
Synthesis of Eu@SNNU-325
The above obtained Y-MOF (50 mg) and EuCl3·6H2O (50 mg) were dispersed in DMF (5 mL) and then placed in an oven at 75 °C for 24 h. The obtained Eu@SNNU-325 sample was obtained by washing several times with fresh DMF and then dried in an oven at 50 °C.
Results and discussion
Characterizations of SNNU-325 and Eu@SNNU-325
The preparation of SNNU-325 was reported in the literature.31 The PXRD peaks of as-synthesized SNNU-325 are in good agreement with the simulated peaks from the crystal structure data (Fig. 1). The author pointed out in the original paper that SNNU-325 cannot be prepared without the addition of Sc(NO3)3·6H2O. After careful analysis of this Y-MOF structure, we speculate that Sc3+ ions not only play a structural guiding role in the formation of MOF and but also as cations exist within the MOF framework. To prove our hypothesis, we first soaked the as-synthesized Y-MOF in methanol for 4 days, and then conducted EDS testing on the as-synthesized and the methanol-treated sample, respectively (Fig. S1 and S2†). The EDS results show that the atomic ratios of Sc/Y significantly decrease from 1.99 to 0.35 in the as-synthesized sample and the methanol-treated sample. The PXRD peaks of the methanol-treated sample are well-matched with the as-synthesized sample (Fig. 1). Through the methanol-treated sample for 4 days, it can be clearly observed that the Sc3+ ions only decrease in the MOF framework, but cannot be completely removed. This phenomenon indicates that there is a strong interaction force between Sc3+ ions within the MOF framework. Therefore, the presence of Sc3+ ions within the MOF framework encourages us to design Eu3+ doped Y-MOFs by using a cation exchange strategy.
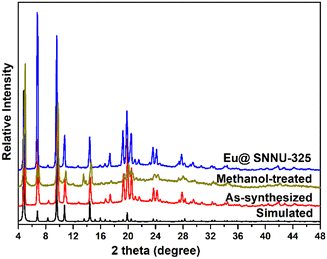 |
| Fig. 1 PXRD patterns of the simulated, as-synthesized SNNU-325, methanol-treated SNNU-325 and Eu@SNNU-325. | |
The mixture of as-synthesized Y-MOF and EuCl3·6H2O under DMF solvent was further employed to construct Eu-functionalized Y-MOFs by post-synthetic modification strategy. By comparing the powder X-ray diffraction (PXRD) peaks (Fig. 1), the peaks of Eu@SNNU-325 remain highly consistent with that of the as-synthesized sample, indicating that this Y-MOF still maintains its crystalline structure after the incorporation of Eu3+ ions into the framework. As shown in Fig. 2a and b, the SEM images show that the morphologies of Eu3+ remain consistent before and after being introduced into the MOF framework. The elemental mapping images clearly show the homogeneous distribution of C, N, O, F, Cl, Y, Sc and Eu elements in the Eu@SNNU-325 (Fig. S3†), indicating that the Eu3+ ions were incorporated into the MOF framework through the Sc3+ ions exchange process. Notably, the presence of F or Cl elements in the Eu@SNNU-325 are originated from the raw materials (2-FBA and EuCl3·6H2O). Moreover, the FT-IR spectra of SNNU-325 and Eu@SNNU-325 are basically consistent, further indicating that the incorporation of Eu3+ ions not change the original structure (Fig. 2c). To demonstrate whether Eu3+ has exchanged Y3+ ions in the skeleton, X-ray photoelectron spectroscopy (XPS) spectra of SNNU-325 and Eu@SNNU-325 were recorded. The results show that the O 1s XPS spectra of SNNU-325 and Eu@ SNNU-325 remain consistent, indicating that Eu3+ ions are not bonded to oxygen atoms in the MOF skeleton, and also indicating that Eu3+ ions do not exchange Y3+ ions in the skeleton (Fig. 2d).
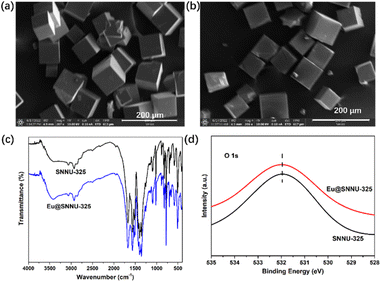 |
| Fig. 2 SEM images of SNNU-325 (a) and Eu@SNNU-325 (b). (c) FT-IR spectra of SNNU-325 and Eu@ SNNU-325. (d) The O 1s XPS spectra of SNNU-325 and Eu@ SNNU-325. | |
Photoluminescence study
Considering the unique Y3+ ion characteristic, rich π-conjugated organic ligand, and the very obvious Eu3+ emission bands, we measured the solid-state photoluminescence (PL) spectra of SNNU-325 and Eu@SNNU-325 at room temperature. As shown in Fig. 3a, the emission spectrum of SNNU-325 shows two characteristic emission centers (λex = 355 nm), one at 435 nm belonging to ligand emission,32 and the other at 488, 544, 578, 592, 618, 651 and 699 nm belonging to Y3+ ion characteristic emission.33 However, the emission spectrum of Eu@SNNU-325 is consistent with that of SNNU-325, except for the disappearance of the emission peak of the TATB3− ligand (λex = 300 nm) (Fig. 3b). It can be clearly observed that the absence of the emission band (at about 435 nm) arising from the ligand, indicating that the ligand emission process in Eu@SNNU-325 should be suppressed by the energy transfer from the ligand to the Eu3+ and Y3+ ions.34,35 Under UV-light irradiation, SNNU-325 displays dark purple visible emission, and Eu@SNNU-325 displays red visible emission (insets in Fig. 3b). The above results demonstrate that the emission spectrum of Eu3+ doped Y-MOFs has not changed compared to that of Y-MOF, but affect the energy transfer between the organic ligand and Eu3+ and Y3+ ions, resulting in Eu@MOF displaying strong red emission of Eu3+. This phenomenon is very rare in other Eu3+-doped MOF systems.
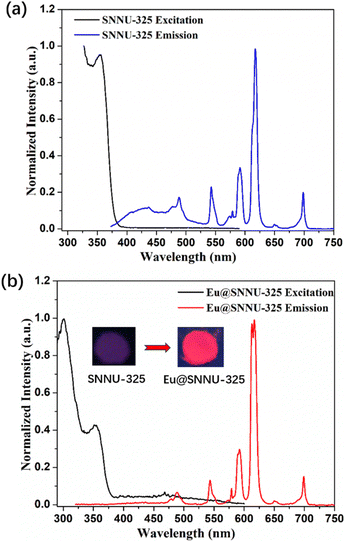 |
| Fig. 3 Excitation and emission spectra of SNNU-325 (λex = 353 nm) (a) and Eu@SNNU-325 (λex = 300 nm) (b) (insets show the photographs under 254 nm UV light). | |
Temperature-dependent emission properties
The significant luminescence characteristics of Y3+/Eu3+ ions in the Eu@SNNU-325 inspired us to evaluate its application in the field of low-temperature sensing. The temperature-dependent fluorescence performance of Eu@SNNU-325 was studied over a wide range of temperature from 80 K to 300 K. As shown in Fig. 4, the luminescence intensities in the Eu@SNNU-325 gradually decrease of both I618 and I544 with the increasing temperature under excitation at 300 nm, which can be mainly attributed to the thermal deactivation of the Y3+/Eu3+ ions. As a ratiometric luminescent thermometer, the intensity ratio of I618/I544 (Δ) of Eu@SNNU-325 was used to evaluate its temperature sensing performance. The Boltzmann equation (Δ = (A1 − A2)/(1 + exp((T − T0)/dT)) + A2) was used to fit the relationship between Δ and the a wide temperature range (80–260 K). Interestingly, the result shows that Eu@SNNU-325 exhibits a good fit (R2 = 0.9946) (Fig. 5a, and Table S1†).
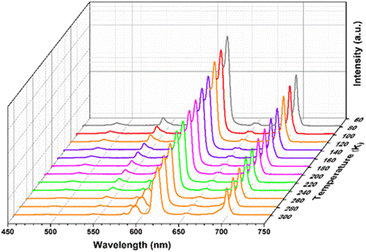 |
| Fig. 4 Temperature-dependent emission spectra of Eu@SNNU-325 recorded in the range of 80–300 K. | |
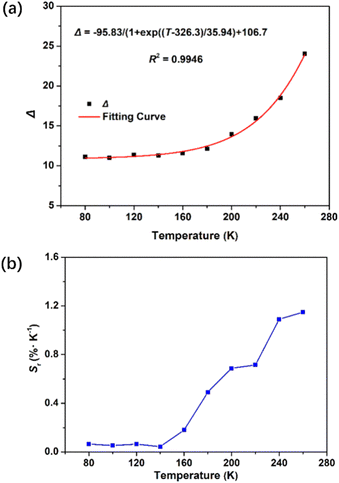 |
| Fig. 5 (a) Temperature-dependent emission intensity ratio Δ (I618/I544) and the fitted curve of Eu@SNNU-325 from 80 to 260 K. (b) Temperature-dependent relative sensitivity (Sr) of Eu@SNNU-325. | |
To further evaluate the sensing sensitivity, the relative sensitivity (Sr, Sr = |(∂Δ/∂T)/Δ|) was used as an important index. Because Sr represents an intrinsic nature of the thermometer. As a result, the maximum relative sensitivity (Sm) was determined to be 1.2% K−1 at 260 K (Fig. 5b), which was comparable to that of the other reported luminescent MOF thermometers such as Tb0.80Eu0.20(bpda) (1.39),36 ZJU-88⊃perylene (1.28)37 and Nd0.577Yb0.423BDC-F4 (1.2).38 The above results show that Eu@SNNU-325 can be used as a smart thermometer for self-calibrating temperature sensing.
Conclusions
In summary, a functionalized Eu-doped Y-MOF (Eu@SNNU-325) has been constructed by the cation exchange strategy. The obtained Eu@SNNU-325 was characterized by combining the PXRD, SEM, XPS, FT-IR, and EDX mapping. Due to the introduction of Eu3+ ions into the Y-MOF framework, Eu@SNNU-325 exhibits the characteristic emission centers of Y3+/Eu3+ ions. Moreover, the smart luminescent Eu@SNNU-325 exhibits highly sensitive temperature sensing over a wide range of temperature from 80 K to 260 K. Most importantly, the maximum relative sensitivity (Sm) of Eu@SNNU-325 reached 1.2% K−1 at 260 K, indicating that it can be applied as a temperature transfer material in practical applications. This work provides a powerful and effective synthesis strategy for constructing Eu-doped Y-MOFs and applying a field of fluorescence temperature sensing.
Data availability
Data are available upon request from the authors.
Author contributions
Wei Wei: experiment instruction, methodology. Xi Li: data curation. Yong-Ya Zhang: software. Jian-Wei Zhang: writing – review and editing.
Conflicts of interest
There are no conflicts to declare.
Acknowledgements
This work was supported by the National Natural Science Foundation of China (22301172 and 52472222) and the Science and Technology Innovation Talents in Universities of Henan Province (22HASTIT028).
References
- Y. Cui, Y. Yue, G. Qian and B. Chen, Chem. Rev., 2012, 112, 1126–1162 CrossRef CAS PubMed.
- B. Li, H.-T. Fan, S.-Q. Zang, H.-Y. Li and L.-Y. Wang, Coord. Chem. Rev., 2018, 377, 307–329 CrossRef CAS.
- E. J. McLaurin, L. R. Bradshaw and D. R. Gamelin, Chem. Mater., 2013, 25, 1283–1292 CrossRef CAS.
- A. Karmakar and J. Li, Chem. Commun., 2022, 58, 10768–10788 RSC.
- G.-H. Wen, X.-M. Chen, K. Xu, X. Xie, S.-S. Bao and L.-M. Zheng, Dalton Trans., 2021, 50, 17129–17139 RSC.
- J. Dong, D. Zhao, Y. Lu and W.-Y. Sun, J. Mater. Chem. A, 2019, 7, 22744–22767 RSC.
- S. Bhowal and A. Ghosh, RSC Adv., 2021, 11, 27787–27800 RSC.
- S.-Y. Li, X. Yan, J. Lei, W.-J. Ji, S.-C. Fan, P. Zhang and Q.-G. Zhai, ACS Appl. Mater. Interfaces, 2022, 14, 55997–56006 CrossRef CAS PubMed.
- Z. Hu, B. J. Deibert and J. Li, Chem. Soc. Rev., 2014, 43, 5815–5840 RSC.
- Y. Zhang, S. Yuan, G. Day, X. Wang, X. Yang and H.-C. Zhou, Coord. Chem. Rev., 2018, 354, 28–45 CrossRef CAS.
- G.-L. Yang, X.-L. Jiang, H. Xu and B. Zhao, Small, 2021, 17, 2005327 CrossRef CAS PubMed.
- Y. Liu, X.-Y. Xie, C. Cheng, Z.-S. Shao and H.-S. Wang, J. Mater. Chem. C, 2019, 7, 10743–10763 RSC.
- M. Ding, R. W. Flaig, H.-L. Jiang and O. M. Yaghi, Chem. Soc. Rev., 2019, 48, 2783–2828 RSC.
- Y. Cui, B. Li, H. He, W. Zhou, B. Chen and G. Qian, Acc. Chem. Res., 2016, 49, 483–493 CrossRef CAS PubMed.
- D. Yue, Z. Li, D. Chen, W. Li, B. Qin, B. Zhang, Y. Li, D. Zhao and Z. Wang, J. Solid State Chem., 2023, 327, 124279 CrossRef CAS.
- Z.-H. Zhu, Z. Ni, H.-H. Zou, G. Feng and B. Z. Tang, Adv. Funct. Mater., 2021, 31, 2106925 CrossRef CAS.
- Z.-J. Li, X. Wang, L. Zhu, Y. Ju, Z. Wang, Q. Zhao, Z.-H. Zhang, T. Duan, Y. Qian, J.-Q. Wang and J. Lin, Inorg. Chem., 2022, 61, 7467–7476 CrossRef CAS PubMed.
- E. Velasco, G. Zhang, S. J. Teat, K. Tan, S. Ullah, T. Thonhauser and J. Li, Inorg. Chem., 2023, 62, 16435–16442 CrossRef CAS PubMed.
- C. Yao, Y. Xu and Z. Xia, J. Mater. Chem. C, 2018, 6, 4396–4399 RSC.
- C. Yang, X. Xu and B. Yan, Inorg. Chem. Front., 2023, 10, 2951–2960 RSC.
- S. Wu, H. Min, W. Shi and P. Cheng, Adv. Mater., 2020, 32, 1805871 CrossRef CAS PubMed.
- H.-Q. Yin and X.-B. Yin, Acc. Chem. Res., 2020, 53, 485–495 CrossRef CAS PubMed.
- Y.-M. Wang, X.-T. Tian, H. Zhang, Z.-R. Yang and X.-B. Yin, ACS Appl. Mater. Interfaces, 2018, 10, 22445–22452 CrossRef CAS PubMed.
- Y. Yang, Y. Wang, Y. Feng, X. Song, C. Cao, G. Zhang and W. Liu, Talanta, 2020, 208, 120354 CrossRef CAS PubMed.
- K. Li, D. Mei and B. Yan, Chem. Eng. J., 2023, 475, 146380 CrossRef CAS.
- L. Li, J.-Y. Zou, S.-Y. You and L. Zhang, Inorg. Chem., 2023, 62, 14168–14179 CrossRef CAS PubMed.
- C. Hong, L. Li, J.-Y. Zou, S.-Y. You, E.-L. Wang, L. Zhang, Y.-W. Liu and Y.-L. Huang, Inorg. Chem., 2024, 63, 4697–4706 CrossRef CAS PubMed.
- E.-L. Wang, L. Li, J.-Y. Zou, C. Hong, L. Zhang and S.-Y. You, ACS Appl. Nano Mater., 2024, 7, 17748–17758 CrossRef CAS.
- T. Xia, J. Wang, K. Jiang, Y. Cui, Y. Yang and G. Qian, Chin. Chem. Lett., 2018, 29, 861–864 CrossRef CAS.
- S. Xing and C. Janiak, Chem. Commun., 2020, 56, 12290–12306 RSC.
- J. Lei, P. Zhang, Y.-Y. Xue, J. Xu, H.-P. Li, H.-J. Lv, Y. Wang, S.-N. Li and Q.-G. Zhai, Sep. Purif. Technol., 2022, 283, 120211 CrossRef CAS.
- J.-W. Zhang, X. Li, Y.-Y. Zhang and X.-C. Hu, J. Mol. Struct., 2024, 1309, 138154 CrossRef CAS.
- D.-P. Li, F. Wang, Y. Tian, S.-M. Liu, D.-C. Liu, B. Yang and B.-Q. Xu, Opt. Mater., 2020, 108, 109757 CrossRef CAS.
- Y.-J. Chen, C.-X. Dou, P.-P. Yin, J.-T. Chen, X.-G. Yang, B. Li, L.-F. Ma and L.-Y. Wang, Dalton Trans., 2023, 52, 13872–13877 RSC.
- R. He, Y.-L. Wang, H.-F. Ma, S.-G. Yin and Q.-Y. Liu, Dyes Pigm., 2018, 151, 342–347 CrossRef CAS.
- D. Zhao, X. Rao, J. Yu, Y. Cui, Y. Yang and G. Qian, Inorg. Chem., 2015, 54, 11193–11199 CrossRef CAS PubMed.
- Y. Cui, R. Song, J. Yu, M. Liu, Z. Wang, C. Wu, Y. Yang, Z. Wang, B. Chen and G. Qian, Adv. Mater., 2015, 27, 1420–1425 CrossRef CAS PubMed.
- X. Lian, D. Zhao, Y. Cui, Y. Yang and G. Qian, Chem. Commun., 2015, 51, 17676–17679 RSC.
|
This journal is © The Royal Society of Chemistry 2024 |
Click here to see how this site uses Cookies. View our privacy policy here.