DOI:
10.1039/D4RA06457G
(Paper)
RSC Adv., 2024,
14, 34918-34924
Novel polycyclic “turn-on” and “turn-off” pyrazoline and pyrazole fluorescent sensors for selective real-world monitoring of Fe3+/Fe2+ in aqueous environments†
Received
6th September 2024
, Accepted 20th October 2024
First published on 31st October 2024
Abstract
Seven novel polycyclic pyrazoline and pyrazole sensors were synthesised and screened for useful photophysical properties with pyrazoline 2 and pyrazole 7, displaying an Fe3+ “turn-off” response in aqueous environments with Fe3+ limits of detection (LoD) of 2.12 μM and 3.41 μM, respectively. Both 2 and 7 sensors functioned in aqueous environments with real-world examples of Fe3+ detection in tap water and mineral water samples. 2 and 7 are suitable for the detection of Fe3+ at concentrations below the maximum iron limits for drinking water set by the Environmental Protection Agency (EPA) and European Union (EU).
Introduction
Iron is the most abundant transition metal in the human body1 and is vital for a range of biological functions, including oxygen transport via haemoglobin,2 catalytic activity of iron oxygenases,3 and DNA synthesis and repair.4 Ferric (Fe3+) iron and ferrous (Fe2+) iron are the two predominant forms of iron in the human body with the redox cycling between oxidation states being pivotal to their biological functions.1,5 Excess iron is linked to numerous medical problems,6 including hemochromatosis,7 Alzheimer's disease, and Parkinson's disease.8,9 Therefore, regular monitoring of iron intake is of paramount importance. The Environmental Protection Agency (EPA) in the USA has set the iron limit in drinking water at 5.4 μM,10 whereas the European Union (EU) has set it at 3.5 μM.11 Fluorescence spectroscopy offers many advantages in monitoring iron levels in drinking water, including a low limit of detection, high specificity and the ability to fine-tune the fluorescence emission wavelength (λem).12,13 Pyrazoline,14 a five-membered heterocycle with two adjacent nitrogen atoms (blue in Scheme 1 and Fig. 1), has well-established fluorescent properties with sensors reported for Zn2+,18 Al3+,19 and Fe3+.20 Pyrazole21 (red in Scheme 1 and Fig. 1) is closely related to pyrazolines and displays useful fluorescent properties.16,17 Chalcones22,23 are versatile precursors enabling the generation of large libraries of pyrazolines and pyrazoles via short (2–3 step) syntheses (Scheme 1) from commercially available starting materials.
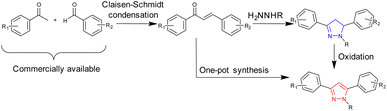 |
| Scheme 1 Synthesis of pyrazoline and pyrazoles from chalcone precursors. | |
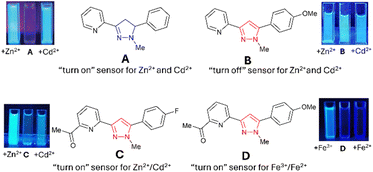 |
| Fig. 1 Recently reported pyrazoline and pyrazole fluorescent sensors; images are reproduced from ref. 15–17. | |
“Turn-on” sensors display an increased λem in the presence of an analyte, for example, A with Zn2+ and Cd2+ (Fig. 1).15 “Turn-off” sensors display reductions in the λem with an analyte; for example, B demonstrates a minor reduction in the λem with Zn2+ and Cd2+. Recent studies highlighted that the addition of an acetyl side group adjacent to the chelation site greatly enhanced analyte selectivity, for example, Zn2+/Cd2+ for C and Fe3+/Fe2+ for D.16,17 A variety of mechanistic pathways can account for an increased λem, including blocking of photoinduced electron transfer (PET)24 and the chelation enhanced fluorescence effect (CHEF).25 A decreased λem can result from the chelation enhancement quenching effect (CHEQ)26 or fluorescence resonance energy transfer (FRET).27
While sensors A–D confirm that structural complexity is not a prerequisite for complex functionality, their potential as fluorescent sensors to date has been limited to organic solvents.15–17 A key requirement for real-world monitoring is a fluorescent response in aqueous solutions. To address this research need and further investigate how to fine-tune the photophysical properties of these sensors, we developed seven novel sensors incorporating phenyl, naphthalene and anthracene units. Incorporation of one or more polycyclic component into sensor design has been shown to confer favourable fluorescent properties.18a,28 The two lead sensors (2 and 7) were analysed in aqueous solution, confirming that pyrazoline A and pyrazole B can transition from purely organic solvent sensors to sensors that are functional in mixed organic and aqueous solutions with minor modifications. The lead sensors displayed excellent selectivity for Fe3+/Fe2+, with the Fe3+ limit of detections below the USA and EU iron drinking water limit, validating their application in real-world monitoring of iron concentrations in drinking water.
Results and discussion
Chalcone precursors C1 with naphthalene (X = Nap) and C2 with anthracene (X = An) units were prepared (ESI S1†) via literature methods29,30 in excellent yield (75–93%) (Scheme 2). The pyrazoline series 1–4 was synthesised by adapting previously used methods15,17 in acceptable yields. The pyrazole series 5–7 were synthesised using a direct chalcone-to-pyrazole one-pot method.16 With seven novel sensors in hand, we investigated their photophysical properties initially in MeCN. MeCN was selected to allow direct comparison with three previous studies on the structurally related sensors A–D, which was also performed in MeCN. The lead sensors were then investigated in a mixed organic aqueous solution of 7
:
3 MeCN
:
H2O. Well established protocols for screening fluorescent sensors in organic solvents were followed throughout the study.28
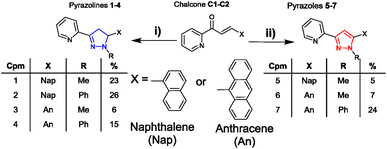 |
| Scheme 2 Synthesis of seven novel sensors from shared chalcone precursors: (i) 2.0 equivalent hydrazine (H2NNHMe or H2NNHPhe), MeOH, 60 °C, and 24 h (ref. 15) (ii) is the same as (i) + 1.0 equivalent CuCl2.16 | |
UV/vis spectroscopy was used to confirm that 1–7 undergo chelation. We initially used the group 12 metals (Zn2+, Cd2+ and Hg2+), as structurally similar sensors A and B are known to chelate this metal group (Fig. 2 for 1 + Zn2+, and ESI S4† for 2–7). Upon increasing equivalents of Zn2+, the initial absorbance band at 315 nm decreased with the formation of a new absorbance band at 350 nm up to 5.0 equivalents of Zn2+ (Fig. 2). Further additions resulted in a plateau at 350 nm. A similar trend was observed with the pyrazole series (see ESI S4† for 2–7).
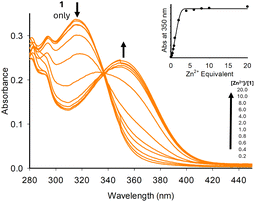 |
| Fig. 2 UV/vis study for 1 (20 μM, MeCN) with 0–20 equivalents of Zn2+. | |
1H NMR studies with and without Zn2+ were performed to confirm that 1–4 underwent chelation, with the results from 1 representative of all pyrazolines (Fig. 3 for 1, ESI S3† for 2–4). Upon addition of 2.0 equivalents of Zn2+, the pyridine protons demonstrated broadening and downfield movement in the chemical shift; for example, Ha from 8.57 ppm to 8.63 ppm, Hd from 7.97 ppm to 8.02 ppm, and Hc from 7.70 ppm to 7.90 ppm (Fig. 3A and B). This is characteristic of chelation, and has been reported previously for A (ref. 15) and other sensors.20,28a,e,31a,d,e
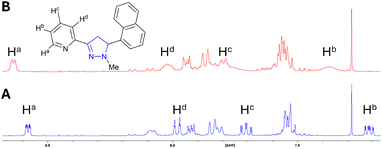 |
| Fig. 3 Partial 1H NMR spectra: (A) is 1 (20 μM, CDCl3) and (B) is 1 + 2.0 equivalents of Zn2+. | |
A further 1H NMR study was conducted for pyrazole 7 with a similar trend upon the addition of 2.0 equivalents of Zn2+ (Fig. 4). The chemical shift of pyridine protons Ha increased from 8.73 ppm to 8.77 ppm, while that of the anthracene singlet He increased from 8.55 ppm to 8.68 ppm and pyridine Hb from 7.31 ppm to 7.45 ppm. Broadening of all signals was also observed upon the addition of Zn2+, confirming chelation and agrees with a similar study conducted on C.17 These results indicate that the chelate site is centred about the pyridine ring, and chelation occurs with either a pyrazoline or pyrazole heterocycle, or a naphthalene or anthracene side unit.
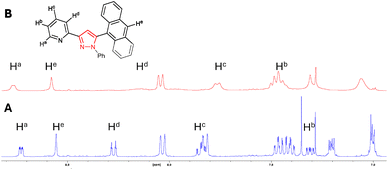 |
| Fig. 4 Partial 1H NMR spectra: (A) is 7 (15 μM, CDCl3) and (B) is 7 + 2.0 equivalents Zn2+. | |
The fluorescence response of pyrazoline 1 with a range of metals was investigated in MeCN with increased λem 460 nm with Cd2+ and Zn2+ (Fig. 5A), analogous to that of pyrazoline A, which was previously reported. A linear response of up to 5.0 equivalents of both Cd2+ and Zn2+, reaching a plateau on further addition, was observed (Fig. 5B and C). An unexpected “turn-on” response for Fe3+ at λem 535 nm suggested that 1 could function as a multi-analyte sensor, as observed in previous studies.32 This response was not observed in sensor A, suggesting that the naphthalene unit was responsible for this additional feature. The increase at λem 535 nm upon addition of Fe3+ peaked at 5.0 equivalents of Fe3+, with further addition reducing the fluorescence, which may be possibly due to paramagnetic quenching of the excited state (Fig. 5D).
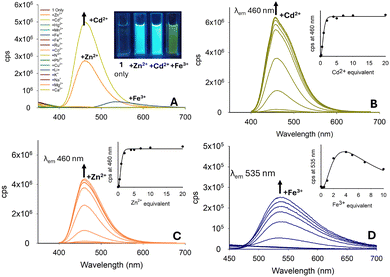 |
| Fig. 5 Photophysical properties of 1 (20 μM, MeCN). (Panel A) Metal screen at λex 280 nm with 5.0 equivalents of the indicated metal. (Panels B–D) Titration studies for Cd2+, Zn2+ and Fe3+, respectively; cps is counts per second. Cuvette images were taken under the irradiation of a 100 W λex 365 nm lamp. | |
Repeating the Cd2+ titration study for pyrazoline 1 in a 7
:
3 MeCN
:
H2O solution resulted in an approx. 90% reduction in the “turn-on” response (see ESI S5†), greatly hindering the potential of 1 in aqueous environments. Previous sensors A–D and pyrazoline 1 all had a methyl substituent on the nitrogen ring. In pyrazoline 2, this was replaced by a phenyl unit and unexpectedly reversed the fluorescence response from “turn-on” to “turn-off”, which was most noticeable for Fe3+ (Fig. 6A). The λem at 470 nm for 2 only (φf 0.74) decreased with up to 5.0 equivalents Fe3+ (φf < 0.01), and further addition resulted in complete quenching of the fluorescence (Fig. 6B). A Fe2+ titration study demonstrated no significant change in λem 470 nm with up to 20.0 equivalents (Fig. 5C), suggesting that pyrazoline 2 could selectively detect iron in the +3 oxidation state over iron in the +2 oxidation state (Fe3+/Fe2+). A Fe3+ titration study in 7
:
3 MeCN
:
H2O was conducted to determine if this “turn-off” response remained in aqueous samples (see Fig. 6D for Fe3+, and ESI S5† for the full metal screen). The presence of water reduced λem by approximately 30% (φf 0.83), but retained a linear (R2 = 0.984) reduction in λem (φf 0.07) in the concentration range between 20–100 μM Fe3+, suggesting that pyrazoline 2 could be utilised for the selective quantification of Fe3+ in aqueous samples (Fig. 6D). A slight increase of 20 nm in λem from 470 nm in 100% MeCN to 490 nm in 7
:
3 MeCN
:
H2O was observed (Fig. 5D).
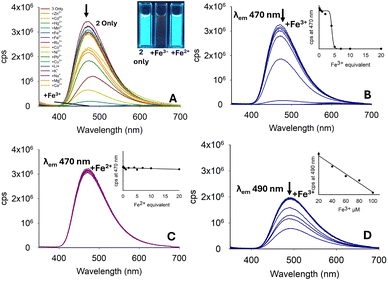 |
| Fig. 6 Photophysical properties of 2 (20 μM, MeCN). (Panel A) is the metal screen at λex 280 nm with 5.0 equivalents of the indicated metal. (Panels B and C) are titration studies for Fe3+ and Fe2+ in MeCN, respectively. (Panel D) is the Fe3+ titration in 7 : 3 MeCN : H2O; cps is counts per second. Cuvette images were taken under the irradiation of a 100 W λex 365 nm lamp. | |
Our focus shifted to the anthracene pyrazolines 3–4 to determine if a third aromatic ring would confer beneficial properties. Pyrazoline 3 displayed a “turn-off” response for Fe3+/Fe2+ at 420 nm. However, several other metals produced a “turn-off” response that was equal to or greater than that of Fe3+; for example, Co2+ and Cu2+ (Fig. 7A). The λem of 3 only was significantly less than that of 2, further limiting the application of this pyrazoline as a sensor.
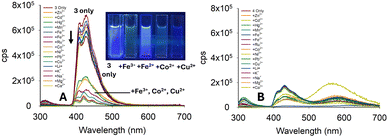 |
| Fig. 7 Photophysical properties of 3 (20 μM, MeCN) (Panel A) and 4 (Panel B) metal screen at λex 250 nm with 5.0 equivalents of the indicated metal; cps is counts per second. Cuvette images were taken under the irradiation of a 100 W λex 365 nm lamp. | |
Pyrazoline 4 contained a phenyl instead of a methyl group. This change significantly altered the photophysical properties of 1 and 2. Pyrazoline 4 was also analysed, and displayed very weak fluorescence at 420 nm with insignificant differences observed on addition of a range of metals (Fig. 7B). The λem of 4 was approximately a third of the value for 3, suggesting that 4 was also unsuitable for sensing. In summary, the two anthracene pyrazolines did not display improved fluorescence properties over the naphthalene sensors. A summary of the pyrazoline series is displayed in Fig. 8.
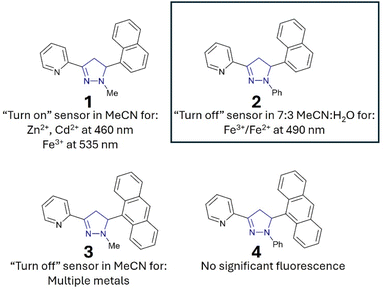 |
| Fig. 8 Summary of the pyrazoline series. 2 (inset) was selected for further investigation. | |
With the initial studies on the pyrazoline series complete and pyrazoline 2 selected for further investigation, we performed a similar analysis on the pyrazole series 5–7. To our surprise, pyrazole 5 displayed a “turn-on” response at 310 nm (Fig. 9A), whereas pyrazole 6 with an additional aryl ring displayed a “turn-off” response to a variety of metals (Fig. 7B). Unfortunately, no selectively to Fe3+ was observed. Therefore, these pyrazoles were not selected for further investigation.
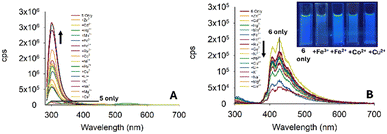 |
| Fig. 9 (Panel A) Metal screen for 5 (20 μM, MeCN) at λex 280 nm with 5.0 equivalents of the indicated metal. (Panel B) Metal screen for 6 at λex 250 nm in MeCN; cps is counts per second. Cuvette images were taken under the irradiation of a 100 W λex 365 nm lamp. | |
Pyrazole 7 with a phenyl unit on the pyrazole nitrogen displayed significant λem intensity at 420 nm in the absence of metals (φf 0.33), and this was diminished only in the presence of a small number of metals, most noticeably Fe3+, Co2+ and Cu2+ (Fig. 10A). Significant reduction in λem at 420 nm was observed upon the addition of 4.0 equivalents of Fe3+ (φf < 0.01). Further addition of Fe3+ resulted in complete quenching of the fluorescence intensity (Fig. 10B). A similar study with Fe2+ demonstrated no reduction, showing excellent Fe3+/Fe2+ selectivity (Fig. 10C). Repeating the Fe3+ titration in a 7
:
3 MeCN
:
H2O solution demonstrated an excellent “turn-off” response with a linear reduction in λem (R2 = 0.978) (φf 0.43 to φf 0.18), suggesting this would be a suitable sensor for Fe3+ in aqueous environments (see Fig. 10D for Fe3+, and ESI† for the full metal screen). A summary of the pyrazole series is displayed in Fig. 11 and pyrazole 7 was selected for further investigation.
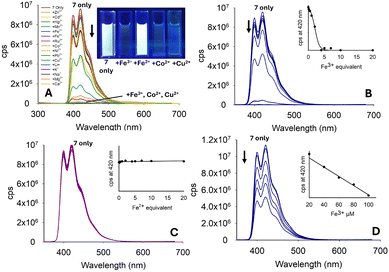 |
| Fig. 10 Photophysical properties of 7 (20 μM, MeCN). (Panel A) Metal screen at λex 280 nm with 5.0 equivalents of the indicated metal. (Panels B and C) Titration studies for Fe3+ and Fe2+ in MeCN, respectively. (Panel D) Fe3+ titration in 7 : 3 MeCN : H2O; cps is counts per second. Cuvette images were taken under the irradiation of a 100 W λex 365 nm lamp. | |
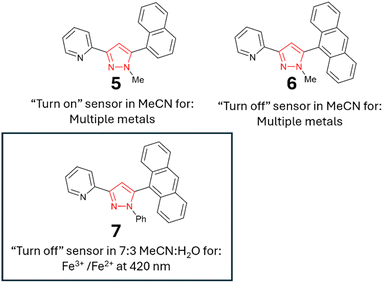 |
| Fig. 11 Summary of the pyrazole series; 7 (inset) was selected for further investigation. | |
With two lead sensors selected, one pyrazoline and one pyrazole, we performed a range of competition assays to determine if the Fe3+-triggered fluorescence “turn-off” response is retained in the presence of competing cations. Pyrazoline 2 was the most affected by competition across the range of cations screened, except for Ru3+ (Fig. 12A). Pyrazole 7 was similarly impacted by the presence of competing cations, except for Ru3+ and Cu2+ (Fig. 12B). This result suggests that neither naphthalene or anthracene, nor pyrazoline or pyrazole, are responsible for the high competition observed. It is highly possible that the large open chelation site, as demonstrated from a previous X-ray crystal structure for the pyrazole analogue of A,15 is responsible. Restricting the chelation site (for example, by the addition of an acetyl group) should be further explored in future analogues of 2 and 7, as this was shown to be highly effective for C and D.17
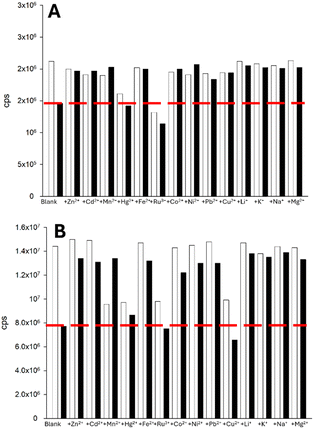 |
| Fig. 12 Competition assays for 2 (Panel A) and 7 (Panel B). The white bar represents the sensor (20 μM, MeCN) with 5.0 equivalents of the indicated cation; the black bars is the same with 5.0 equivalents Fe3+ after equilibrating for 3 min. 2 λex 280 nm with λem 490 nm; 7 λex 250 nm with λem 420 nm. | |
A study was conducted to determine the real-world potential for 2 and 7 at detecting Fe3+ in two types of samples: tap water and mineral water. Both 2 and 7 demonstrated measurable reductions in λem at 420 nm in the presence of 50 μM and 100 μM Fe3+ (Fig. 13 for 7 and ESI S6† for 2).
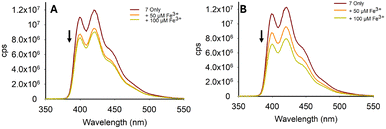 |
| Fig. 13 Fe3+-triggered “turn-off” response for 7 in tap water (Panel A). Fe3+-triggered “turn-off” response for 7 in mineral water (Panel B), λex 250 nm. Solvent was 7 : 3 MeCN : H2O; cps is counts per second. | |
This study indicated that despite the reduction in the “turn-off” response observed in the competition assays (Fig. 12), the “turn-off” response was detectable in real world samples. Limit of detection (LoD) studies were performed in a 7
:
3 MeCN
:
H2O solution, and confirmed that 2 and 7 had LoD values of 2.12 μM and 3.41 μM, respectively (see ESI S8†). This confirmed that both pyrazoline 2 and pyrazole 7 can detect Fe3+ below the iron drinking water limit of the EPA and EU, and validates their application in real-world monitoring. These LoD are comparable with other Fe3+-specific fluorescence sensors. For example, Chattopadhyay et al. reported on a Fe3+-specific “turn-off” sensor with a LoD value of 3.5 μM,33 Goswami et al. reported on a Fe3+ “turn-on” sensor with a LoD of 2.9 μM,34 and Wang et al. reported on a Fe3+-specific colorimetric sensor with a LoD value of 1.0 μM35 (see ESI S8† for further Fe3+ LoD examples). An additional study was conducted with 7 using pond (Fig. 14A) and river (Fig. 14B) water samples with a measurable “turn-off” response with 50 μM and 100 μM Fe3+, respectively (see ESI S7† for similar study with 2). This confirmed that both 2 and 7 can operate in external water sources containing a range of interferences beyond the cations screened in the competition studies (Fig. 12), such as sediment and bacteria present in natural water sources.
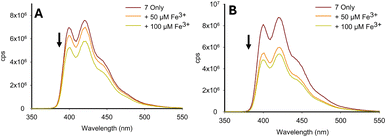 |
| Fig. 14 Fe3+-triggered “turn-off” response for 7 in pond water (Panel A). Fe3+-triggered “turn-off” response for 7 in river water (Panel B), λex 250 nm. Solvent composition was 7 : 3 MeCN : H2O; cps is counts per second. | |
A reversibility study was conducted with 2 and 7 in the presence of several cycles of Fe3+, followed by EDTA, confirming that both sensors can be used multiple times for Fe3+ detection (Fig. 15A for 2 and Fig. 15B for 7).
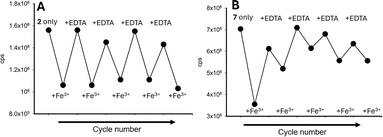 |
| Fig. 15 Repeatability study of the addition of 5.0 equivalents of Fe3+, followed by 5.0 equivalents of EDTA, repeated for 5 cycles in a 7 : 3 MeCN : H2O solution. (Panel A) is 2 λex 280 nm and (Panel B) is 7 λex 250 nm; cps is counts per second. | |
A proposed 1
:
1 binding mechanism of Fe3+ with sensors 2 and 7 is shown in Fig. 16, and agrees with the Job plot for 7 with Fe3+ (see ESI S11†) and the previously reported X-ray crystal structure complex of the pyrazole A analogue with Zn2+.15
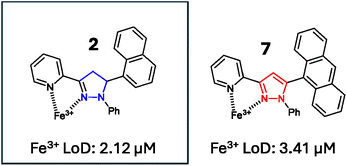 |
| Fig. 16 Proposed 1 : 1 binding mechanism of 2 and 7 with Fe3+ with the calculated limit of detection for Fe3+ in 7 : 3 MeCN : H2O solution. Pyrazoline 2 was selected as the lead compound from this study. | |
Conclusions
Seven novel polycyclic pyrazoline and pyrazoles were synthesised to determine how phenyl, naphthalene and anthracene units influence photophysical properties. Small modifications (for example, substitution of a methyl in 1 for a phenyl in 2) reversed the photophysical properties from “turn-on” to “turn-off”. 2 retained a good “turn-off” response in 7
:
3 MeCN
:
H2O solutions, and was selected for further analysis. The addition of a third aromatic ring to 2, resulting in 3, significantly disrupted λem and was detrimental to sensing. Pyrazoline 4 displayed minimum λem and was unsuitable for sensing applications. For the pyrazole series, 5 demonstrated a “turn-on” response at λem 310 nm to a variety of metals, whereas 6 displayed a “turn-off” response at λem 420 nm to a range of metals. Neither showed selectively to Fe3+, and were not suitable for sensing. 7 was the most useful pyrazole with complete quenching of λem at 420 nm on addition of 4.0 equivalents of Fe3+ in organic solutions. Lead sensors 2 and 7 were confirmed to display a Fe3+-triggered “turn-off” response in aqueous samples with the Fe3+ limit of detection values of 2.12 μM and 3.41 μM, respectively. Real-world analysis confirmed that 2 and 7 could detect Fe3+ in both tap water and mineral waters, and be useful for the industrial scale monitoring of Fe3+ in drinking water. 2 and 7 were also confirmed to display a “turn-off” response in pond and river waters, suggesting these sensors could be used for environmental monitoring of Fe3+ in external water sources. Further work is required to mitigate competing cations from influencing the fluorescence response. The introduction of additional chelation groups around the pyridine is a promising solution, as it has been shown to be highly beneficial for C and D.17 These results set a firm foundation for the development of future generations of improved phenyl substituted pyrazoline and pyrazole Fe3+-specific “turn-off” sensors that are purposely designed to operate in aqueous environments.
Data availability
The data supporting this article have been included as part of the ESI.†
Author contributions
Alexander Ciupa designed, synthesized, characterised, performed all spectroscopy studies, and authored the manuscript.
Conflicts of interest
There are no conflicts to declare.
Acknowledgements
The author acknowledges Steven Robinson for assistance with time-of-flight high resolution mass spectrometry and Krzysztof Pawlak with fluorescence spectroscopy. This work used shared equipment located at the Materials Innovation Factory, created as part of the UK Research Partnership Innovation Fund (Research England) and co-funded by the Sir Henry Royce Institute.
References
- Selected examples:
(a) L. Silvestri, M. Pettinato, V. Furiosi, L. Bavuso Volpe, A. Nai and A. Pagani, J. Mol. Sci., 2023, 24, 3995 CrossRef CAS PubMed;
(b) N. Abbaspour, R. Hurrell and R. Kelishadi, Res. J. Med. Med. Sci., 2014, 19, 164 Search PubMed;
(c) A. Yiannikourides and G. O. Latunde-Dada, Medicines, 2019, 6, 85 CrossRef CAS PubMed.
- A.-C. S. Vogt, T. Arsiwala, M. Mohsen, M. Vogel, V. Manolova and M. F. Bachmann, Int. J. Mol. Sci., 2021, 22, 4591 CrossRef CAS PubMed.
- P. C. A. Bruijnicx, G. Van Koten and R. J. M. Klein Gebbink, Chem. Soc. Rev., 2008, 37, 2716 RSC.
- S. Puig, L. Ramos-Alonso, A. M. Romero and M. T. Martínez-Pastor, Metallomics, 2017, 9, 1483 CrossRef PubMed.
- P. A. Frey and G. H. Reed, ACS Chem. Biol., 2012, 7, 1477 CrossRef CAS PubMed.
- G. J. Brewer, Chem. Res. Toxicol., 2010, 23, 319 Search PubMed.
- A. Piperno, S. Pelucchi and R. Mariani, Transl. Gastroenterol. Hepatol., 2020, 5, 25 Search PubMed.
- J.-L. Liu, Z.-Y. Wang and C. Guo, Front. Neurosci., 2018, 12, 411985 Search PubMed.
- K. Wojtunik-Kulesza, A. Oniszczuk and M. Waksmundzka-Hajnos, Biomed. Pharmacother., 2019, 111, 1277 CrossRef CAS PubMed.
- Environmental Protection Agency, Secondary drinking water regulations: Guidance for nuisance chemicals, 2013. https://www.epa.gov/sdwa/secondary-drinking-water-standards-guidance-nuisance-chemicals Search PubMed.
- Directive (EU) 2020/2184 Of The European Parliament And Of The Council of 16 December 2020, on the quality of water intended for human consumption, 2015, http://data.europa.eu/eli/dir/2020/2184/oj Search PubMed.
- H. N. Kim, W. X. Ren, J. S. Kim and J. Yoon, Chem. Soc. Rev., 2012, 41, 1130 RSC.
- Y. Chen, Y. Bai, Z. Han, W. He and Z. Guo, Chem. Soc. Rev., 2015, 44, 4517 RSC.
- B. Varghese, S. N. Al-Busa, F. O. Suliman and S. M. Z. Al-Kindy, RSC Adv., 2017, 7, 46999 RSC.
- A. Ciupa, M. F. Mahon, P. A. De Bank and L. Caggiano, Org. Biomol. Chem., 2012, 10, 8753 RSC.
- A. Ciupa, New J. Chem., 2024, 48, 13900 RSC.
- A. Ciupa, RSC Adv., 2024, 14, 3519 RSC.
- Selected examples
(a) T. T. Zhang, X. P. Chen, J. T. Liu, L. Z. Zhang, J. M. Chu, L. Su and B. X. Zhao, RSC Adv., 2014, 4, 16973 RSC;
(b) Z. L. Gong, F. Ge and B.-X. Zhao, Sens. Actuators, B, 2011, 159, 148 CrossRef CAS;
(c) M. M. Li, F. Wu, X. Y. Wang, T. T. Zhang, Y. Wu, Y. Xiao, J. Y. Miao and B.-X. Zhao, Anal. Chim. Acta, 2014, 826, 77 CrossRef CAS PubMed;
(d) T.-T. Zhang, F.-W. Wang, M.-M. Li, J. T. Liu, J. Y. Miao and B.-X. Zhao, Sens. Actuators, B, 2013, 186, 755 CrossRef CAS.
- Selected examples: R. Manjunath and K. Palaninathan, New J. Chem., 2018, 42, 10891 RSC.
- Selected examples
(a) P. Sharma, S. Bhogal, I. Mohiuddin, M. Yusuf and A. K. Malik, J. Fluoresc., 2022, 32, 2319 Search PubMed;
(b) Y.-P. Zhang, Q. Teng, Y.-S. Yang, H.-C. Guo and J.-J. Xue, Inorg. Chim. Acta, 2021, 525, 120469 CrossRef CAS;
(c) A. M. Asiri, N. S. M. Al-Ghamdi, H. Dzudzevic-Cancar, P. Kumar and S. A. Khan, J. Mol. Struct., 2019, 1195, 670 CrossRef CAS;
(d) Y. P. Zhang, X. F. Li, Y. S. Yang, J. L. Wang, Y. C. Zhao and J. J. Xue, J. Fluoresc., 2021, 31, 29 CrossRef CAS PubMed;
(e) S. Maity, A. Kundu and A. Pramanik, RSC Adv., 2015, 5, 52852 RSC.
- Selected examples
(a) A. Tigreros and J. Portilla, RSC Adv., 2020, 10, 19693 RSC;
(b) M.-C. Ríos and J. Portilla, Chemistry, 2022, 4, 940 CrossRef;
(c) J.-C. Castillo and J. Portilla, Targets Heterocycl. Syst., 2018, 22, 194 CAS;
(d) S. Fustero, M. Sánchez-Roselló, P. Barrio and A. Simón-Fuentes, Chem. Rev., 2011, 111(11), 6984 CrossRef CAS PubMed.
- A. A. Singh and V. Kumar, Eur. J. Med. Chem., 2014, 85, 758 CrossRef PubMed.
- A. Ciupa, N. J. Griffiths, S. K. Light, P. J. Wood and L. Caggiano, Med. Chem. Commun., 2011, 2, 1011 RSC.
- H. Nui, J. Liu, H. M. O'Connor, T. Gunnlaugsson, T. D. James and H. Zhang, Chem. Soc. Rev., 2023, 52, 2322 RSC.
- J. W. Nugent, H. Lee, H.-S. Lee, J. H. Reibenspies and R. D. Hancock, Chem. Commun., 2013, 49, 9749 RSC.
- L. G. T. A. Duarte, F. L. Coelho, J. C. Germino, G. G. da Costa, J. F. Berbigier, F. S. Rodembusch and T. D. Z. Atvars, Dyes Pigm., 2020, 181, 108566 CrossRef.
- L. Wu, C. Huang, B. P. Emery, A. C. Sedgwick, S. D. Bull, X. P. He, H. Tian, J. Yoon, J. L. Sessler and T. D. James, Chem. Soc. Rev., 2020, 49, 5110 RSC.
- Selected examples
(a) A. Sahana, A. Banerjee, S. Das, S. Lohar, D. Karak, B. Sarkar, S. K. Mukhopadhyay, A. K. Mukherjee and D. Das, Org. Biomol. Chem., 2011, 9, 5523 RSC;
(b) S. Santhoshkumar, K. Velmurugan, J. Prabhu, G. Radhakrishnan and R. Nandhakumar, Inorg. Chim. Acta, 2016, 439, 1 CrossRef CAS;
(c) N. Bhuvanesh, S. Suresh, K. Kannan, V. R. Kannan, N. Maroli, P. Kolandaivel and R. Nandhakumar, New J. Chem., 2019, 43, 2519 RSC;
(d) Y. Mise, K. Imato, T. Ogi, N. Tsunoji and Y. Ooyama, New J. Chem., 2021, 45, 4161 RSC;
(e) D. Karak, S. Das, S. Lohar, A. Banerjee, A. Sahana, I. Hauli, S. K. Mukhopadhyay, D. A. Safin, M. G. Babashkina, M. Bolte, Y. Garcia and D. Das, Dalton Trans., 2013, 42, 6708 RSC;
(f) G. Mun, S. H. Jung, A. Ahn, S. S. Lee, M. Y. Choi, D. H. Kim, J.-Y. Kim and J. H. Jung, RSC Adv., 2016, 6, 53912 RSC.
- Z. Abbas, S. Dasari and A. K. Patra, RSC Adv., 2017, 7, 44272 RSC.
- H. G. El-Attar, M. A. Salem, S. A. Ibrahim and E. A. Bakr, Res. Chem. Intermed., 2023, 49, 469 CrossRef CAS.
- Selected examples
(a) X. Wu, Z. Zhang, H. Liu and S. Pu, RSC Adv., 2020, 10, 15547 RSC;
(b) S. Lohar, S. Pal, M. Mukherjee, A. Maji, N. Demitri and P. Chattopadhyay, RSC Adv., 2017, 7, 25528 RSC;
(c) M. Akula, P. Z. El-Khoury, A. Nag and A. Bhattacharya, RSC Adv., 2014, 4, 25605 RSC;
(d) Y. Zhang, H. Lui, W. Gao and S. Pu, RSC Adv., 2019, 9, 27476 RSC;
(e) W. Luo, Z. Yuwen, H. Li and S. Pu, New J. Chem., 2022, 46, 2411 RSC.
- Selected examples
(a) D. Li, A. Liu, Y. Xing, Z. Li, Y. Luo, S. Zhao and J. Li, Dyes Pigm., 2023, 213, 111180 CrossRef CAS;
(b) P. Kaur and D. Sareen, Dyes Pigm., 2011, 88, 296 CrossRef CAS.
- S. Sen, S. Sarkar, B. Chattopadhyay, A. Moirangthem, A. Basu, K. Dhara and P. Chattopadhyay, Analyst, 2012, 137, 3335 RSC.
- S. Paul, A. Manna and S. Goswami, Dalton Trans., 2015, 44, 11805 RSC.
- X. Lui, N. Li, M.-M. Xu, J. Wang, C. Jiang, G. Song and Y. Wang, RSC Adv., 2018, 8, 34860 RSC.
|
This journal is © The Royal Society of Chemistry 2024 |
Click here to see how this site uses Cookies. View our privacy policy here.