DOI:
10.1039/D4RA06005A
(Review Article)
RSC Adv., 2024,
14, 37752-37762
A review on the multifaceted effects of δ-MnO2 on heavy metals, organic matter, and other soil components
Received
19th August 2024
, Accepted 8th November 2024
First published on 26th November 2024
Abstract
Manganese oxide minerals are prevalent in soils and play a pivotal role in the immobilization of heavy metals and the transformation of materials. Characterized by their low point of zero charge and numerous active adsorption sites, manganese oxides effectively accumulate heavy metals such as lead (Pb) and cadmium (Cd) from contaminated environments. Different manganese oxides, mediated by Mn(II), vary in their capacity to enrich heavy metals. Their distinctive nanostructures can be harnessed to create composite materials that boast enhanced adsorption properties and environmental sustainability. As a highly reactive element in soil, manganese engages in multiple reactions with soil organic components and inorganic ions through processes like oxidation-reduction. This activity aids in the elimination of organic pollutants and facilitates carbon sequestration through various mechanisms. This article explores the metal enrichment capabilities of manganese oxides and their influence on soil physicochemical properties, enhancing our understanding of how these oxides adsorb metallic elements and interact with soil components. Such knowledge is crucial for advancing heavy metal remediation strategies and comprehending the biogeochemical processes within soil environments.
1 Introduction
As soil metal contamination increasingly threatens human health and well-being, there is a growing focus on mitigating the pollution of metal elements. Soils and sediments contain a variety of minerals, among which iron and manganese oxides, along with their hydroxides, represent a significant component. Despite constituting only a fraction of the total solid phase in soils or sediments, these oxides and hydroxides play a crucial role due to their high adsorption capacities, they significantly influence the mobility and bioavailability of trace metal pollutants.1 Manganese oxides, in particular, often form surface coatings on soil particles and nodules. Although present in relatively low concentrations, manganese oxides have a higher redox potential and stronger adsorption affinity compared to iron oxides, enhancing their capacity to accumulate metal elements.2 Table 1 showcases the adsorption capacities of these manganese oxide minerals for heavy metals, emphasizing their importance in soil remediation efforts.5–7
Table 1 Adsorption of heavy metal elements by different manganese oxide minerals3,4
Minerals |
Mineral structure |
Adsorption of heavy metal elements (mmol kg−1) |
Pb |
Co |
Cu |
Cd |
Zn |
Birnessite |
Mn4+ layered structure |
1832 |
1084 |
1268 |
1042 |
1207 |
Cryptomelane |
Mn4+ tunnel structure |
292.8 |
75.5 |
132.5 |
88.8 |
87.1 |
Todorokite |
Mn4+ tunnel structure |
284.3 |
117.3 |
191.4 |
85.1 |
67.3 |
Hausmannite |
Low-valence manganese |
105.3 |
44.4 |
189 |
3.3 |
43.1 |
Manganese oxides, capable of existing in multiple valence states (II, III, IV), form a variety of minerals such as manganite, cryptomelane, and birnessite.3 These minerals are pivotal in enriching soil with metal ions like Pb, Cd, and Co through mechanisms like adsorption and isomorphic substitution.8,9 They also play a role in the migration of various metals (Pb, Cu, Ni, Cr) and metalloids (As) within the soil. However, the role of manganese oxides extends beyond mere enrichment of metal elements. The interaction of manganese oxides with complex soil components—organic matter, oxyanions, and cations—and environmental factors like pH and Eh leads to structural and morphological changes.10,11 These transformations result in various crystalline forms of manganese oxide minerals, influencing the migration, bioavailability, and toxicity of metals in soils.13–15
Moreover, manganese's role as a bio-oxidative metal is vital in the carbon cycle of terrestrial ecosystems, it facilitates the deposition of organic substances within mineral-rich soils and sediments, thereby influencing carbon migration and transformation.16,17 The multifaceted interactions of manganese oxides with soil components are critical in stabilizing soil ecosystems. This has led to significant research interest across various disciplines, highlighting the importance of manganese oxides in environmental science and soil remediation.
2. Enrichment of metal elements by manganese oxides
Long-term heavy metal pollution poses significant risks to arable soil and human health, with cadmium (Cd) and lead (Pb) being common contaminants that infiltrate soil and water bodies through anthropogenic activities.18,19 Manganese oxides are particularly noted for their negatively charged surfaces and distinctive nanostructures, making them valuable for adsorbing, immobilizing, and transferring heavy metals. Manganese oxides can also be combined with other adsorbents and reducing agents to enhance remediation efforts.
δ-MnO2, a primary form of manganese oxide, can evolve into other secondary manganese oxide minerals.3 This transformation is influenced by its interaction with heavy metal ions, which is closely linked to its crystal structure. The structure of δ-MnO2 consists of disordered layers of [MnO6] octahedra and water molecules, within the [MnO6] octahedral layer, vacancies caused by low-valence manganese substitution or manganese deficiency, alongside [Mn(III)O6] octahedra arranged in a shared-apex configuration, contribute to the negative charge of δ-MnO2.20–22 This negative charge is crucial for the electrostatic adsorption of cationic pollutants.23
Birnessite, a type of δ-MnO2 with a low point of zero charge (PZC < 3), is effective in removing metal cations across a broad pH range through adsorption and co-precipitation.24,25 Fig. 1 illustrates the mechanisms of metal ion enrichment by manganese oxides, where adsorption typically involves electrostatic attraction at the solid/liquid interface, and co-precipitation includes both this interfacial binding and the formation of surface precipitates from metal oxides.26 Manganese oxides are integral to the biogeochemical cycles of trace metals and metalloids, significantly influencing the bioavailability and mobility of these elements in the environment.27–29
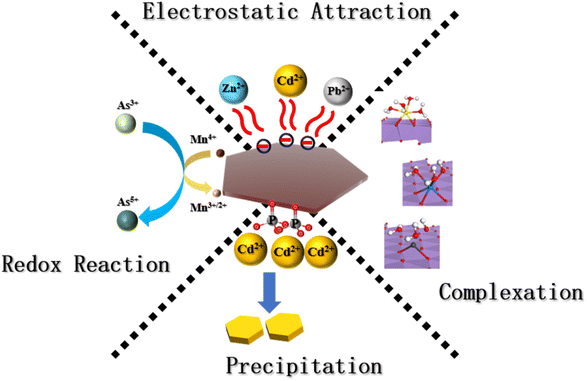 |
| Fig. 1 Manganese oxide enrichment mode for different metal ions. | |
2.1 Enrichment of Cd by manganese oxides
Cd(II) is commonly found in soils as hydroxides or carbonate precipitates. Manganese oxides are highly effective at removing Cd(II) across a wide pH range, significantly reducing its bioavailability and toxicity. Below a pH of 4.0, Cd(II) removal primarily occurs through ion exchange, in the pH range from 5.0 to 6.0, the predominant mechanisms are the formation of outer-sphere and inner–sphere complexes, at a pH of 6.0, Cd(II) binds to the (001) and (002) crystal faces of δ-MnO2 through surface complexation, forming triple corner-sharing (TCS) complexes, achieving a maximum adsorption capacity of 104.17 mg g−1.30–32 Above a pH of 7.0, Cd(II) is completely adsorbed by MnO2.33 The processes of Cd(II) removal through δ-MnO2 involve distinct mechanisms during adsorption and co-precipitation. In the adsorption process, Cd(II) interacts with layered manganese oxide (IV), whereas in co-precipitation, Mn(II) is oxidized to Mn(III) and forms double corner sharing (DCS) complex with Cd(II).34–36 This detailed understanding of the interactions and conditions affecting Cd(II) removal enhances the potential for targeted remediation strategies in contaminated soils.
To enhance the adsorption capacity of manganese oxides for metal ions, researchers have developed innovative combinations with other adsorptive materials. Wang created a composite of todorokite and biochar (BRB), which demonstrated a remarkable adsorption capacity for Cd at 9068 mg kg−1, significantly surpassing that of birnessite alone at 4335 mg kg−1.18 Xia improved the adsorptive properties of MnO2 by incorporating yeast, finding that potassium permanganate (KMnO4) could alter the yeast's cell wall structure at pH values above 4.51, this alteration exposes more functional groups, like carboxyl and amino, enhancing the composite's capacity for Cd(II) adsorption.37–39 Additionally, zeolite-loaded manganese oxide has been utilized as a soil amendment to promote the association of exchangeable Cd with manganese oxide, thereby bolstering the immobilization of Cd and reducing its uptake by plants. The use of this amendment led to significant reductions in Cd content in wheat grains, straw, and roots by 65.0%, 11.7%, and 55.3%, respectively.40,41
The adsorption mechanisms of manganese oxides involve spontaneous redox reactions; δ-MnO2, for instance, can be reduced and dissolved by reductive substances. Qian explored combining δ-MnO2 with S2+ and cysteine, revealing that S2+ could relocate Cd(II) from vacancies to edge sites, forming CdS adsorbed on δ-MnO2 surfaces, at pH 5.5, cysteine induced the release of Cd(II) (52.8–68.6%) previously held in vacancies, while at pH 7.5, it facilitated the formation of Cd-cysteine complexes.6,42,43 Wang reported maintaining a high removal efficiency of Cd at 96% at pH 5 with a thiosulfate/manganate (TS/Mn) molar ratio of 3, demonstrating the potential for tailored approaches to enhance heavy metal remediation in contaminated environments.44,45 Furthermore, the reduction of Fe(II) to Mn(III) impacts Cd(II) adsorption on MnO2 by shifting adsorption sites from vacancies to edge sites, forming DCS and double edge shared (DES) complexes. The introduction of new minerals, such as β-MnOOH and iron oxides, at pH 7.5 with Fe(II) resulted in weaker adsorption capabilities for Cd(II) compared to MnO2.46,47
These studies highlight how integrating manganese oxides with various materials can significantly boost their adsorption capacity for cadmium (Cd). This enhancement not only improves their effectiveness in controlling heavy metal pollution but also broadens their applicability in soil remediation efforts. Such advancements are crucial for developing more efficient strategies to mitigate the impact of heavy metals on the environment.
2.2 Enrichment of Pb by manganese oxides
Manganese oxides are particularly selective in their adsorption of metal ions, with δ-MnO2 demonstrating a preference for Pb over Cu, Ni, and Zn, Pb to form stable Pb–O/OH bonds on the surface of δ-MnO2, which enhances the stability of DES and TCS complexes, significantly increasing the adsorption selectivity for Pb.48 A critical factor influencing this capacity is the Mn average oxidation state (MnAOS) of the oxides, as the MnAOS increases from 3.67 to 3.92, the maximum adsorption capacity for Pb correspondingly rises from 1320 to 2457 mmol kg−1.49,50 X-ray photoelectron spectroscopy (XPS) analysis highlighted that used potassium permanganate (KMnO4) as a catalyst not only boosts the effectiveness of hydrated manganese dioxide (HMO) in removing Pb but also facilitates the oxidation of Pb(II) to Pb(IV) under alkaline conditions, in redox reaction, Mn(II) and Pb(IV) form Mn(OH)2 and PbO2, respectively, with an oxidation efficiency for Pb(IV) reaching 50%.51
Manganese oxides already exhibit strong adsorptive properties for Pb. The strategic combination of manganese oxides with other materials further enhances this efficiency, creating composite materials with synergistic effects. These composites show great promise for the immobilization of Pb in various environmental applications.
For instance, a study produced manganese dioxide biochar (MBR) that demonstrated increased specific surface area and pore volume, XPS analysis identified the formation of Pb–O bonds or combinations with –OH groups as critical mechanisms for Pb(II) removal in MBR.52 Wu developed a novel manganese dioxide modified biochar-based porous hydrogel (MBCG), this three-dimensional structure offers a uniform pore distribution and outstanding adsorptive performance, achieving a Pb adsorption capacity of 70.90 mg g−1, remarkably, MBCG also maintained an 80.5% adsorption efficiency after several recycling processes.53 Layered double hydroxides (LDHs) and layered double oxides (LDOs) are celebrated for their ion-exchange and metal ion adsorption capabilities due to their anionic nature and robust thermal and chemical stability. Huang synthesized two materials, MnO2/MgFe-LDH and MnO2/MgFe-LDO, with Pb(II) adsorption primarily occurring through precipitation, functional group complexation, electrostatic attraction, cation exchange, isomorphic substitution, and memory effects. The MnO2/MgFe-LDO demonstrated exceptional regenerative adsorption capabilities, with an impressive adsorption capacity of 531.86 mg g−1 for Pb(II).54 Cui introduced a new MnO2@polyaniline (PANI) composite, showcasing enhanced adsorption capabilities for Pb(II) compared to the original materials, achieving over 99% removal efficiency at a dosage of 0.5 g L−1.55,56
Poly(m-phenylenediamine) (PmPD) has faced limitations as a heavy metal adsorbent due to its positively charged surface and small surface area. However, Xiong's innovative synthesis of MnO2@PmPD composites has demonstrated notable improvements. The key to these composites' enhanced performance lies in the oxidation products of aniline (a reducing group) present in PmPD, specifically quinone imine and carboxylate groups. These functional groups effectively bind with Pb(II). Through Density Functional Theory (DFT) analysis, it was determined that the (311) crystal plane of MnO2@PmPD serves as the primary adsorption site for Pb(II).65,66
Manganese oxides were combined with substances that possess adsorption capabilities, their ability to adsorb lead (Pb) is significantly enhanced, this synergistic effect also can improve the capability of PmPD to address its deficiencies in metal adsorption. The application of manganese oxides, both individually and in conjunction with other adsorbents, offers substantial promise for effectively stabilizing lead in soil environments. This approach is crucial for reducing lead mobility and toxicity, contributing to safer and healthier soil ecosystems.
2.3 Enrichment of other metal elements by manganese oxides
Manganese oxides are highly effective in adsorbing various metal elements within the soil environment. Notably, Ni and Co could integrate into the structure of δ-MnO2 via isomorphic substitution, replacing Mn4+ within its layers. However, Ni was effectively adsorbed onto the vacancies of the birnessite (001) surface, forming trigonal shared complexes that facilitated a removal rate of over 80% for Ni(II) at a pH of 7,67 birnessite exhibited a marked increase in its adsorption capacity for Co(II) at pH 7.5, reaching about 280 μmol/0.1 g, which was roughly double the capacity observed at pH 6.8,68 Wu's research further revealed that the incorporation of Co(III) into manganese oxides resulted in a reduction of Mn(III) octahedra, which hindered the structural transition from layered to tunnel configurations.69 The presence of Ni during the crystallization process of birnessite could inhibit the incorporation of Co, reducing the number of manganese layers in the co-doped mineral. This interaction demonstrated the competitive dynamics between Ni and Co within manganese oxide structures.48,70
Koppula explored NiCo(BDC)@MnO2 composites maintained excellent stability at high temperatures and showcased remarkable adsorption capacities for Cu2+ (756.82 mg g−1), Cr6+ (111.22 mg g−1), and U6+ (365.25 mg g−1).71
Zn(II) is absorbed onto acid birnessite and δ-MnO2 as endohedral complexes (Zn IV or Zn VI), which also decreases the Mn(III) content within the MnOx layers.72 Interestingly, the adsorption rate for Zn(II) significantly increases from 36% to 96% as the pH value rises from 3 to 9.73 Hawash's synthesis of two types of modified clinoptilolite, using Fe(III) and MnO2, demonstrated through Differential Thermal Analysis (DTA) that crystalline MnO2-clinoptilolite has a stronger adsorption capacity for Zn(II) compared to the amorphous Fe(III) clinoptilolite, reaching an efficiency of 99.65%.74
The removal of arsenic (As) varies depending on its oxidation state and the adsorption dynamics on the surfaces of metal oxides. Todorokite, for instance, can remove As with an efficiency of up to 98% within a pH range of 3 to 9. Wang revealed that As could be removed through endohedral complexes formed within the core–shell structure of MnO2@La(OH)3. Further enhancing the technology, Wen synthesized a MnO2-coated Fe3O4 magnetic flower-like nanocomposite material, which achieved maximum adsorption capacities for As(III) and As(V) of 76.73 mg g−1 and 120.50 mg g−1, respectively.75–77
Manganese can enter the soil through the deposition of throughfall or litterfall. Excessive uptake of soil manganese by plants, beyond their nutritional needs, can impair photosynthesis in species such as sugar maple and various flowers.78 Applying manganese can alter the soil's manganese content as well as the mobility and transformation of heavy metals. Table 2 details the study on the use of various manganese oxides and their composites to stabilize heavy metals in contaminated soils. By incorporating manganese oxide minerals and modified manganese materials, heavy metals can be concentrated in multiple ways (illustrated in Fig. 1) at different locations (shown in Fig. 2). These investigations underscore how manganese oxide minerals and their modified forms can significantly enrich metal elements through a variety of mechanisms at different sites. It has been noted that the valence state of manganese within these oxides plays a critical role in their capacity to enrich metals. Manganese oxides typically present in II/III/IV valence states, with divalent manganese often found in solution and serving as cations that adhere to manganese oxide minerals. This facilitates the transformation of manganese from lower to higher valence states, which subsequently impacts the behavior of heavy metal ions, either fixing or releasing them from manganese oxides.
Table 2 Study on using various manganese oxides and their composites to stabilize heavy metals in contaminated soils
Manganese oxide material |
Treatments |
Effects |
Reference |
Amorphous manganese oxide (AMO) |
Applied to agricultural soil contaminated by a smelting plant, adjust the pH to between 3 and 8 |
pH > 5, MnCO3 forms on the surface of AMO, leading to reductions in the soil concentrations of As, Cu, Pb, and Sb to 20%, 35%, 7%, and 11% of their original levels, respectively |
57 |
AMO, Maghemite, and Magnetite were each applied to the soil surrounding a lead-copper smelting plant |
After applying AMO, the concentrations of Cd, Cu, and Pb decreased by 92%, 92%, and 93%, respectively, which is an order of magnitude greater reduction compared to the other two materials used |
58 |
Natural manganese oxide |
Soil contaminated with Pb |
After applying NMO, the soil showed a significant decrease in water-exchangeable Pb |
59 |
Hydrous manganese oxides (HMO) |
Sludge containing heavy metals and soil from around a smelting plant were used to simultaneously cultivate ryegrass and tobacco |
HMO altered the binding of Cd and Pb in the soil, reducing their transfer from the soil to the soil solution and ultimately decreasing plant uptake of these heavy metals |
60 |
Zeolite-loaded manganese oxide |
Alkaline dryland soil containing Cd |
The application reduced the available Cd content in the soil by 44.3% and increased the available Mn content by 61.9% |
61 |
Fe–Mn modified biochar composite (FMBC) |
Arsenic-contaminated paddy soil |
FMBC application decreased the available arsenic content in the soil by promoting the transformation of non-specifically sorbed and specifically bound arsenic into residual forms and those bound to amorphous and crystalline hydrous oxides |
62 |
Hiol-functionalized graphene oxide/Fe–Mn composite (SGO/Fe–Mn) |
Soil contaminated with Hg |
Applying 0.8% SGO/Fe–Mn can reduce the extractable mercury content by up to 98.9% |
63 |
Birnessite |
Soil contaminated with Cu |
Birnessite lowered the free Cu2+ activity in the soil |
64 |
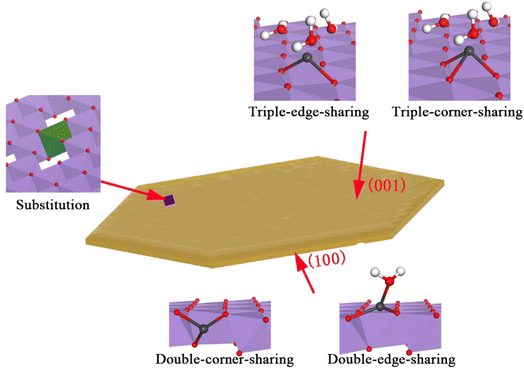 |
| Fig. 2 Schematic representation of the different sites of enrichment of metallic elements by manganese oxide9,23 | |
The subsequent section will delve into the influence of low-valence Mn(II) on the adsorption of metal ions by manganese oxides. This exploration aims to further illuminate the diverse and potent capabilities of different manganese oxide forms to enrich metal ions, offering deeper insights into the mechanisms that govern their interactions and efficiencies in environmental remediation scenarios.
3 Impact of Mn(II) on manganese oxide enrichment of heavy metal
The varying oxidation states of manganese, particularly Mn(III) and Mn(IV), are essential for the catalytic efficiency of manganese oxides, previous studies have demonstrated that Mn(II) can alter the composition and structure of δ-MnO2 minerals.3 A key process in the interaction of Mn(II) with manganese oxides is the comproportionation reaction, where Mn(II) and Mn(IV) combine to form two Mn(III).79 This newly formed Mn(III) then undergoes disproportionation, reverting to Mn(II) and Mn(IV). This continuous cycle of reactions promotes the dynamic exchange among manganese different oxidation states, enhancing the reactivity and functional versatility of manganese oxides.80,81 Higher pH levels facilitate the comproportionation and disproportionation reactions involving Mn(II),72,82 when the pH exceeds 7.5 and Mn(II) is introduced, a noticeable transformation occurs in δ-MnO2, transitioning to minerals like manganite (γ-Mn(III)OOH), feitknechtite (β-Mn(III)OOH), and hausmannite (Mn3O4), which have a greater capacity to stabilize Zn and Ni.11,12,83–85 Research indicated that Mn(II) regulated the ability of manganese oxides to adsorb metal ions such as Co, Sb, and Cd.10,86–89 This change underscores how pH and Mn(II) concentrations critically influence the structural development of manganese oxides. The changes in adsorption capacities resulted not only from structural modifications in the oxides but also from the role of Mn(II) in promoting the regeneration and transformation of manganese oxides into more reactive forms. This dynamic behavior emphasizes the complex interactions within manganese oxides and their potential effectiveness in environmental remediation applications.
3.1 Mn(II) Facilitation of manganese oxide enrichment of metal elements
3.1.1 Ni. Ni adsorption on δ-MnO2 is relatively low, with only about 10% of Ni being adsorbed when the molar ratio of added Ni to δ-MnO2 is approximately 13, primarily because Ni tends to occupy manganese vacancies.25,67 The interaction of pH and Mn(II) further affects Ni binding to manganese oxides.80,90,91 At pH 6.5 and 4.0, Ni(II) is displaced from these vacancies by Mn(III), which is generated through Mn(II)-mediated comproportionation–disproportionation reactions, prompting Ni(II) to relocate from vacancy to edge sites, at pH 7.5, the presence of aqueous Mn(II) triggers the transformation of δ-MnO2 into secondary feitknechtite containing Ni(II), thereby increasing the removal rate of Ni(II) by approximately 30%.92
3.1.2 Zn. When δ-MnO2 adsorbed both Zn(II) and Mn(II), Mn(II) initially occupied the vacancies meant for Zn(II), prompting Zn(II) to shift from the vacancies of todorokite to edge sites.82,93 This process was aided by the bio-oxidation of manganese through surface ion exchange, which released Mn(II) oxidized by Mn(II) oxidase, thereby reducing the competitive adsorption between Mn(II) and Zn(II).94 At pH 7.5, the interaction between Mn(II) and Zn(II) transformed todorokite into a spinel Zn(II)1−xMn(II)xMn(III)2O4 precipitate, significantly increasing the adsorption capacities for Mn(II) and Zn(II) to 800 μmol/0.1 g and 400 μmol/0.1 g, respectively—eight and two times higher than at pH 6.5.82 Moreover, Mn(II) triggered the formation of feitknechtite, manganite, and hausmannite from δ-MnO2, with minimal Zn(II) precipitation in the solution. These newly formed minerals greatly enhanced the fixation of Zn(II).95Mn(II), despite its strong competitive adsorption with Ni and Zn, can prompt the transformation of δ-MnO2 into feitknechtite and other tunnel-structured manganese oxides. This transformation enhances the stabilization of Ni and Zn within these structures.
3.2 Mn(II) inhibition of manganese oxide enrichment of metal elements
3.2.1 Cd. At a pH of 5.5, introducing Mn(II) at concentrations of 2 mM and 8 mM into a system containing δ-MnO2 adsorbing Cd(II) at 5 mM had led to a reduction in Cd(II) adsorption by approximately 10% and 20%, respectively.96 To mitigate the inhibitory effect of Mn(II) on Cd(II) adsorption by δ-MnO2, research had indicated that intermittent introduction of low concentrations of Mn(II) could effectively remove Mn with an efficiency of 97.40%, while causing minimal desorption of Cd(II), which had a release rate of only 0.73%, in contrast, adding high concentrations of Mn(II) directly had reacted with KMnO4 to regenerate MnO2, providing a dual fixation effect that had enhanced the stabilization of Cd(II).97 Natacha found that aeration treatments, which oxidized β-MnOOH and δ-MnO2, had resulted in a decrease in pH and subsequent leaching of Cd(II). On the other hand, anoxic treatments had helped prevent some Mn(II) from oxidizing into MnSiO3 and Mn(II)Al-LDH, thereby better stabilizing Cd(II).98
3.2.2 Co. For Co(II), it could substitute Mn(II) within the secondary β-Mn(III)OOH lattice formed during the reaction between Mn(II) and δ-MnO2, thereby inhibiting the adsorption and reductive transformation of Mn(II) on δ-MnO2.92 At a pH of 7.5, the introduction of Mn(II) (1 mM) had facilitated the reductive transformation of isomorphically substituted Co(III) in δ-MnO2 (0.1 g/L), leading to the formation of feitknechtite containing Co(II), with some Co(II) being released into the solution.8
3.2.3 Sb. Antimony (Sb), a toxic and carcinogenic element, was found in trace amounts in natural environments, typically in the oxidation states Sb(III) and/or Sb(V).99 Previous research had demonstrated that manganese oxides played a crucial role in regulating antimony in soils and sediments. Sb was adsorbed onto the edge sites of manganese oxides through coprecipitation, forming endohedral complexes with an adsorption capacity of up to 342.0 μmol g−1.100–103 In a pH 7.5 δ-MnO2 coprecipitation system with Sb, the addition of 10 mM Mn(II) had transformed δ-MnO2 into manganite and hausmannite within 24 hours, resulting in a composition of 93% and 7%, respectively, the released Mn(II) concentration was around 5 mM, while the release of Sb was almost undetectable.104Additionally, Sb(V) significantly affected the Mn(II)-induced transformation of δ-MnO2. At varying concentrations of aqueous Sb(V) (low: 10 μmol L−1, medium: 200 μmol L−1, and high: 600 μmol L−1), δ-MnO2 was transformed into manganite, hausmannite, and groutite (α-Mn(III)OOH). The removal efficiencies for Sb at these concentrations were 85%, 28%, and 14%, respectively, with the released Mn(II) concentration stabilizing at approximately 2.7 mmol L−1.105
These studies highlighted the intricate interactions between Mn(II) and other metal ions in the presence of manganese oxides, demonstrating that Mn(II) could either facilitate or hinder metal adsorption during the transformation of todorokite into other manganese oxide minerals. This transformation was influenced by several factors, including pH, Mn(II) concentration, and the presence of competing metal ions. Such processes were vital for regulating the mobility of heavy metals, as Mn(II) played a key role in the structural and morphological evolution of manganese oxides within the soil environment. Understanding these dynamic interactions is crucial for managing the geochemical behavior of heavy metals in contaminated soils, offering valuable insights for environmental remediation and pollution control strategies.
4 Interaction between manganese oxides and soil components
Soil, as the most frequently interacted-with layer in human activities, contains a diverse array of minerals and reactive substances formed through sedimentary processes. These soil components mutually influence each other's migration and transformation. Manganese oxide minerals play a key role in the removal and transformation of organic materials, humic substances, and oxyanions within the soil.16 Conversely, these soil components can also impact the structure and functionality of manganese oxides, leading to complex and interdependent interactions between them.
4.1 Potential of manganese oxides for organic matter degradation and stabilization
Manganese was a highly reactive component in soil, actively participating in the removal and transformation of organic matter through redox reactions under both aerobic and anaerobic conditions, making it a key player in carbon sequestration.106–109 The primary mechanism by which manganese oxides adsorbed organic matter mirrored their role in heavy metal removal, relying on electrostatic interactions.109 In neutral environments, manganese oxides carried a negative charge, allowing them to adsorb positively charged functional groups, such as those in proteins.110
The adsorption of organic matter on manganese oxide surfaces was influenced by the specific functional groups present.111 Organic compounds containing hydrophobic groups and nitrogen tended to be more readily adsorbed onto manganese oxides.112,113 Additionally, high molecular weight organic compounds with aromatic structures were preferentially adsorbed and were more likely to undergo oxidation, breaking down into lower molecular weight compounds.114 This selective adsorption and transformation of organic matter by manganese oxides played a crucial role in the cycling and stabilization of organic carbon in soils.
In the context of organic pollutant removal, it was essential to consider both the degradation of these pollutants and the fixation of carbon. Research had shown that during the removal of organic matter by various manganese oxide minerals, dissolved oxygen oxidized Mn(II) to Mn(III), which induced the transformation of δ-MnO2 into minerals such as feitknechtite, manganite, and hausmannite, however, the formation of Mn(III) could block active sites on the manganese oxides, thereby reducing the oxidation of phenol and ultimately inhibiting its degradation.115 Additionally, oxygen competed with fulvic acid (FA) for active sites on MnO2, making anaerobic conditions more effective for removing total organic carbon (TOC), achieving removal rates as high as 79.8%, the transformation of δ-MnO2 into MnOOH could also lead to the preservation of organic carbon by producing insoluble MnCO3 crystals.87,116–119
Humification was another crucial process, significantly influencing the physicochemical properties of soil and supporting plant growth and development.119 Manganese oxides, with their high redox potential, held promise for enhancing humification processes. MnO2 and its reduction products (MnOOH and Mn(II)) could catalyze the reduction of fulvic-like acids (FLAs) and humic-like acids (HLAs), leading to the production of CO2.120 Research by Qi found that δ-MnO2 could catalyze the further condensation of unstable substances into highly aromatic complex substances, thereby increasing the degree of humification of FA and improving the bioavailability of HLAs.17,121 Similarly, Li's research on methanol oxidation using Mn2O3 and δ-MnO2 revealed that the comproportionation–disproportionation reactions within these manganese oxides could enhance lattice oxygen transfer and Mn(IV) oxidation, thereby boosting the efficiency of methanol oxidation.122 These findings underscored the multifaceted role of manganese oxides in both pollutant degradation and the enhancement of soil organic matter stability.
These studies illustrate the significant role of manganese oxides in the removal of organic substances and in the transformation and fixation of carbon, underlining their importance in soil management and environmental remediation strategies.
4.2 Influence of cations and anions on the structure and functionality of manganese oxides
4.2.1 Cationic. In soil environments, the presence of cations had a profound impact on the structure of manganese oxides, and these structural changes, in turn, affected their adsorption and oxidation capabilities. For example, Na(I) and Ca(II) promoted the formation of Mn(III) and its orderly distribution within the Mn octahedral layers. Conversely, H(I) and Ni(II) led to the creation of vacancies, which enhanced the competitive adsorption of Ni(II) and Mn(II) at these sites.123 Additionally, increasing concentrations of K(I) increased the amount of mobile oxygen on the surface of MnO2, thereby boosting the oxidative activity of δ-MnO2 toward formaldehyde (HCHO).124The presence of NH4+ in mineral resource utilization presented challenges due to its removal difficulty and the potential for secondary pollution. Numerous studies had investigated the transformation and removal processes of NH4+ within manganese oxide minerals, revealing that the average oxidation state of manganese (MnAOS) and pH were critical factors in its transformation and removal.125 NH4+ was more readily released under alkaline conditions than under acidic ones, with electrostatic interactions and ion exchange between OH- and NH4+ being the primary mechanisms for its removal. NH4+ could be oxidized by Mn(IV) to NO3− and N2, with Mn present in forms such as MnO2, Mn2O3, MnOOH, and Mn3O4.126–128 The removal rate of NH4+ was also influenced by coexisting cations in the solution, with the effectiveness ranking as Ca2+ > K+ > Mg2+ > Na+.129
4.2.2 Oxygenated anion. Oxygenated anion in soil played a crucial role in regulating the crystalline growth of manganese oxides and their ability to degrade various substances. Research had shown that oxyanions such as phosphate, sulfate, and silicate could adsorb at the edge sites of MnO6 octahedra, thereby inhibiting the lateral growth of the MnO6(100) crystal face, with phosphate exerting the most significant influence.14,130 The interactions between these oxyanions and manganese could mutually affect the binding capabilities of manganese oxides. Negatively charged polycarboxylic compounds tended to bind preferentially at edge sites, which enhanced the stabilization ability of δ-MnO2 for Mn(III).109,131–134 Additionally, the involvement of Mn(II) in proton exchange could reduce the negative surface charge of MnO2, thereby increasing its capacity to remove oxyanions such as phosphate and silicate.85The adsorption of sulfate (SO4−) and hydroxyl radicals (·OH) on manganese oxides could also facilitate the degradation of organic substances.135 Density Functional Theory (DFT) calculations had indicated that the α-MnO2 (211 crystal face) could more effectively activate peroxymonosulfate (PMS), leading to the generation of SO4− and ·OH radicals. Consequently, manganese oxides with higher crystallinity, such as α-, β-, and γ-MnO2, exhibited stronger capabilities for degrading organic materials compared to δ-MnO2.136,137
These findings underscore the complex chemistry between manganese oxides and both cations and anions in soil, which profoundly impacts their environmental roles and their applications in soil remediation and management.
4.3 Microbial promotion of the manganese cycle
Microorganisms, including bacteria, fungi, algae, and diatoms, played a crucial role in biogeochemical cycles through a variety of enzymatic and non-enzymatic pathways.138 As key components of the soil ecosystem, these microorganisms significantly influenced the mineralization processes of natural manganese oxides.139 Microbes utilized enzymes to promote the production of extracellular superoxide radicals (in bacteria) and reactive oxygen species (in fungi and algae), which indirectly oxidized Mn(II) to Mn(III), through comproportionation–disproportionation reactions, these processes generated Mn(II) and Mn(IV), with superoxide-producing microbes potentially serving as key drivers of mineral formation and transformation in soil environments.140–142 The microbial mediation of mineralization could occur at the micrometer scale and proceeded at a high rate, highlighting the significant role of bacteria and fungi in the reduction of Mn(IV).143
Microorganisms indirectly participated in the mineralization of Mn(II) and the reduction of Mn(IV), acting as catalytic agents that drove the cycling of manganese across various oxidation states within the environment.144 This microbial activity not only facilitated the transformation and cycling of manganese but also enhanced the biogeochemical cycling of other essential nutrients and metals within the soil, thereby contributing to soil fertility and overall ecosystem functioning.
5 Conclusions and future perspective
Manganese oxides play a crucial role in maintaining the normal operation of the soil layer. They act as catalysts for redox reactions, which are vital for nutrient cycling and the degradation of contaminants. Manganese oxides can oxidize organic contaminants and reduce potentially toxic metal ions, thereby mitigating their mobility and bioavailability in the soil environment. Their high reactivity also allows manganese oxides to interact with soil organic matter, leading to the formation of stable organo–mineral complexes that can sequester carbon and metals, further influencing soil fertility and structure.
Additionally, manganese oxides can interact with other soil minerals to affect soil pH, electrochemical properties, and cation exchange capacity, all of which are fundamental to soil health and plant growth. The presence of manganese oxides can also enhance the microbial degradation of organic compounds by serving as electron acceptors, thereby promoting biogeochemical cycles within the soil.
Thus, understanding the interactions between manganese oxides and soil components is essential for developing strategies for soil management and remediation, as well as for predicting the environmental fate of pollutants in the soil matrix. These interactions highlight the importance of manganese oxides in environmental geochemistry and their potential applications in sustainable agriculture and environmental protection.
Data availability
This article is a review and does not contain any new data. All information is derived from previously published articles, which are cited accordingly in the reference section. Data sharing is not applicable to this article as no new data were created or analyzed in this study. All data discussed are from previously published research articles.
Conflicts of interest
The authors have declared there exist no competing interests.
Acknowledgements
Thanks to the support of Yunnan Provincial Basic Research Program No. 202201AU070178, Yunnan Agricultural University Research Initiation Project No. F2022-07, The National Natural Science Foundation of China No. 42367005, Yunnan Fundamental Research Projects No. 202301AU070122, 202401AT070044 and Yunnan Academy of Agricultural Sciences pre-research project No. 2024KYZX-04.
References
- M. Sathish, C. L. Peacock, S. Franziska, G. Anja, M. Dirk, K. Erika and B. Georg, Chem. Geol., 2015, 402, 6–17 CrossRef.
- D. Gasparatos, Environ. Chem. Lett., 2013, 11, 1–9 CrossRef CAS.
- E. J. Elzinga, Environ. Sci. Technol., 2011, 45, 6366–6372 CrossRef CAS PubMed.
- X. H. Feng, L. M. Zhai, W. F. Tan, F. Liu and J. Z. He, Environ. Pollut., 2006, 147, 366–373 CrossRef PubMed.
- L. D. Puppa, M. Komárek, F. Bordas, J.-C. Bollinger and E. Joussein, J. Colloid Interface Sci., 2013, 399, 99–106 CrossRef PubMed.
- A. A. Simanova, K. D. Kwon, S. E. Bone, J. R. Bargar, K. Refson, G. Sposito and J. Peña, Geochim. Cosmochim. Acta, 2015, 164, 191–204 CrossRef CAS.
- Q. Wang, P. Yang and M. Zhu, Geochim. Cosmochim. Acta, 2019, 250, 292–310 CrossRef CAS.
- Z. Huaiyan, F. Xionghan, L. Sungsik, R. Benjamin and E. J. Elzinga, Chem. Geol., 2023, 618, 121281 CrossRef.
- H. Yin, J. Sun, X. Yan, X. Yang, X. Feng, W. Tan, G. Qiu, J. Zhang, M. Ginder-Vogel and F. Liu, Environ. Pollut., 2020, 256, 113462 CrossRef CAS PubMed.
- Z. Huaiyan, Z. Mengqiang, L. Wei, E. E. J, V. Mario, L. Fan, Z. Jing, F. Xionghan and S. D. L, Environ. Sci. Technol., 2016, 50, 1750–1758 CrossRef PubMed.
- J. P. Lefkowitz, A. A. Rouff and E. J. Elzinga, Environ. Sci. Technol., 2013, 47, 10364–10371 CrossRef CAS PubMed.
- Y. Peng, W. Ke, B. K. A, X. Wenqian, W. Qian, M. Dong, W. Juan and Z. Mengqiang, Environ. Sci. Technol., 2021, 55, 3419–3429 CrossRef PubMed.
- P. Jaya and P. Tarasankar, Nanoscale, 2015, 7, 14159–14190 RSC.
- W. Qian, L. Xianya, X. Wenqian, R. Yang, K. J. Livi and Z. Mengqiang, Inorg. Chem., 2016, 55, 10248–10258 CrossRef PubMed.
- G. R. Aiken, H.-K. Heileen and J. N. Ryan, Environ. Sci. Technol., 2011, 45, 3196–3201 CrossRef CAS PubMed.
- K. Markus, I. C. Bourg, E. K. Coward, C. M. Hansel, S. C. B. Myneni and N. Naoise, Nat. Rev. Earth Environ., 2021, 2, 402–421 CrossRef.
- O. W. Moore, C. Lisa, W. Clare, J. A. Bradley, B. Peyman, B. J. W. Mills, W. B. Homoky, X. KeQing, A. W. Bray, B. J. Fisher, K. Majid, K. Burkhard, A. W. Dale and C. L. Peacock, Nature, 2023, 621, 312–317 CrossRef CAS.
- H. Wang, C. Peng, Z. Yong-Guan, C. Kuang and S. Guo-Xin, Environ. Sci. Pollut. Res. Int., 2019, 26, 8575–8584 CrossRef CAS.
- S. F. Qing, C. J. Dong, T. Zhong, L. W. Ju, H. X. Yuan and Z. F. Jie, Plant Soil, 2018, 433, 377–389 CrossRef.
- X. Liang, J. E. Post, B. Lanson, X. Wang, M. Zhu, F. Liu, W. Tan, X. Feng, G. Zhu, X. Zhang and J. J. D. Yoreo, Environ. Sci.: Nano, 2019, 7, 238–249 RSC.
- M. Villalobos, B. Toner, J. Bargar and G. Sposito, Geochim. Cosmochim. Acta, 2003, 67, 2649–2662 CrossRef CAS.
- J. E. Post, Proc. Natl. Acad. Sci. U. S. A., 1999, 96, 3447–3454 CrossRef CAS PubMed.
- K. D. Kwon, K. Refson and G. Sposito, Geochim. Cosmochim. Acta, 2013, 101, 222–232 CrossRef CAS.
- M. Alain and S. N. Steinmann, ACS Earth Space Chem., 2021, 5, 66–76 CrossRef.
- C. A. J. Appelo and D. Postma, Geochim. Cosmochim. Acta, 2000, 64, 3931 CrossRef CAS.
- G. Lee, J. M. Bigham and G. Faure, Appl. Geochem., 2002, 17, 569–581 CrossRef CAS.
- Å. Agneta, B. Lars, B. I. A, N. G. F, N. Monica and S. Staffan, Environ. Health Perspect., 2014, 122, 431–438 CrossRef PubMed.
- Z. Lingxiao, G. Cheng, C. Chuan, Z. Wenwen, H. Xin-Yuan and Z. Fang-Jie, Environ. Sci. Technol., 2020, 54, 10100–10108 CrossRef PubMed.
- R. Liu, H. Liu, Z. Qiang, J. Qu, G. Li and D. Wang, J. Colloid Interface Sci., 2008, 331, 275–280 CrossRef.
- C. L. Chen and X. K. Wang, Appl. Geochem., 2007, 22, 436–445 CrossRef CAS.
- K. Eun-Ju, L. Chung-Seop, C. Yoon-Young and C. Yoon-Seok, ACS Appl. Mater. Interfaces, 2013, 5, 9628–9634 CrossRef PubMed.
- X. Huang, T. Chen, X. Zou, M. Zhu, D. Chen and M. Pan, Int. J. Environ. Res. Public Health, 2017, 14, 1145 CrossRef PubMed.
- M. I. Zaman, S. Mustafa, S. Khan and B. Xing, J. Colloid Interface Sci., 2009, 330, 9–19 CrossRef CAS PubMed.
- S. Kohei, K. Tatsuya, F. Shigeshi and T. Chiharu, Chem. Geol., 2020, 550, 119744 CrossRef.
- A. A. Mamun, M. Morita, M. Matsuoka and C. Tokoro, J. Hazard. Mater., 2017, 334, 142–149 CrossRef PubMed.
- Y. Wang, X. Feng, M. Villalobos, W. Tan and F. Liu, Chem. Geol., 2012, 292–293, 25–34 CrossRef CAS.
- Y. Zhang, J. Zhu, L. Zhang, Z. Zhang, M. Xu and M. Zhao, Desalination, 2011, 278, 42–49 CrossRef CAS.
- Y. Xia, L. Meng, Y. Jiang, Y. Zhang, X. Dai and M. Zhao, Chem. Eng. J., 2015, 259, 927–935 CrossRef CAS.
- Y. Ren, N. Yan, Q. Wen, Z. Fan, T. Wei, M. Zhang and J. Ma, Chem. Eng. J., 2011, 175, 1–7 CrossRef CAS.
- J. Lin, Y. Zhan, Z. Zhu and Y. Xing, J. Hazard. Mater., 2011, 193, 102–111 CrossRef CAS PubMed.
- W. Weihua, L. Tao, L. Lihu, Y. Xiong, S. Xuecheng, Q. Guohong, H. Dangling and Z. Dongmei, J. Hazard. Mater., 2021, 419, 126464 CrossRef PubMed.
- Q. Sun, P.-X. Cui, M. Zhu, T.-T. Fan, S. T. Ata-Ul-Karim, J.-H. Gu, S. Wu, D.-M. Zhou and Y.-J. Wang, Environ. Int., 2019, 130, 104932 CrossRef CAS PubMed.
- S. Qian, C. Peixin, W. Song, L. Cun, F. Tingting, A. M. Eduardo, C. Hu, H. Meiying, Z. Dongmei and W. Yujun, Environ. Sci. Technol., 2020, 54, 14955–14963 CrossRef.
- P. Wang, H. Cheng, J. Ding, J. Ma, J. Jiang, Z. Huang, J. Li, S. Pang, C. Guan and Y. Gao, Chem. Eng. J., 2020, 380, 122585 CrossRef CAS.
- Y. Liu, L. Wang, X. Wang, Z. Huang, C. Xu, T. Yang, X. Zhao, J. Qi and J. Ma, Water Res., 2017, 124, 149–157 CrossRef CAS PubMed.
- C. M. van Genuchten and J. Peña, Environ. Sci.: Processes Impacts, 2016, 18, 1030–1041 RSC.
- Q. Sun, P. X. Cui, T. T. Fan, S. Wu, M. Zhu, M. E. Alves, D. M. Zhou and Y. J. Wang, Chem. Eng. J., 2018, 353, 167–175 CrossRef CAS.
- A. Manceau, M. Lanson and Y. Takahashi, Am. Mineral., 2014, 99, 2068–2083 CrossRef.
- W. Zhao, W. Tan, M. Wang, J. Xiong, F. Liu, L. Weng and L. K. Koopal, Environ. Sci. Technol., 2018, 52, 10522–10531 CrossRef CAS PubMed.
- W. Zhao, X. Feng, W. Tan, F. Liu and S. Ding, J. Environ. Sci., 2009, 21, 520–526 CrossRef CAS PubMed.
- X. Qi and F. C. Xie, Chem. Eng. J., 2018, 351, 22–30 CrossRef CAS.
- J. Liang, X. M. Li, Z. G. Yu, G. M. Zeng, Y. Luo, L. B. Jiang, Z. X. Yang, Y. Y. Qian and H. P. Wu, ACS Sustainable Chem. Eng., 2017, 5, 5049–5058 CrossRef CAS.
- Z. Y. Wu, X. X. Chen, B. L. Yuan and M. L. Fu, Chemosphere, 2020, 239, 124745 CrossRef CAS PubMed.
- Y. X. Huang, X. P. Luo, C. M. Liu, S. H. You, S. Rad and L. T. Qin, RSC Adv., 2023, 13, 19288–19300 RSC.
- C. Cui, X. H. Sun, C. Q. Zhou, Y. W. Liu, H. X. Xiong, Y. N. Li and J. Han, Colloids Surf., A, 2021, 616, 126336 CrossRef CAS.
- J. Han, J. Dai and R. Guo, J. Colloid Interface Sci., 2011, 356, 749–756 CrossRef CAS PubMed.
- V. Ettler, Z. Tomásová, M. Komárek, M. Mihaljevic, O. Sebek and Z. Michálková, J. Hazard. Mater., 2015, 286, 386–394 CrossRef CAS.
- Z. Michálková, M. Komárek, H. Sillerová, L. Della Puppa, E. Joussein, F. Bordas, A. Vanek, O. Vanek and V. Ettler, J. Environ. Manage., 2014, 146, 226–234 CrossRef.
- C. M. McCann, N. D. Gray, J. Tourney, R. J. Davenport, M. Wade, N. Finlay, K. A. Hudson-Edwards and K. L. Johnson, Chemosphere, 2015, 138, 211–217 CrossRef CAS.
- M. J. Mench, V. L. Didier, M. Löffler, A. Gomez and P. Masson, J. Environ. Qual., 1994, 23, 58–63 CrossRef CAS.
- W. Wang, T. Lu, L. Liu, X. Yang, X. Sun, G. Qiu, D. Hua and D. Zhou, J. Hazard. Mater., 2021, 419, 126464 CrossRef CAS PubMed.
- L. N. Lin, Z. Y. Li, X. W. Liu, W. W. Qiu and Z. G. Song, Environ. Pollut., 2019, 244, 600–607 CrossRef CAS.
- Y. Huang, M. Wang, Z. Li, Y. Gong and E. Y. Zeng, J. Hazard. Mater., 2019, 373, 783–790 CrossRef CAS PubMed.
- M. B. McBride and C. E. MartÍnez, Environ. Sci. Technol., 2000, 34, 4386–4391 CrossRef CAS.
- T. Xiong, X. Z. Yuan, H. Wang, L. B. Jiang, Z. B. Wu, H. Wang and X. Y. Cao, J. Hazard. Mater., 2020, 389, 122154 CrossRef CAS PubMed.
- T. Rong, L. Qin, D. Liang, C. Hao and Z. Jianping, Environ. Technol., 2012, 33, 341–348 CrossRef PubMed.
- C. L. Peacock and D. M. Sherman, Chem. Geol., 2006, 238, 94–106 CrossRef.
- P. Yang, J. W. Wang, S. Wang, C. Y. Yang, P. Y. Zhao, B. F. Huang, Q. Wang and H. F. Wang, ACS Omega, 2022, 7, 37452–37464 CrossRef CAS.
- Z. K. Wu, C. L. Peacock, B. Lanson, H. Yin, L. R. Zheng, Z. J. Chen, W. F. Tan, G. H. Qiu, F. Liu and X. H. Feng, Geochim. Cosmochim. Acta, 2019, 246, 21–40 CrossRef CAS.
- H. Yin, H. Li, Y. Wang, M. Ginder-Vogel, G. H. Qiu, X. H. Feng, L. R. Zheng and F. Liu, Chem. Geol., 2014, 381, 10–20 CrossRef CAS.
- S. Koppula, P. Jagasia, M. K. Panchangam and S. B. M. Surya, J. Solid State Chem., 2022, 312, 123168 CrossRef CAS.
- S. L. Zhao, Q. Wang, J. Y. Sun, O. J. Borkiewicz, R. X. Huang, E. M. Saad, B. Fields, S. Chen, M. Q. Zhu and Y. Z. Tang, Chem. Geol., 2018, 493, 234–245 CrossRef CAS.
- Z. Wang, C. Peacock, K. D. Kwon, X. Y. Gu, X. H. Feng and W. Li, Geochim. Cosmochim. Acta, 2023, 348, 68–84 CrossRef CAS.
- H. B. I. Hawash, E. Chmielewska, Z. Netriová, J. Majzlan, H. Pálková, P. Hudec and R. Sokolík, J. Environ. Chem. Eng., 2018, 6, 6489–6503 CrossRef CAS.
- Y. L. Wang, C. Guo, L. Zhang, X. H. Lu, Y. H. Liu, X. H. Li, Y. Y. Wang and S. F. Wang, Int. J. Environ. Res. Public Health, 2022, 19 DOI:10.3390/ijerph191710649.
- Y. Zhang, B. C. Zhao, C. J. Wang, Y. Y. Huang, X. Liu, R. K. Wang and C. B. Wang, J. Hazard. Mater., 2023, 459, 132079 CrossRef CAS PubMed.
- Z. P. Wen, Y. L. Zhang, Y. Wang, L. N. Li and R. Chen, Chem. Eng. J., 2017, 312, 39–49 CrossRef CAS.
- S. B. Horsley, R. P. Long, S. W. Bailey, R. A. Hallett and T. J. Hall, Can. J. For. Res., 2000, 30, 1365–1378 CrossRef CAS.
- J. R. Bargar, B. M. Tebo, U. Bergmann, S. M. Webb, P. Glatzel, V. Q. Chiu and M. Villalobos, Am. Mineral., 2015, 90, 143–154 CrossRef.
- E. J. Elzinga and A. B. Kustka, Environ. Sci. Technol., 2015, 49, 4310–4316 CrossRef CAS PubMed.
- E. J. Elzinga, Environ. Sci. Technol., 2016, 50, 8670–8677 CrossRef CAS PubMed.
- J. P. Lefkowitz and E. J. Elzinga, Environ. Sci. Technol., 2015, 49, 4886–4893 CrossRef CAS PubMed.
- Q. Wang, P. Yang and M. Q. Zhu, Geochim. Cosmochim. Acta, 2019, 250, 292–310 CrossRef CAS.
- P. Yang, S. Lee, J. E. Post, H. F. Xu, Q. Wang, W. Q. Xu and M. Q. Zhu, Geochim. Cosmochim. Acta, 2018, 240, 173–190 CrossRef CAS.
- Q. Z. Li, R. Pokharel, L. Zhou, M. Pasturel and K. Hanna, Environ. Sci.: Nano, 2020, 7, 4022–4031 RSC.
- M. A. G. Hinkle, E. D. Flynn and J. G. Catalano, Geochim. Cosmochim. Acta, 2016, 192, 220–234 CrossRef CAS.
- J. E. Johnson, P. Savalia, R. Davis, B. D. Kocar, S. M. Webb, K. H. Nealson and W. W. Fischer, Environ. Sci. Technol., 2016, 50, 4248–4258 CrossRef CAS PubMed.
- E. Silvester, A. Manceau and V. A. Drits, Am. Mineral., 2015, 82, 962–978 Search PubMed.
- D. R. Learman, S. D. Wankel, S. M. Webb, N. Martinez, A. S. Madden and C. M. Hansel, Geochim. Cosmochim. Acta, 2011, 75, 6048–6063 CrossRef CAS.
- A. Manceau, C. Tommaseo, S. Rihs, N. Geoffroy, D. Chateigner, M. Schlegel, D. Tisserand, M. A. Marcus, N. Tamura and Z.-S. Chen, Geochim. Cosmochim. Acta, 2005, 69, 4007–4034 CrossRef CAS.
- M. A. Marcus, A. Manceau and M. Kersten, Geochim. Cosmochim. Acta, 2004, 68, 3125–3136 CrossRef CAS.
- J. P. Lefkowitz and E. J. Elzinga, Chem. Geol., 2017, 466, 524–532 CrossRef CAS.
- M. A. G. Hinkle, K. G. Dye and J. G. Catalano, Environ. Sci. Technol., 2017, 51, 3187–3196 CrossRef CAS PubMed.
- J. N. Chang, Y. Tani, H. Naitou, N. Miyata, F. Tojo and H. Seyama, Chem. Geol., 2014, 383, 155–163 CrossRef CAS.
- S. L. Zhao, Y. A. González-Valle, E. J. Elzinga, E. M. Saad and Y. Z. Tang, Chem. Geol., 2018, 492, 12–19 CrossRef CAS.
- Q. Sun, P. X. Cui, M. Zhu, T. T. Fan, S. T. Ata-Ul-Karim, J. H. Gu, S. Wu, D. M. Zhou and Y. J. Wang, Environ. Int., 2019, 130, 104932 CrossRef CAS PubMed.
- J. Zhang and F. C. Xie, Environ. Sci. Pollut. Res., 2022, 29, 36295–36312 CrossRef CAS PubMed.
- N. Van Groeningen, I. Christl and R. Kretzschmar, Environ. Sci. Technol., 2021, 55, 1650–1658 CrossRef CAS PubMed.
- M. Filella, N. Belzile and Y. W. Chen, Earth-Sci. Rev., 2002, 57, 125–176 CrossRef CAS.
- X. Wang, M. He, C. Lin, Y. Gao and L. Zheng, Geochemistry, 2012, 72, 41–47 CrossRef CAS.
- Q. Sun, C. Liu, M. E. Alves, S. T. Ata-Ul-Karim, D. M. Zhou, J. Z. He, P. X. Cui and Y. J. Wang, J. Eng., 2018, 473, DOI:10.1016/j.cej.2018.02.091.
- N. Belzile, Y.-W. Chen and Z. Wang, Chem. Geol., 2001, 174, 379–387 CrossRef CAS.
- X. Sun, B. Li, F. Han, E. Xiao, Q. Wang, T. Xiao and W. Sun, Environ. Pollut., 2019, 252, 1872–1881 CrossRef CAS PubMed.
- N. Karimian, S. G. Johnston and E. D. Burton, J. Hazard. Mater., 2021, 404, 124227 CrossRef CAS PubMed.
- N. Karimian, K. Hockmann, B. Planer-Friedrich, S. G. Johnston and E. D. Burton, Environ. Sci. Technol., 2021, 55, 9854–9863 CrossRef CAS PubMed.
- C. K. Remucal and M. Ginder-Vogel, Environ. Sci.: Processes Impacts, 2014, 16, 1247–1266 RSC.
- J. W. Stuckey, G. Christopher, W. Jian, L. A. Kaplan, V.-E. Prian, T. P. Beebe and D. L. Sparks, Geochem. Trans., 2018, 19, 6 CrossRef PubMed.
- J. Chorover and M. K. Amistadi, Geochim. Cosmochim. Acta, 2001, 65, 95–109 CrossRef CAS.
- Q. Wang, P. Yang and M. Zhu, Environ. Sci. Technol., 2018, 52, 1844–1853 CrossRef CAS PubMed.
- M. Rabe, D. Verdes and S. Seeger, Adv. Colloid Interface Sci., 2010, 162, 87–106 CrossRef PubMed.
- W. Zhao, H. F. Cheng and S. Taos, Environ. Sci. Technol., 2020, 54, 1475–1483 CrossRef CAS PubMed.
- S. Allard, L. Gutierrez, C. Fontaine, J. P. Croué and H. Gallard, Sci. Total Environ., 2017, 583, 487–495 CrossRef CAS PubMed.
- K. Johnson, G. Purvis, E. Lopez-Capel, C. Peacock, N. Gray, T. Wagner, C. März, L. Bowen, J. Ojeda, N. Finlay, S. Robertson, F. Worrall and C. Greenwell, Nat. Commun., 2015, 6, 7628 CrossRef CAS PubMed.
- E. L. Trainer, M. Ginder-Vogel and C. K. Remucal, Environ. Sci. Technol., 2021, 55, 12084–12094 CrossRef CAS PubMed.
- E. Hu, S. Y. Pan, W. Z. Zhang, X. L. Zhao, B. Liao and F. He, Environ. Sci.: Processes Impacts, 2019, 21, 2118–2127 RSC.
- Q. Q. Li, X. C. Huang, G. J. Su, M. H. Zheng, C. H. Huang, M. J. Wang, C. Y. Ma and D. Wei, Environ. Sci. Technol., 2018, 52, 13351–13360 CrossRef CAS PubMed.
- Z. Q. Wang, H. Z. Jia, H. R. Zhao, R. Zhang, C. Zhang, K. C. Zhu, X. T. Guo, T. C. Wang and L. Y. Zhu, Environ. Sci. Technol., 2022, 56, 9806–9815 CrossRef CAS PubMed.
- Z. L. Chi, X. Y. Zhao, Y. L. Chen, J. L. Hao, G. H. Yu, B. A. Goodman and G. M. Gadd, Environ. Microbiol., 2021, 23, 893–907 CrossRef CAS PubMed.
- W. G. Sunda and D. J. Kieber, Nature, 1994, 367, 62–64 CrossRef CAS.
- Y. C. Zhang, D. B. Yue, X. Wang and W. F. Song, J. Environ. Sci., 2019, 77, 167–173 CrossRef CAS PubMed.
- H. S. Qi, A. Zhang, Z. Du, J. Q. Wu, X. M. Chen, X. Zhang, Y. Zhao, Z. M. Wei, X. Y. Xie, Y. Li and M. Ye, Waste Manage., 2021, 128, 16–24 CrossRef CAS PubMed.
- W. C. Li, X. Y. Wen, X. J. Wang, J. Li, E. B. Ren, Z. F. Shi, C. M. Liu, D. Q. Mo and S. P. Mo, Mol. Catal., 2021, 514, 111847 CrossRef CAS.
- Z. Mengqiang, G.-V. Matthew, P. S. J, F. Xiong-Han and S. D. L, Environ. Sci. Technol., 2010, 44, 4465–4471 CrossRef PubMed.
- R. M. Fang, Q. Y. Feng, H. B. Huang, J. Ji, M. He, Y. J. Zhan, B. Y. Liu and D. Y. C. Leung, Catal. Today, 2019, 327, 154–160 CrossRef CAS.
- Y. Cheng, T. L. Huang, Y. K. Sun and X. X. Shi, Chem. Eng. J., 2017, 322, 82–89 CrossRef CAS.
- J. F. Zhong, J. M. Liu, R. Hu, D. D. Pan, S. C. Shao and X. W. Wu, Bioresour. Technol., 2023, 377, 128957 CrossRef CAS PubMed.
- Y. Song, Q. Pan, H. Z. Lv, D. Yang, Z. M. Qin, M. Y. Zhang, X. Q. Sun and X. X. Liu, Angew. Chem., Int. Ed., 2021, 60, 5718–5722 CrossRef CAS PubMed.
- J. C. Shu, Y. H. Wu, Y. L. Deng, T. Y. Lei, J. F. Huang, Y. H. Han, X. R. Zhang, Z. S. Zhao, Y. F. Wei and M. J. Chen, Sep. Purif. Technol., 2021, 270, 118798 CrossRef CAS.
- L. Zhang, J. L. Wang, H. X. Qiao, F. X. Liu and Z. S. Fu, J. Cleaner Prod., 2020, 272, 123055 CrossRef CAS.
- L. S. Balistrieri and T. T. Chao, Geochim. Cosmochim. Acta, 1990, 54, 739–751 CrossRef CAS.
- T. Toshihiro, H. Kazuhito and N. Ryuhei, J. Am. Chem. Soc., 2012, 134, 18153–18156 CrossRef PubMed.
- M. Zhu, C. L. Farrow, J. E. Post, K. J. T. Livi, S. J. L. Billinge, M. Ginder-Vogel and D. L. Sparks, Geochim. Cosmochim. Acta, 2012, 81, 39–55 CrossRef CAS.
- A.-C. Gaillot, D. Flot, V. A. Drits, A. Manceau, B. Manfred and B. Lanson, Chem. Mater., 2003, 15, 4666–4678 CrossRef CAS.
- D. Banerjee and H. W. Nesbitt, Geochim. Cosmochim. Acta, 2001, 65, 1703–1714 CrossRef CAS.
- H. Ouyang, C. Wu, X. H. Qiu, K. Tanaka, T. Ohnuki and Q. Q. Yu, Environ. Res., 2023, 217, 114874 CrossRef CAS PubMed.
- Z. G. Zhou, H. M. Du, Z. H. Dai, Y. Mu, L. L. Tong, Q. J. Xing, S. S. Liu, Z. M. Ao and J. P. Zou, Chem. Eng. J., 2019, 374, 170–180 CrossRef CAS.
- J. Z. Huang, Y. F. Dai, K. Singewald, C. C. Liu, S. Saxena and H. C. Zhang, Chem. Eng. J., 2019, 370, 906–915 CrossRef CAS.
- L. Hui, S. Fernanda, B. Kristen and H. Elizabeth, Environ. Sci. Technol., 2021, 55, 12136–12152 CrossRef PubMed.
- P. Márta, G. Ildikó, F. Krisztián, H. Henrietta, P.-M. Elemér and B. J. Carlos, Front. Microbiol., 2019, 10, 2731 CrossRef PubMed.
- D. R. Learman, B. M. Voelker, A. I. Vazquez-Rodriguez and C. M. Hansel, Nat. Geosci., 2011, 4, 95–98 CrossRef CAS.
- T. Yuanzhi, Z. C. A, S. C. M and H. C. M, Environ. Microbiol., 2013, 15, 1063–1077 CrossRef PubMed.
- D. L. Chaput, A. J. Fowle, S. Onyou, D. Kelly, C. M. Hanse and C. M. Santelli, Sci. Rep., 2019, 9, 18244 CrossRef CAS PubMed.
- M. Polgári and I. Gyollai, Ore Geol. Rev., 2021, 136, 104203 CrossRef.
- C. M. Hanse, Adv. Microb. Physiol., 2017, 70, 37–83 CrossRef PubMed.
|
This journal is © The Royal Society of Chemistry 2024 |
Click here to see how this site uses Cookies. View our privacy policy here.