DOI:
10.1039/D4RA06804A
(Paper)
RSC Adv., 2024,
14, 40006-40017
Optimization of physicochemical properties of theophylline by forming cocrystals with amino acids†
Received
21st September 2024
, Accepted 15th December 2024
First published on 20th December 2024
Abstract
This study presents the synthesis and characterization of novel cocrystal structures of theophylline (THE) with the amino acids gamma-aminobutyric acid (GABA) and L-arginine (ARG). Despite a large number of reports about THE cocrystals, no crystallographic parameters of cocrystals formed by THE and amino acids have been reported. THE is characterized by low solubility, while amino acids as cocrystal co-formers (CCFs) are increasingly recognized for their high solubility and safety. Consequently, the synthesis of cocrystals with amino acids has garnered considerable research interest. To optimize THE's physicochemical properties, amino acids were chosen as CCFs, resulting in the synthesis of two novel cocrystals: THE-GABA and THE-ARG-2H2O. Comprehensive characterization, such as X-ray diffraction analysis, spectral analysis, thermal analysis, and dynamic vapor sorption were conducted for THE-GABA and THE-ARG-2H2O, alongside stability and solubility assessments. To better explain the characterization and evaluation results, the theoretical calculation methods were adopted, including the molecular electrostatic potential (MESP), topological analysis, energy framework, Hirshfeld surface and crystal voids. The study's findings reveal that the solubility and permeability of THE in both novel cocrystals, THE-GABA and THE-ARG-2H2O, have increased, especially in the latter. Meanwhile, the hygroscopicity of them was at a low level which was basically consistent with THE.
Introduction
Theophylline (THE), a medication commonly used for respiratory diseases1–3 like chronic obstructive pulmonary disease (COPD) and asthma,1,2,4–7 functions by inhibiting phosphodiesterase (PDE) and thus preventing the breakdown of cAMP within cells. At least four anhydrous forms (form I, form II, form III, form IV) and a monohydrate of THE have been reported, among which form II is used for experiments. As a marketed form, form II is more stable than form I at room temperature.8 Form III is produced by dehydration of monohydrate under low pressure or vacuum conditions.9 Form IV is the most thermodynamically stable anhydrous polymorph.10
However, THE is associated with side effects like those of caffeine, primarily affecting the gastrointestinal system, such as nausea, as well as the psychiatric and nervous systems, including symptoms like anxiety and insomnia.11,12 Furthermore, THE's low water solubility restricts its pharmaceutical development and efficacy. Enhancing solubility can reduce the amount of active pharmaceutical ingredient (API) required for equivalent therapeutic effects.13,14 Therefore, increasing the water solubility of THE has been a significant focus of research.
Cocrystals offer a promising method for enhancing the physical and chemical attributes of API.15–17 Cocrystals are unique single crystals formed in a specific stoichiometric ratio, consisting of two or more distinct solid molecular compounds bonded through supramolecular forces.17,18 In the context of APIs, incorporating cocrystal co-formers (CCFs) significantly alters the charge density of the molecule.19,20 This development of cocrystals is often associated with modifications in their physicochemical properties.21–24 Studies have demonstrated that cocrystals can alter various characteristics such as melting point, dissolution rate, thermal stability, and permeability, thereby impacting the drug's bioavailability without altering its chemical structure.25
Based on the Cambridge Crystallographic Data Centre (CCDC) and literature research, approximately 130 cocrystals of THE have been reported. One part of CCFs is acidic with an acidic group such as tartaric acid,26 ferulic acid,27 and aspirin.28–30 The other part is nitrogen-containing alkaline, such as benzamide,31 lamotrigine,32 and niclosamide.33–35 However, no crystals of THE with amino acids are reported on CCDC.
Amino acids, due to their low toxicity and natural occurrence, are deemed ideal and promising CCF candidates.36 As zwitterions, amino acids can exhibit both acidity and alkalinity.37 At the same time, amino acids have been widely used as CCFs to improve the stability and solubility of an API38,39 in cocrystal structures. The solubility of ritonavir increased after forming a cocrystal with D-alanine.40 In addition, cocrystals with amino acids can also reduce the side effects of the drug substance.41 Mesalamine was an anti-inflammatory drug in the small intestine with a bloating effect. Mesalamine hydrochloride's cocrystal with glutamine had an acid–base stabilization effect in the gastrointestinal fluid, which reduced bloating.42 There have been even cocrystals with amino acids already on the market, such as Steglatro (co-crystal between ertugliflozin and L-pyroglutamic acid) and Suglat (co-crystal between ipragliflozin and L-proline).43 The commercially available product related to amino acids is sodium theophylline glycinate salt,44 for which no crystallographic parameters have been reported. The sodium theophylline salt has been found to sustain high plasma concentrations of THE without posing a significant risk of severe adverse reactions.45 This potential advantage highlights the substantial research significance of cocrystals formed by THE and amino acids.
Two novel cocrystals formed from THE and amino acids were obtained: theophylline-gamma-aminobutyric acid (THE-GABA) and theophylline-arginine-2H2O (THE-ARG-2H2O). The structures of API and CCFs are shown in Scheme 1. As illustrated in Scheme 2, the MESP of these compounds are marked by red areas indicating electron-rich regions and blue areas denoting electron-deficient regions. During the formation of zwitterionic ions via intramolecular proton transfer, the electron density tends to concentrate, which manifests itself in MESP as greater local maxima and smaller local minima. In this context, the electron-rich region of THE (carbonyl) has the potential to establish hydrogen bonds with the electron-deficient region (quaternary ammonium salt) of GABA and ARG.
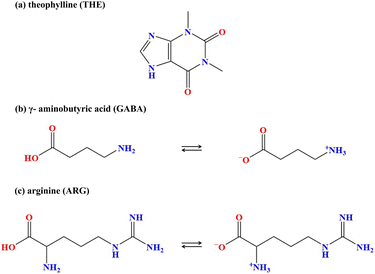 |
| Scheme 1 Chemical structures of THE, ARG, and GABA. | |
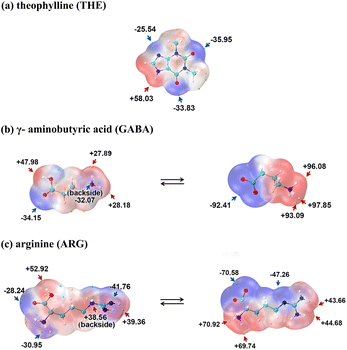 |
| Scheme 2 MESP (kcal mol−1) of THE, ARG, and GABA. | |
In addition to its ability to cross the blood–brain barrier as a major inhibitory neurotransmitter in the mammalian central nervous system (CNS), gamma-aminobutyric acid (GABA) plays a crucial role in promoting neuronal development and relaxation, as well as in the prevention of insomnia and depression.46,47 Arginine (ARG), classified as a nonessential amino acid in healthy adults,48 contributes to immune function by increasing growth hormone secretion.49 Therefore, the cocrystals of THE-GABA and THE-ARG-2H2O hold promising implications in both pharmaceutical and nutritional domains.
In this study, the novel de novo synthesized cocrystals were characterized by single-crystal X-ray diffraction (SCXRD), powder X-ray diffraction (PXRD), differential scanning calorimetry (DSC), dynamic vapor sorption (DVS), and infrared spectroscopy (IR). Additionally, their solubility was systemically assessed in conditions simulating the in vivo environment and in pure water. Subsequently, the results were analyzed and interpreted through theoretical calculations.
Experiment
Materials
THE (Form II) raw material was purchased from Wuhan Yuancheng Gongchuang Technology Co., Ltd (Hubei, China). Arginine was acquired from Hubei Wande Chemical Industry Co., Ltd (Hubei, China), and γ-aminobutyric acid was obtained from Beijing Chemical Reagent Co. (Beijing, China). All analytical grade solvents were sourced from Sigma-Aldrich (St. Louis, MO, USA).
Synthesis and crystallization
Cocrystals of THE-GABA and THE-ARG-2H2O were synthesized by solvent-assisted grinding with mortar and pestle. Equimolar amounts of THE (180.1 mg, 1 mmol) and GABA (103.1 mg, 1 mmol) with 4 mL ethanol were ground for about 40 min and equimolar amounts of THE (180.1 mg, 1 mmol) and ARG (174.2 mg, 1 mmol) in 2 mL water were ground for about 40 min. The two cocrystals were both dissolved in methanol and then left to stand under ambient conditions for approximately 5–10 days, resulting in the formation of colorless crystals.
Unsaturated states of THE: to attain unsaturation, 2 mL water solution was added, followed by approximately 10 mg of THE. The solution was stirred at 37 °C for 48 hours after the rotor was added, and the residual powder was measured after the water solution evaporated.
Supersaturated states of THE: to attain supersaturation, 2 mL water solution was added, followed by approximately 50 mg of THE. The solution was stirred at 37 °C for 48 hours after the rotor was added, and the residual powder was measured after the water solution evaporated.
SCXRD analysis
The Rigaku XtaLAB Synergy four-circle diffractometer (Rigaku, Americas) with Cu-Kα radiation (wλ = 1.54178 Å) was used to collect all the diffraction intensity data of single crystals. The SCXRD measurement was conducted at 293 K. Crystal structures were generated through Olex2 (Version 1.5)50–53 and SHELXL (2018.3)54 software. The crystal structure was solved by the direct method,55 using the least squares method to correct the structural parameters. All the non-hydrogen atoms were refined anisotropically and the hydrogen atoms were located using Fourier analysis and geometric calculation.
THE-GABA (1
:
1) crystallized in the Ia space group of the monoclinic system and the asymmetric unit of one contains one THE molecule and one GABA molecule. THE-ARG-2H2O (1
:
1
:
2) crystallized in the P1 space group of the triclinic system and the asymmetric unit of one contains one THE molecule, one ARG molecule and two water molecules.
PXRD analysis
PXRD patterns of API, CCFs, and cocrystals were collected with a Rigaku D/Max-2550 powder X-ray diffractometer (Rigaku, Tokyo, Japan) under a Cu Kα radiation of λ = 1.54178 Å, 2θ scanning range of 3–40°, and a scanning speed of 8° min−1. Simultaneously, the theoretical powder patterns of the cocrystals were calculated using the Mercury (Version 2023.3.0)56 software.
DSC analysis
DSC curves of API, CCFs, and cocrystals were measured by a DSC instrument (Mettler Toledo, Switzerland). Samples (3–5 mg) were placed in an aluminum crucible with a lid and heated at 10 °C min−1. STARe evaluation software (Version 16.30) was used to process the atlas.
IR analysis
Each sample was measured by Spectrum 400 Fourier transform IR with attenuated total reflection accessory (PerkinElmer, Waltham, MA, USA). The experimental conditions were over the range of 4000–400 cm−1 with 16 scanning times having a resolution of 4.000 cm−1.
DVS analysis
DVS is a well-established method for the measurements of vapor sorption and desorption.57,58 Surface Measurement Systems (SMS) DVS apparatus (London, U.K.) was used to record moisture sorption and desorption measurements of THE and cocrystals. Approximately 30–50 mg of the sample was placed onto a quartz DVS round-bottom pan. The sample was pre-equilibrated with a continuous flow of dry nitrogen for 5 min. Subsequently, the relative humidity (RH) was incrementally increased from 0% to 90%, with each step consisting of a 10% increase. Following this, the RH gradually decreased to 0% in equal increments. Throughout the process, the equilibrium criterion, defined as dm /dt (the rate of mass change over time), was maintained at 0.02% min−1 for every step.
Solubility measurements
The solubility tests of THE and cocrystals were performed at 37 °C in the dissolution media of water (pH 7.0), phosphate buffer (pH 6.8), acetate buffer (pH 4.5), and hydrochloric aqueous solution (pH 1.2). The buffer recipes were as follows: pH 7.0: purified water; pH 6.8
:
6.8 g of potassium dihydrogen phosphate and 0.94 g sodium hydroxide were dissolved in 1 L water; pH 4.5
:
18 g sodium acetate and 9.8 mL glacial acetic acid were added to 1 L water; pH 1.2
:
9 mL hydrochloric acid was added to 1 L water.
After the addition of 2 mL buffer solution, about 50 mg cocrystal samples were added to achieve a suspension state. Subsequently, the mixture was stirred for 48 h at 37 °C, diluted to the desired concentration, and analyzed using high-performance liquid chromatography (HPLC). The concentrations of THE were quantified on an Agilent HPLC system (Agilent 1200 series, East Brunswick, NJ, USA) with a Welch Materials XB-C18 column (4.6 mm × 250 mm, 5 μm), and the detection wavelength was 271 nm. The mobile phase was prepared with water: ACN (85
:
15); the flow rate was 1 mL min−1, and the column temperature was set at 30 °C. The remaining powder was collected and dried for phase analysis by PXRD.
IDR test
The intrinsic dissolution rate (IDR) of THE and its cocrystals was measured by an RC12AD dissolution apparatus over 30 min. Accurately weighed solids equivalent to 120 mg of THE were placed in the intrinsic attachment and compressed at a pressure of 300 kg for 1 min to create a flat surface on one side. The container holding the compressed sample was immersed in 900 mL of deionized water, maintained at a temperature of 37 ± 0.2 °C. Samples were collected at 2, 6, 10, 14, 18, 22, 26, 30 min at 37 °C and 100 rpm rotating speed at the same depth. After filtration through a 0.45 μm syringe tip filter, the samples were detected on an HPLC system while the test condition remained the same as mentioned in Solubility measurements.
Permeability test
Permeability of THE and cocrystals was determined experimentally by cellulose nitrate membrane (0.45 μm, Cytiva, Germany) and a modified Franz diffusion cell apparatus. 5 mL buffer medium (pH 6.8) was added to the device and bubbles were expelled. The buffer medium was then kept at 37 ± 0.2 °C and rotated at 100 ± 5 rpm. Then 10 mg THE and the same equivalent of cocrystals were placed on the membrane. According to the set predetermined time, a 0.5 mL sample was taken from the device at a time and supplemented with fresh medium.
Theoretical calculation
The intermolecular interactions between API and CCFs were explored by density functional theory, and topological analysis was conducted by applying the quantum theory of atoms in molecules (QTAIMs).59 The B3LYP-D3/6-31G* level was employed for all the hydrogen atom geometry optimizations. The B3LYP-D3/6-311G** level was used for single point energy calculations using Gaussian 16 package.60 The Multiwfn 3.8 program was used for all wave function analyses.61 Crystal voids, promolecule density, and energy frameworks were calculated using CrystalExplorer 21.3 software.62
Results and discussion
Crystal structure analysis
The two cocrystals obtained by the slow evaporation method were characterized by SCXRD. The detailed crystallographic information on all cocrystals is listed in Table 1. Hydrogen bond interactions are the main motivations for the formation of these cocrystals. The detailed formation of hydrogen bonds is listed in Table S1.† The formation of hydrogen bonds is accompanied by changes in electron density around atoms close to each other. The electron density around different hydrogen bond motifs is partially shown in Fig. 3. Besides, the cocrystal lattice energies already reported for THE were summarized (Fig. S1†). The two cocrystal lattice energies are in average positions. The cocrystals of THE involved in Fig. S1 are listed in Table S2.†
Table 1 Crystal cell parameters and structure refinement of the cocrystals
Cocrystal |
Formula |
THE-GABA |
THE-ARG-2H2O |
Space group |
Ia |
P1 |
a (Å) |
7.2517(2) |
5.8709(2) |
b (Å) |
21.6583(5) |
7.7140(3) |
c (Å) |
8.7412(3) |
11.1553(3) |
α (deg.) |
90 |
72.564(3) |
β (deg.) |
112.434(3) |
81.718(3) |
γ (deg.) |
90 |
72.804(3) |
Volume (Å3) |
1268.99(7) |
459.59(3) |
Z |
4 |
1 |
Density (g cm−3) |
1.483 |
1.411 |
R1 (I > 2σ(I)) |
0.0416 |
0.0430 |
wR2 (I > 3σ(I)) |
0.1127 |
0.1155 |
CCDC deposition no. |
2307422 |
2307423 |
In THE-GABA, the main hydrogen bond motifs are shown on the left of (a) in Fig. 1. The hydrogen bonds of N4–H4⋯O1S (2.68 Å) and N1S–H1S⋯N3 (3.09 Å) link the THE and GABA molecules together. The oxygen atoms of the carboxylate group of the GABA molecule act as H-bond acceptors towards THE molecule (O1S) and two GABA molecules (O2S, which works as bifurcated acceptors), as shown in Fig. 1. These hydrogen bond interactions assemble THE and GABA molecules. Besides, the two planes where THE and GABA are located separately form 66° in Fig. 2.
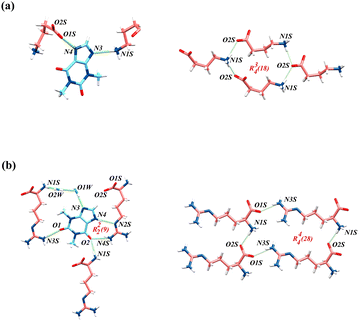 |
| Fig. 1 (a) Hydrogen bonding interactions and hydrogen bond motifs R43(18) of THE-GABA; (b) Hydrogen bonding interactions and hydrogen bond motifs R22(9) and R44(28) of THE-ARG-2H2O. | |
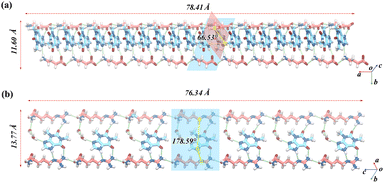 |
| Fig. 2 (a) The stepped-like structure of THE-GABA; (b) the ladder-like structure of THE-ARG-2H2O. | |
In THE-ARG-2H2O, the hydrogen bonds are -affected by the presence of two water molecules. N3 of THE forms a hydrogen bond with O1W–H1WB of one water molecule, which forms a hydrogen bond with O2W of another water molecule at the same time. And O2W forms a hydrogen bond with N1S of ARG. The hydrogen bond interactions connect THE, ARG and two water molecules. Water in organic molecules can be divided into two categories: lattice water and channel water. Fig. S2† illustrates the analysis of the locations of crystallization water molecules, conducted using the water analyzer module of Mercury. And the water in THE-ARG-2H2O belongs to channel water. The probe radius is set to 1.2 Å, which is the approximate molecular radius of one water molecule. The volume of the water molecule is 53.80 Å3, which is 11.7% of unit cell volume. Similar to THE-GABA, the hydrogen bond is formed by the carboxyl group and the amino group of ARG molecules. However, the connection of the amino group of ARG to THE is assisted by two water molecules. Unlike THE-GABA, the carbonyl groups in THE are involved in forming classical hydrogen bonds.63,64 “Classical hydrogen bond” refers to hydrogen bonds that donors do not include groups consisting of C–H. THE is connected with one ARG by N4–H4⋯N2S and N4S–H4S⋯O2 in the motif R22(9). Hydrogen bonds N3S–H3S⋯O1 and O1W–H1W⋯N3 are also involved in the connection of THE and ARG. Furthermore, because THE, ARG, and the two water molecules are almost on the same plane (178.59°), the hydrogen bond interactions assemble them into a ladder-like structure, as shown in the middle of (b) in Fig. 2. From the ladder structure, the hydrogen bonds between the two water molecules significantly contribute to stabilizing THE's binding to the chain structure formed by ARG.65,66
Topological analysis
Topological analysis based on atoms in molecules (AIM) theory can be used to analyze hydrogen bond relationships, in which bond critical point (BCP) and bond path are important parameters. For classical hydrogen bonds, hydrogen bond strength is the focus in the study of intermolecular interactions to distinguish the strength of interactions. Sometimes, bond path length is used to compare the strength of hydrogen bonds, but it is an unreliable criterion for judgment. According to,67 the electron density at BCP has an optimal correlation with the strength of hydrogen bonds. This study uses this method to analyze the strength of each hydrogen bond formed with THE in each cocrystal while comparing it to the bond path length. The results are shown in Fig. 3. For example, in cocrystal THE-ARG-2H2O, imidazole in THE forms a hydrogen bond with the guanidine group in ARG with a bond path length of 2.915 Å, which is supposed to be a weak hydrogen bond as judged from the bond path length. However, the results show that this hydrogen bond is the strongest in Fig. 3(b) because of the highest electron density at BCP.
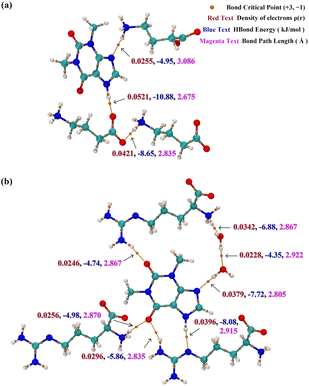 |
| Fig. 3 Topological analysis of the electronic density of THE-GABA (a) and THE-ARG-2H2O (b). | |
PXRD analysis
PXRD is mainly used for the solid-phase analysis of powder.68 The newly formed cocrystals often have specific PXRD patterns due to their unique crystal structure. Therefore, PXRD patterns of the cocrystal are compared with patterns calculated from the SCXRD structure (Fig. 4: left). To facilitate intuitive observation of the variation of the diffraction peaks, the variation in the characteristic diffraction peaks is labeled as “
”. The pure phase of API and CCFs PXRD patterns are included. It is evident that the patterns calculated from the SCXRD structure (labeled with the prefix “THEO”) align closely with the experimental patterns. Notably, the primary characteristic peaks of THE and CCFs disappear, and new characteristic diffraction peaks are generated, signifying the creation of new phases. Water molecules are presented in THE-ARG-2H2O. The removal of water molecules at elevated temperatures was conducted to determine the feasibility of obtaining anhydrous THE–ARG. Upon equilibrating the powder of THE-ARG-2H2O at 130 °C for 30 minutes, the PXRD pattern of the powder exhibited peaks corresponding to THE and ARG. The anhydrous THE–ARG was ultimately not acquired.
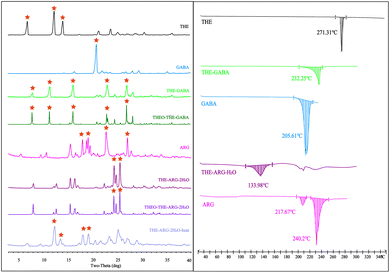 |
| Fig. 4 PXRD patterns (left) and DSC (right) of API, CCFs, and the corresponding cocrystals (characteristic peaks are marked as ). | |
Thermal analysis
DSC is a common detection technique to identify thermodynamic changes in cocrystal and crystalline forms.69 The DSC curves of cocrystals are shown in Fig. 4 (right). THE-GABA exhibited a melting endothermic peak at 232.25 °C, whereas THE-ARG-2H2O showed a corresponding peak at 133.98 °C. Among them, the endothermic peak of THE-ARG-2H2O at 133.98 °C indicates the release of water, and then melting occurs after 190 °C. The peaks of the cocrystal exhibit apparent differences from API or CCFs, which implies the formation of cocrystals. TG (Fig. S3:† right) analysis was applied to investigate the crystalline solvent presented in the structures. Therefore, based on the results of TG analysis, the cocrystal THE-GABA does not contain solvent or water. For THE-ARG-2H2O, the TG curve shows a weight loss step in the temperature range of 80–140 °C, and the mass loss rate is 8.68%, consistent with the theoretical mass loss rate of 9.23% of two water molecules.
IR analysis
The formation of the hydrogen bonding interaction between API and CCFs led to changes in the IR spectra of the cocrystals compared with the IR spectra of API and CCFs.70 SCXRD data show that the sites forming hydrogen bonds of THE mainly are on the secondary amine and imine group of the imidazole ring and carbonyl of the pyrimidine ring. The intermolecular hydrogen bonding interaction led to alterations in the IR spectra of the cocrystals, specifically in the wavenumber bands of 3500–3100 cm−1 (νN–H), 3400–3300 cm−1 (νC
N) and 1690–1640 cm−1 (νC
O). Compared with THE, the cocrystals of vibration frequency generally moved to lower frequencies. The main vibrational data of the cocrystals and their preliminary attribution are shown in Table S3, and the IR spectra are shown in Fig. S3 (left).†
DVS analysis
Hygroscopicity can be defined as “a phenomenon that occurs when a certain amount of water vapor exposed to a solid at a given temperature and relative humidity is taken up and retained utilizing noncovalent interactions, particularly hydrogen bonding, with some effect on the surface and/or bulk properties of the solid”.71,72 Hygroscopicity may be attributed to the forming of hydrogen bonds between functional groups exposed to air on a solid surface and water molecules and voids in the crystal structure.73 ARG and GABA as zwitterions (Schemes 1 and 2) can cause strong ion–water interaction, so the hygroscopic properties of API, amino acid, and cocrystals were examined.
Sorption and desorption of THE, GABA, ARG, and their cocrystals are illustrated in Fig. S4.† The results from the DVS indicate that the anhydrous forms of THE (BAPLOT01) and ARG (ARGIND11) exhibit negligible hygroscopicity, with values of 0.075% and 0.786%, respectively. In contrast, GABA (GAMBUT01) displays significant hygroscopic characteristics, with a much higher value of 63.33%. Notably, the formed cocrystals reduce the hygroscopicity of GABA and ARG to varying degrees.
The hygroscopicity of THE-GABA, measured at 0.064%, falls below the standard threshold of 0.2% for hygroscopicity, categorizing it as having negligible or virtually no hygroscopic properties. Although the moisture weight gains of the THE-ARG-2H2O (0.426%) are higher than that of THE, it only belongs to the substance with slight hygroscopicity. The crystal voids were examined (Fig. 5 and Table 2) about the potential presence of voids following the development of the co-crystals, which could let the ingress of water molecules, ultimately compromising the structure.
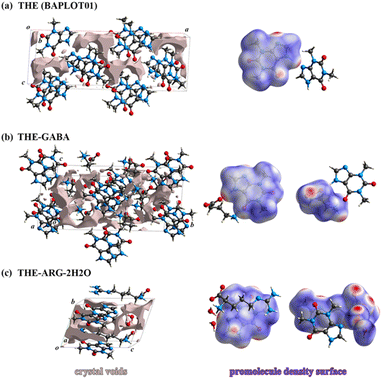 |
| Fig. 5 Crystal voids occupied in the unit cell and promolecule density surface of THE (a), cocrystal THE-GABA (b), and THE-ARG-2H2O (c) at a 0.002 a.u. isosurface. | |
Table 2 The information of the void surface and promolecule density (isovalue 0.002 e au−3)
Compounds |
Voids (Å3) |
Voids (%) |
Promolecule density (Å3) |
Promolecule density (%) |
Moisture weight (%) |
THE |
79.80 |
10.0 |
174.24 |
87.0 |
0.0749 |
THE-GABA |
76.98 |
6.1 |
291.79 |
92.1 |
0.0660 |
THE-ARG-2H2O |
44.82 |
9.8 |
412.43 |
89.7 |
0.428 |
Crystal voids analysis
The void space refers to the unoccupied areas within a crystal structure where solvent molecules can attach or be incorporated during dissolution or solvation processes. In a novel cocrystal complex of THE and malonic acid,14 the hygroscopicity of THE and its cocrystals were linked to the voids and promolecule density74 of the respective crystals. The relevant calculation results are listed in Table 2 and shown in Fig. 5. Generally, smaller voids or a higher promolecule density in the crystal correlate with reduced hygroscopicity. This correlation has been confirmed in the case of the THE-GABA cocrystal. However, the dihydrate cocrystal, THE-ARG-2H2O, deviates from this pattern, likely due to the inherent water content of the hydrate itself.
Solubility and IDR
High solubility and fast dissolution are essential for optimal pharmacokinetics and improved therapeutic efficacy.75
The IDR parameter is a good indicator to predict bioavailability performance. The IDR results of THE cocrystals are presented in Fig. 6. At pH 7.0, 6.8, 4.5, and 1.2, THE-ARG-2H2O and THE-GABA significantly increase the dissolution rate of THE in the order THE-ARG-2H2O > THE-GABA.
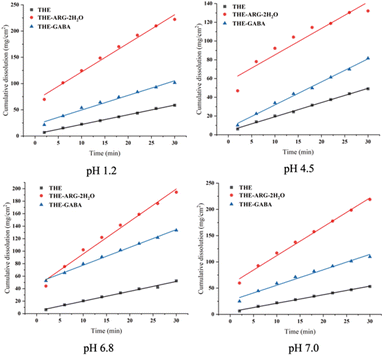 |
| Fig. 6 IDR results of THE and cocrystals. | |
Fig. 7 and Table 3 show the solubility, final solid powder and post-experimental pH results of THE (THE form II, CSD ref-code BAPLOT) at pH = 1.2, pH = 4.5, pH = 6.8, and pH = 7.0 buffered solutions. Solubility experiments were carried out by the suspension stirring method at 37 °C for 48 h. The solubilities of THE-ARG-2H2O are higher than that of THE at pH = 6.8 and pH = 7.0 buffered solutions, around five times and 3.2 times, respectively. After the buffered solutions were absorbed, the final powder in the vial was subjected to PXRD testing. Interestingly, it was observed that only at pH levels of 7.0 and 6.8 did the PXRD patterns of the final powder closely match those of THE-ARG-2H2O. In contrast, whether it is THE-ARG-2H2O at pH 1.2 and pH 4.5, or THE-GABA and THE in the four buffered solutions, the final powder predominantly transforms into a monohydrate of THE. Concurrently, it is also noted that these powder patterns largely correspond with THE in supersaturated water. The pH was measured after the experiments. The PH values of THE-GABA and THE are close to each other in the four media solutions. In contrast, in THE-ARG-2H2O, the data of pH in four media solutions are greater than THE, with 6.8 being close to 7.0. This may be because the pKa value of ARG is greater than those of GABA and THE. The pH values in Table 3 suggest that the buffer capacity was overwhelmed in some cases. This is all the more reason to list the suspension composition in the Experiment section.
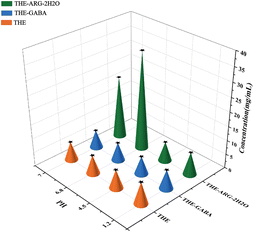 |
| Fig. 7 Solubility results of THE and cocrystals. | |
Table 3 Solubility of THE and cocrystals (mean ± SD mg mL−1, n = 3)
|
Compound |
THE |
THE-GABA |
THE-ARG-2H2O |
pH 1.2 |
Saturation concentration |
7.8 ± 0.09 |
6.81 ± 0.06 |
8.05 ± 0.18 |
Post-experimental pH |
1.84 |
2.04 |
3.21 |
Final solid powder |
Monohydrate of THE |
Monohydrate of THE |
Monohydrate of THE |
pH 4.5 |
Saturation concentration |
6.67 ± 0.17 |
6.42 ± 0.58 |
6.95 ± 0.059 |
Post-experimental pH |
4.80 |
4.82 |
5.53 |
Final solid powder |
Monohydrate of THE |
Monohydrate of THE |
Monohydrate of THE |
pH 6.8 |
Saturation concentration |
6.68 ± 0.12 |
6.49 ± 0.04 |
34.83 ± 1.00 |
Post-experimental pH |
6.90 |
6.88 |
8.92 |
Final solid powder |
Monohydrate of THE |
Monohydrate of THE |
Cocrystal |
pH 7.0 |
Saturation concentration |
6.66 ± 0.31 |
6.42 ± 0.26 |
21.55 ± 2.09 |
Post-experimental pH |
6.27 |
6.28 |
8.93 |
Final solid powder |
Monohydrate of THE |
Monohydrate of THE |
Cocrystal |
The process of solubility occurs when the cocrystals enter the aqueous solution, the crystal surface is surrounded by water molecules, which also enter the void space thereby structurally disintegrating. Since the molecules generally tend to exist in a more stable state, the more water-soluble CCF leaves the lattice first into the aqueous medium.76 The results of IDR and solubility experiments show that the dissolution rate of THE-GABA is significantly improved compared to that of THE, but no change is notable in solubility. This may be because the presence of GABA could not limit the transformation of THE polymorph II to THE monohydrate during standing at equilibrium solubility. In THE-ARG-2H2O, the guanidine group of ARG exhibits sensitivity to hydrogen ion concentration, indicating that pH (H+ concentration) influences the state of the cocrystals, which exist in a cocrystal state at pH 6.8 and 7.0.
Furthermore, the conversion of THE polymorph II with water to THE monohydrate necessitated supersaturation conditions that were discovered serendipitously. The supersaturated and unsaturated states of THE in water were compared. As observed in Fig. 8, it is found that under identical conditions in water, only the supersaturated state of THE (polymorph II) primarily converted into THE monohydrate. The PXRD pattern observed in the unsaturated state remains consistent with the API form of THE.
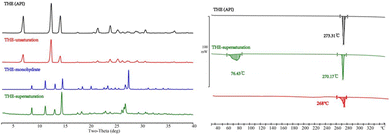 |
| Fig. 8 PXRD and DSC patterns of THE. | |
Permeability
Oral drug bioavailability is mainly affected by the permeability of drugs through the gastrointestinal membrane and the solubility/dissolution of drugs in the gastrointestinal environment.1 Therefore, intestinal pH 6.8 was simulated to conduct permeability studies on THE and cocrystals. The results are shown in Fig. 9. Taking into account the importance of the apparent permeability coefficient (Papp) values for the overall evaluation of the permeability of drug-like compounds. The Papp of THE is 142.40 × 10−6 cm s−1, the Papp of THE-GABA is 85.59 × 10−6 cm s−1, and the Papp of THE-ARG-2H2O is 31.32 × 10−6 cm s−1. In Fig. 9, THE-ARG-2H2O has basically reached saturation penetration at 60 min, THE-GABA has basically reached saturation at 180 min, and THE has not reached saturation after 250 min. Therefore, both cocrystals improve the permeability of THE, especially THE-ARG-2H2O.
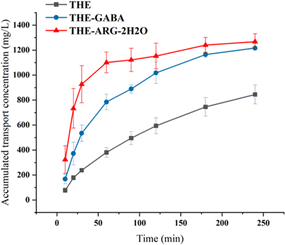 |
| Fig. 9 Permeability results of THE and cocrystals. | |
Hirshfeld surface and 3D energy frameworks
The nature and individual contributions of each intermolecular interaction within a crystal lattice can be illustrated by the Hirshfeld surface. The fingerprint on the right and percentage contributions to the Hirshfeld surface revealed that H⋯H contacts have maximum contributions in both cocrystals. In Fig. 10, the deep red spots indicate sites where hydrogen bonds were formed, and the shade of color corresponds to the strength of the interaction. In THE-GABA, O⋯H (16.2%), H⋯O (13.2%), and N⋯H (6.8%) interactions have high contributions to the Hirshfeld surface. In THE-ARG-2H2O, O⋯H (13.4%), N⋯H (11.1%), and H⋯O (5.9%) interactions have notable contributions to the Hirshfeld surface. The H–O/O–H and N–H/H–N contributions of THE, THE-GABA and THE-ARG-2H2O were 40.3%, 35.9%, and 38.7%, respectively.
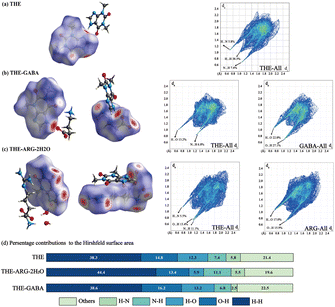 |
| Fig. 10 Hirshfeld surface (mapped with dnorm) of THE (a), THE-GABA (b) and THE-ARG-2H2O (c) and percentage contributions to the Hirshfeld surface area (d). | |
The 3D energy framework can be utilized to analyze the energy interaction between molecules. This total interaction energy is calculated per unit cell. The energy framework calculations are based on molecular wave functions, generating electron densities using the CE-B3LYP/6-31G (d, p) method. The energy frameworks for the molecule, encompassing coulomb, dispersion, and total energies, are depicted using cylinders of different colors. These cylinders were scaled (tube size) by a factor of 100 and have a cut-off energy of −5 kJ mol−1. The cylinder's diameter varies according to the strength of the interaction. As illustrated in Fig. S5,† the calculation results reveal that Coulomb energy predominates in THE and THE-ARG-2H2O. However, dispersion energy is also significant in THE and THE-ARG-2H2O. The relationship between Coulomb and dispersion energies is reversed in the THE-GABA cocrystal, with dispersion energy playing the leading role.
Conclusions
In this study, two novel cocrystals with amino acids were synthesized successfully, THE-GABA and THE-ARG-2H2O, using solvent-assisted grinding and slow evaporation methods. To identify the predominant cocrystal form, a range of characterizations were conducted, including SCXRD, PXRD, DSC, TGA, and IR, as well as solubility, IDR, permeability and hygroscopicity evaluations. Topological analysis, Hirshfeld surface and 3D energy frameworks were used to analyze intermolecular interaction forces and Crystal voids analysis was used to analyze hygroscopicity. Although THE-GABA demonstrates an enhancement in dissolution rate and permeability, THE-ARG-2H2O displays a more pronounced rise. This may result from variations in the solubility of CCFs. In the Hirshfeld surface results, a smaller percentage of O–H/H–O and N–H/H–N, suggesting weaker intermolecular hydrogen bonding, may explain the higher solubility of THE-ARG-2H2O. The solubility experiments indicated that the solubility of THE-ARG-2H2O enhanced solely at pH levels 6.8 and 7.0. The guanidinium group of ARG is sensitive to hydrogen ion concentration, leading to minimal enhancement in solubility at pH 4.5 and 1.2. Consequently, APIs or CCFs with guanidine groups must consider the influence of pH during polymorphic or cocrystal activity. In addition, it was unexpectedly found that the conversion of THE polymorph II to THE monohydrate requires supersaturation conditions (water solution). Therefore, the saturation state of THE must be considered during pharmacological operations involving water solution related to THE. Our study provides experience and examples for exploring the structure–property relationships in pharmaceutical cocrystals. THE-ARG-2H2O, as a superior cocrystal form, significantly enhances the solubility of THE while remaining stable, offering valuable material for further in vivo studies.
Data availability
The dates of the compounds are available from the authors.
Author contributions
Conceptualization, L. Z. and Y. L.; methodology, Q. L. and Y. X. software, D. Y. and S. L.; validation, D. Y., S. L. and Y. X.; formal analysis, Q. L. and Y. X.; investigation, Y. X. and Q. L.; resources, Q. L. and Z. W; data curation, Z. W. and Q. L; writing—original draft preparation, Q. L.; writing—review and editing, D. Y. and L. Z.; visualization, S. Y.; supervision, L. Z., D. Y. and Y. L.; project administration, G. D.; funding acquisition, Y. L., L. Z. and D. Y. All authors have read and agreed to the published version of the manuscript.
Conflicts of interest
There are no conflicts to declare.
Acknowledgements
This research was funded by the National Natural Science Foundation of China (Grant No. 22278443), CAMS Innovation Fund for Medical Sciences (Grant No. 2022-I2M-1-015), the Key R&D Program of Shan Dong Province (Grant No. 2021ZDSYS26), Chinese Pharmacopoeia Commission Drug Standard Promoting Fund (Grant No. 2023Y11), Independent Innovation and Achievement Transformation Plan Project of Zaozhuang City (Grant No. 2022GH15), the Xinjiang Uygur Autonomous Region Innovation Environment Construction Special Fund and Technology Innovation Base Construction Key Laboratory Open Project (Grant No. 2022D04016) and 2021 Tengzhou Talent Project.
References
- P. J. Barnes, Theophylline, Am. J. Respir. Crit. Care Med., 2013, 188(8), 901–906 CrossRef CAS PubMed.
- M. Weinberger and L. Hendeles, Theophylline in asthma, N. Engl. J. Med., 1996, 334(21), 1380–1388 CrossRef CAS PubMed.
- K. F. Rabe, H. Magnussen and G. Dent, Theophylline and selective PDE inhibitors as bronchodilators and smooth muscle relaxants, Eur. Respir. J., 1995, 8(4), 637–642 CrossRef CAS PubMed.
- L. L. Gong, L. D. Du and G. H. Du, Theophylline, Natural Small Molecule Drugs from Plants Ch., 2018, vol. 78, pp. 469–474 Search PubMed.
- J. D. Journey and T. P. Bentley, Theophylline toxicity, StatPearls, 2018 Search PubMed.
- Y. Ma, D. Jiang, J. Meng, M. Li, H. Zhao, Y. Wang and L. Wang, Theophylline: a review of population pharmacokinetic analyses, J. Clin. Pharm. Therapeut., 2016, 41(6), 594–601 CrossRef CAS PubMed.
- J. B. Peter, Theophylline, Am. J. Respir. Crit. Care Med., 2013, 188(8), 1–19 Search PubMed.
- K. Fucke, G. J. McIntyre, C. Wilkinson, M. Henry, J. A. Howard and J. W. Steed, New insights into an old molecule: interaction energies of theophylline crystal forms, Cryst. Growth Des., 2012, 12(3), 1395–1401 CrossRef CAS.
- K. Matsuo and M. Matsuoka, Solid-state polymorphic transition of theophylline anhydrate and humidity effect, Cryst. Growth Des., 2007, 7(2), 411–415 CrossRef CAS.
- L. Seton, D. Khamar, I. J. Bradshaw and G. A. Hutcheon, Solid state forms of theophylline: presenting a new anhydrous polymorph, Cryst. Growth Des., 2010, 10(9), 3879–3886 CrossRef CAS.
- H. Leslie, W. Miles, S. Stanley and E. Elliot, Safety and efficacy of theophylline in children with asthma, J. Pediatr., 1992, 120(2), 177–183 CrossRef PubMed.
- M. Weinberger and L. Hendeles, Therapeutic effect and dosing strategies for theophylline in the treatment of chronic asthma, J. Allergy Clin. Immunol., 1986, 78(4), 762–768 CrossRef CAS PubMed.
- L. P. Ji, R. M. Chang, Q. Q. Xu, J. J. Chen, S. F. Lu, H. X. Chen, S. M. Lu and T. D. Liang, Analysis of adverse reactions and plasma concentrations of oral commonly used dose sustained-release theophylline in the elderly, J. Emerg. Med., 2020, 25(1), 3 Search PubMed.
- S. A. Stanton, J. J. Du, F. Lai, G. Stanton, B. A. Hawkins, J. A. Ong, P. W. Groundwater, J. A. Platts and D. E. Hibbs, Understanding Hygroscopicity of Theophylline via a Novel Cocrystal Polymorph: A Charge Density Study, J. Phys. Chem. A, 2021, 125(45), 9736–9756 CrossRef CAS PubMed.
- G. Bolla, B. Sarma and A. K. Nangia, Crystal engineering of pharmaceutical cocrystals in the discovery and development of improved drugs, Chem. Rev., 2022, 122(13), 11514–11603 CrossRef CAS PubMed.
- M. A. Yousef and V. R. Vangala, Pharmaceutical cocrystals: molecules, crystals, formulations, medicines, Cryst. Growth Des., 2019, 19(12), 7420–7438 CrossRef CAS.
- R. Shaikh, R. Singh, G. M. Walker and D. M. Croker, Pharmaceutical Cocrystal Drug Products: An Outlook on Product Development, Trends Pharmacol. Sci., 2018, 39(12), 1033–1048 CrossRef CAS PubMed.
- N. Qiao, M. Li, W. Schlindwein, N. Malek, A. Davies and G. Trappitt, Pharmaceutical cocrystals: An overview, Int. J. Pharm., 2011, 419(1–2), 1–11 CrossRef CAS PubMed.
- M. Gryl, S. Cenedese and K. Stadnicka, Crystal Engineering and Charge Density Study of Pharmaceutical Nonlinear Optical Material: Melamine-Barbital Co-Crystal, J. Phys. Chem. C, 2014, 119(1), 590–598 CrossRef.
- B. Dittrich, Is there a future for topological analysis in experimental charge-density research? Acta Crystallographica Section B Structural Science, Acta Crystallogr., Sect. B, 2017, 73(3), 325–329 CrossRef CAS PubMed.
- W. Kuang, S. Ji, Y. Wei, J. Zhang and P. Lan, A new 1:1 cocrystal of lamotrigine and 1,2,3,6-hydrophthalimide: discovery, characterization, and construction of ternary phase diagrams, CrystEngComm, 2020, 22(15), 2681–2688 RSC.
- G. Kuminek, K. L. Cavanagh, M. F. M. da Piedade and N. Rodríguez-Hornedo, Posaconazole Cocrystal with Superior Solubility and Dissolution Behavior, Cryst. Growth Des., 2019, 19(11), 6592–6602 CrossRef CAS.
- J. Wang, X.-L. Dai, T.-B. Lu and J.-M. Chen, Temozolomide–Hesperetin Drug–Drug Cocrystal with Optimized Performance in Stability, Dissolution, and Tabletability, Cryst. Growth Des., 2021, 21(2), 838–846 CrossRef CAS.
- D. Bernasconi, S. Bordignon, F. Rossi, E. Priola, C. Nervi, R. Gobetto, D. Voinovich, D. Hasa, N. T. Duong, Y. Nishiyama and M. R. Chierotti, Selective Synthesis of a Salt and a Cocrystal of the Ethionamide–Salicylic Acid System, Cryst. Growth Des., 2019, 20(2), 906–915 CrossRef.
- M.-H. Qi, H. Li, B. Zhu, M. Hong and G.-B. Ren, Cocrystals of Oxymatrine: Reducing Hygroscopicity and Affecting the Dissolution Rate, Cryst. Growth Des., 2021, 21(7), 3874–3888 CrossRef CAS.
- T. Friščić, L. Fábián, J. C. Burley, D. G. Reid, M. J. Duer and W. Jones, Exploring the relationship between cocrystal stability and symmetry: is Wallach’s rule applicable to multi-component solids?, Chem. Commun., 2008, 14, 1644–1646 RSC.
- H. Liu, H. C. S. Chan, X. Yu, J. Li, J. Li and Z. Zhou, Two Polymorphic Cocrystals of Theophylline with Ferulic Acid, Cryst. Growth Des., 2023, 23(6), 4448–4459 CrossRef CAS.
- S. Darwish, J. Zeglinski, G. R. Krishna, R. Shaikh, M. Khraisheh, G. M. Walker and D. M. Croker, A New 1:1 Drug-Drug Cocrystal of Theophylline and Aspirin: Discovery, Characterization, and Construction of Ternary Phase Diagrams, Cryst. Growth Des., 2018, 18(12), 7526–7532 CrossRef CAS.
- Z.-L. Wang and L.-H. Wei, Theophylline–2,4-dihydroxybenzoic acid–water (1/1/1), Acta Crystallogr., Sect. E:Struct. Rep. Online, 2007, 63(4), 1681–1682 CrossRef.
- S. Aitipamula, P. S. Chow and R. B. H. Tan, Trimorphs of a pharmaceutical cocrystal involving two active pharmaceutical ingredients: potential relevance to combination drugs, CrystEngComm, 2009, 11(9), 1823–1827 RSC.
- F. Fischer, M. U. Schmidt, S. Greiser and F. Emmerling, The challenging case of the theophylline–benzamide cocrystal, Acta Crystallogr., Sect. C: Struct. Chem., 2016, 72(3), 217–224 CrossRef CAS PubMed.
- A. O. L. Évora, R. A. E. Castro, T. M. R. Maria, M. Ramos Silva, J. Canotilho and M. E. S. Eusébio, Lamotrigine: Design and synthesis of new multicomponent solid forms, Eur. J. Pharm. Sci., 2019, 129, 148–162 CrossRef PubMed.
- P. Sanphui, S. S. Kumar and A. Nangia, Pharmaceutical Cocrystals of Niclosamide, Cryst. Growth Des., 2012, 12(9), 4588–4599 CrossRef CAS.
- D. Markad and S. K. Mandal, An exploration into the amide–pseudo amide hydrogen bonding synthon between a new coformer with two primary amide groups and theophylline, CrystEngComm, 2017, 19(47), 7112–7124 RSC.
- A. Delori, P. T. A. Galek, E. Pidcock, M. Patni and W. Jones, Knowledge-based hydrogen bond prediction and the synthesis of salts and cocrystals of the anti-malarial drug pyrimethamine with various drug and GRAS molecules, CrystEngComm, 2013, 15, 15 RSC.
- I. Nugrahani and M. A. Jessica, Amino Acids as the Potential Co-Former for Co-Crystal Development: A Review, Molecules, 2021, 26, 11 Search PubMed.
- Y. Song, L. Y. Wang, F. Liu, Y. T. Li, Z. Y. Wu and W. Yan, Simultaneously enhancing the in vitro/in vivo performances of acetazolamide using proline as a zwitterionic coformer for cocrystallization, CrystEngComm, 2019, 21, 3064–3073 RSC.
- S. J. Dengale, H. Grohganz, T. Rades and K. Löbmann, Recent advances in co-amorphous drug formulations, Adv. Drug Delivery Rev., 2016, 100, 116–125 CrossRef CAS PubMed.
- K. Löbmann, H. Grohganz, R. Laitinen, C. Strachan and T. Rades, Amino acids as co-amorphous stabilizers for poorly water soluble drugs–Part 1: Preparation, stability and dissolution enhancement, Eur. J. Pharm. Biopharm., 2013, 85(3), 873–881 CrossRef PubMed.
- I. Nugrahani and M. A. Jessica, Amino acids as the potential co-former for co-crystal development: a review, Molecules, 2021, 26(11), 3279 CrossRef CAS PubMed.
- A. Shete, S. Murthy, S. Korpale, A. Yadav, S. Sajane, S. Sakhare and R. Doijad, Cocrystals of itraconazole with amino acids: Screening, synthesis, solid state characterization, in vitro drug release and antifungal activity, J. Drug Delivery Sci. Technol., 2015, 28, 46–55 CrossRef CAS.
- D. Rambabu, J. Satyanarayana, V. G. Saraswatula, N. Ravikumar, S. A. Kamalakaran, G. Gopikrishna and K. A. Kumar, Novel cocrystals/molecular salts of mesalamine to be used as improved anti-inflammatory drug, WIPO Patent, WO2012090224A1, 2012 Search PubMed.
- A. Wróblewska, J. Śniechowska, S. Kaźmierski, E. Wielgus, G. D. Bujacz, G. Mlostoń, A. Chworos, J. Suwara and M. J. Potrzebowski, Application of 1-hydroxy-4, 5-dimethyl-imidazole 3-oxide as coformer in formation of pharmaceutical cocrystals, Pharmaceutics, 2020, 12(4), 359 CrossRef PubMed.
- J. C. Krantz, J. M. Holbert, H. K. Iwamoto and C. J. Carr, Sodium Theophylline Glycinate, J. Am. Pharm. Assoc., 1947, 36(8), 248–250 CrossRef CAS PubMed.
- P. Wang, M. Qi, D. Zhong and L. L. Fang, Pharmacokinetics of a new sustained-release formulation of theophylline sodium glycerinate in healthy subjects with a new asymmetric dosage regimen, Biomed. Chromatogr., 2003, 17(1), 58–61 CrossRef CAS PubMed.
- D.-H. Ngo and T. S. Vo, An Updated Review on Pharmaceutical Properties of Gamma-Aminobutyric Acid, Molecules, 2019, 24, 15 CrossRef PubMed.
- D. T. Plante, J. E. Jensen, L. Schoerning and J. W. Winkelman, Reduced γ-Aminobutyric Acid in Occipital and Anterior Cingulate Cortices in Primary Insomnia: a Link to Major Depressive Disorder?, Neuropsychopharmacology, 2012, 37(6), 1548–1557 CrossRef CAS PubMed.
- R. H. Böger and S. M. Bode-Böger, The Clinical Pharmacology of L-Arginine, Annu. Rev. Pharmacol., 2001, 41(1), 79–99 CrossRef PubMed.
- H. Tapiero, G. Mathé, P. Couvreur and K. D. I. Tew, Arginine, Biomed. Pharmacother., 2002, 56(9), 439–445 CrossRef CAS PubMed.
- H. Puschmann, L. J. Bourhis, O. V. Dolomanov, R. J. Gildea and J. A. Howard, OLEX2-a complete package for molecular crystallography, Acta Crystallogr., Sect. A, 2011, 67, C593 Search PubMed.
- H. Puschmann and O. Dolomanov, OLEX2: TEACHING NEW SOFTWARE OLD AND NEW TRICKS, Acta Crystallogr., Sect. A, 2019, 75, e766 Search PubMed.
- F. Kleemiss, O. V. Dolomanov, M. Bodensteiner, N. Peyerimhoff, L. Midgley, L. J. Bourhis, A. Genoni, L. A. Malaspina, D. Jayatilaka, J. L. Spencer, F. White, B. Grundkötter-Stock, S. Steinhauer, D. Lentz, H. Puschmann and S. Grabowsky, Accurate crystal structures and chemical properties from NoSpherA2, Chem. Sci., 2021, 12(5), 1675–1692 RSC.
- L. Midgley, L. J. Bourhis, O. V. Dolomanov, S. Grabowsky, F. Kleemiss, H. Puschmann and N. Peyerimhoff, Vanishing of the atomic form factor derivatives in non-spherical structural refinement–a key approximation scrutinized in the case of Hirshfeld atom refinement, Acta Crystallogr., Sect. A:Found. Adv., 2021, 77(6), 519–533 CrossRef CAS PubMed.
- J. Lübben, C. M. Wandtke, C. B. Hübschle, M. Ruf, G. M. Sheldrick and B. Dittrich, Aspherical scattering factors for SHELXL–model, implementation and application, Acta Crystallogr., Sect. A:Found. Crystallogr., 2019, 75(1), 50–62 CrossRef PubMed.
- L. J. Barbour, X-Seed—A software tool for supramolecular crystallography, J. Supramol. Chem., 2001, 1(4–6), 189–191 CrossRef CAS.
- C. F. Macrae, I. Sovago, S. J. Cottrell, P. T. Galek, P. McCabe, E. Pidcock, M. Platings, G. P. Shields, J. S. Stevens and M. Towler, Mercury 4.0: From visualization to analysis, design and prediction, J. Appl. Crystallogr., 2020, 53(1), 226–235 CrossRef CAS PubMed.
- S. Emami, R. Ghafari and E. Manafzadeh, Solid-State Interaction of Pharmaceutical Cocrystals with Water Vapor, Cryst. Growth Des., 2021, 21(8), 4805–4820 CrossRef CAS.
- M. Humar, R. Repič, D. Kržišnik, B. Lesar, R. Cerc Korošec, C. Brischke, L. Emmerich and G. Rep, Quality Control of Thermally Modified Timber Using Dynamic Vapor Sorption (DVS) Analysis, Forests, 2020, 11, 6 Search PubMed.
- R. F. W. Bader, Atoms in molecules, Acc. Chem. Res., 1985, 18(1), 9–15 CrossRef CAS.
- M. Frisch, G. Trucks, H. Schlegel, G. Scuseria, M. Robb, J. Cheeseman, G. Scalmani, V. Barone, G. Petersson and H. Nakatsuji, Gaussian 16, Revision A. 03, Gaussian, Inc., Wallingford CT, G16 A.03, 2016 Search PubMed.
- T. Lu and F. Chen, Multiwfn: A multifunctional wavefunction analyzer, J. Comput. Chem., 2012, 33(5), 580–592 CrossRef CAS PubMed.
- P. R. Spackman, M. J. Turner, J. J. McKinnon, S. K. Wolff, D. J. Grimwood, D. Jayatilaka and M. A. Spackman, CrystalExplorer: A program for Hirshfeld surface analysis, visualization and quantitative analysis of molecular crystals, J. Appl. Crystallogr., 2021, 54(3), 1006–1011 CrossRef CAS PubMed.
- W. M. Latimer and W. H. Rodebush, Polarity and ionization from the standpoint of the Lewis theory of valence, J. Am. Chem. Soc., 1920, 42(7), 1419–1433 CrossRef CAS.
- Z. S. Derewenda, On the centennials of the discoveries of the hydrogen bond and the structure of the water molecule: the short life and work of Eustace Jean Cuy (1897–1925), Acta Crystallogr., Sect. A:Found. Adv., 2021, 77(5), 362–378 CrossRef CAS PubMed.
- H. Yu, B. Zhang, M. Liu, W. Xing, K. Hu, S. Yang, G. He, N. Gong, G. Du and Y. Lu, Design, Preparation, Characterization and Evaluation of Five Cocrystal Hydrates of Fluconazole with Hydroxybenzoic Acids, Pharmaceutics, 2022, 14, 11 Search PubMed.
- S. Aitipamula, P. S. Chow and R. B. H. Tan, Co-crystals of caffeine and piracetam with 4-hydroxybenzoic acid: Unravelling the hidden hydrates of 1: 1 co-crystal, CrystEngComm, 2012, 14, 7 Search PubMed.
- S. Emamian, T. Lu, H. Kruse and H. Emamian, Exploring Nature and Predicting Strength of Hydrogen Bonds: A Correlation Analysis Between Atoms-in-Molecules Descriptors, Binding Energies, and Energy Components of Symmetry-Adapted Perturbation Theory, J. Comput. Chem., 2019, 40(32), 2868–2881 CrossRef CAS PubMed.
- S. Alam, S. Patel and A. K. Bansal, Effect of sample preparation method on quantification of polymorphs using PXRD, Pharm. Dev. Technol., 2009, 15(5), 452–459 CrossRef PubMed.
- J. D. Menczel, L. Judovits and R. B. Prime, Differential scanning calorimetry (DSC), Thermal Analysis of Polymers: Fundamentals and Applications, 2009, pp. 7–239 Search PubMed.
- S. Ishihara, Y. Hattori and M. Otsuka, MCR-ALS analysis of IR spectroscopy and XRD for the investigation of ibuprofen - nicotinamide cocrystal formation, Spectrochim. Acta, Part A, 2019, 221, 117142 CrossRef CAS PubMed.
- A. W. Newman, S. M. Reutzel-Edens and G. Zografi, Characterization of the hygroscopic properties of active pharmaceutical ingredients, J. Pharm. Sci., 2008, 97(3), 1047–1059 CrossRef CAS PubMed.
- C. Ahlneck and G. Zografi, The molecular basis of moisture effects on the physical and chemical stability of drugs in the solid state, Int. J. Pharm., 1990, 62(2–3), 87–95 CrossRef CAS.
- D. P. Kale, B. Ugale and C. M. Nagaraja, Molecular basis of water sorption behavior of rivaroxaban-malonic acid cocrystal, Mol. Pharm., 2019, 16(7), 2980–2991 CrossRef CAS PubMed.
- A. S. Mitchell and M. A. Spackman, Molecular surfaces from the promolecule: A comparison with Hartree-Fockab initio electron density surfaces, J. Comput. Chem., 2000, 21(11), 933–942 CrossRef CAS.
- J. Varshosaz, Biopharmaceutical characterization of oral theophylline and aminophylline tablets. Quantitative correlation between dissolution and bioavailability studies, Eur. J. Pharm. Biopharm., 2000, 50(2), 301–306 CrossRef CAS PubMed.
- D. D. Bavishi and C. H. Borkhataria, Spring and parachute: How cocrystals enhance solubility, Prog. Cryst. Growth Charact. Mater., 2016, 62(3), 1–8 CrossRef CAS.
Footnotes |
† Electronic supplementary information (ESI) available. CCDC 2307422 and 2307423. For ESI and crystallographic data in CIF or other electronic format see DOI: https://doi.org/10.1039/d4ra06804a |
‡ Qi Li and Yifei Xie contributed to the work equally as joint first authors. |
|
This journal is © The Royal Society of Chemistry 2024 |
Click here to see how this site uses Cookies. View our privacy policy here.