DOI:
10.1039/D4RA07008A
(Paper)
RSC Adv., 2024,
14, 34811-34815
Electrophilic aromatic substitution of electron-rich arenes with N-fluorobenzenesulfonimide (NFSI) as an electrophile†
Received
29th September 2024
, Accepted 25th October 2024
First published on 31st October 2024
Abstract
An efficient amidation of electron-rich arenes using NFSI as a nitrogen source has been successfully disclosed. This amidation process can be easily conducted at elevated temperatures, without the need for catalysts or additives. A wide range of arenes substituted with hydroxy, alkoxy, or carbonyl groups were found to be compatible, yielding the desired amination products. Computational study shows that the amidation proceeds via an electrophilic aromatic substitution pathway, comprising a three-step process that includes substitution, addition, and elimination, which differs slightly from the classical mechanism.
Introduction
N-Fluorobenzenesulfonimide (NFSI)1–4 is a readily available, stable, and highly soluble crystalline powder that is widely utilized as a fluorinating reagent5–14 or oxidant in organic reactions.15–29 Additionally, NFSI has proven to be an efficient source of nitrogen for the C–H bond amidation of aromatic, heteroaromatic, benzyl, allyl, and aldehyde groups.30–36 In the amidation reactions, NFSI acts as a source of nitrogen radicals37 or as a nucleophilic nitrogen in various metal-catalyzed transformations of aromatic and aliphatic compounds.38–67 In this context, we describe a different type of amidation reaction in which NFSI serves as a source of electrophilic nitrogen, while the arene functions as a nucleophile.
Several research groups have reported the regioselective amidation reactions of indoles, pyrroles, quinolones, and imidazoheterocycles, which were mediated solely by a base or the hypervalent iodine reagents under mild conditions without using any metallic catalyst or oxidant.68–71 These groups hypothesized that the amidation reaction likely proceeds via a free radical mechanism (Scheme 1a).70 Additionally, it has been documented that the fluorinating reagent NFSI can be added to C60, yielding adduct 5 with over 90% efficiency on a large scale, again without the necessity for a metal catalyst or base. A concerted (2 + 2) cycloaddition mechanism has been proposed for the formation of this adduct (Scheme 1b).72
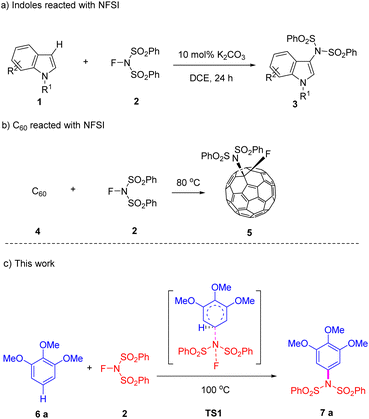 |
| Scheme 1 Reactions of arenes with NFSI without the need for catalysts. | |
Following the established literature procedures for metal-free amidation of heterocycles, we initially treated electron-rich arenes with NFSI. We were pleased to find that the mixture of 1,2,3-trimethoxybenzene (TMB) and NFSI could efficiently yield product 7a under elevated temperature conditions, without the need for any catalyst or additive (Scheme 1c). Furthermore, through density functional theory (DFT) calculations, we proposed a three-step mechanism characterized by the formation of adduct intermediates, which differs slightly from the classic electrophilic aromatic substitution mechanism.73
Our investigations started with the reaction between TMB and NFSI in various solvents at their respective refluxing temperatures. Unfortunately, only a poor yield of the desired product was isolated in dichloromethane after an extended reaction time. Even worse, only traces of product 7a were detected when the reaction was conducted in diethyl ether, which has a lower boiling point; a significant amount of starting material remained in the reaction mixture (Table 1, entries 1 and 2). Using high boiling point solvents, moderate yields of the desired product have been obtained (entries 3 and 4). Through detailed investigation, we believed that the reaction temperature plays a crucial role in the progression of the reaction. A nearly quantitative yield of the desired product was obtained when the reaction mixture was refluxed in DCE (entry 7). Conversely, minimal amounts of the desired product were produced when the reaction temperature was lowered down to 40 °C, with a substantial quantity of starting materials remaining (entry 5). The quantity of NFSI has also been assessed. Notably, some TMB remained when an equivalent amount of NFSI was used in this reaction, and a significantly larger excess of NFSI did not enhance the yield (entries 8 and 9). Notably, an excellent yield of the desired product was also achieved when the reaction mixture was stirred at its melting temperature without any solvent (entry 10). Consequently, we began to explore the role of solvents in this reaction, discovering that it could be effectively performed in various solvents, particularly in water (entries 11 and 12).
Table 1 Conditions optimizationa
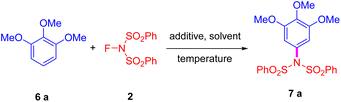
|
Entry |
Solvent |
Additive |
Temp [°C] |
Yieldb [%] |
Reaction condition: 6a (1.0 mmol), 2 (2.0 mmol), additives (1.0 mmol) in solvent (5 mL) under argon. Isolated yield of 7a. NFSI was used in 1.0 equivalent. NFSI was used in 3.0 equivalent. |
1 |
Et2O |
— |
35 |
<5 |
2 |
CH2Cl2 |
— |
40 |
<10 |
3 |
CCl4 |
— |
75 |
79 |
4 |
Toluene |
— |
110 |
62 |
5 |
DCE |
— |
40 |
<10 |
6 |
DCE |
— |
60 |
18 |
7 |
DCE |
— |
80 |
>95 |
8 |
DCE |
— |
80 |
68c |
9 |
DCE |
— |
80 |
92d |
10 |
Neat |
— |
110 |
91 |
11 |
MeOH |
— |
65 |
<5 |
12 |
H2O |
— |
100 |
31 |
13 |
DCE |
TEMPO |
80 |
68 |
14 |
Neat |
TEMPO |
110 |
64 |
Inspired by these results, we hypothesized that the formation of the desired product resembles an electrophilic aromatic substitution process rather than a radical mechanism,74–77 despite NFSI generally undergoing homolysis at elevated temperatures.78–81 To test our hypothesis, the radical scavenger TEMPO was selected.82 Upon the addition of an equivalent of TEMPO, we observed no significant change in the reaction outcome, with only a slight decrease in yield compared to the original reaction, regardless of whether the reaction was conducted in a solvent or in neat conditions (Table 1, entries 13 and 14).
To demonstrate the generality and efficiency of this method, a diverse array of electron-rich arenes was screened (Scheme 2). Benzenes bearing two or more alkyloxy groups proved to be suitable substrates for this transformation, yielding the desired products in good to excellent yields (7a–m). Notably, these amidation reactions proceeded smoothly in both DEC and neat conditions, regioselectively furnishing the final product as a single isomer. Moreover, the presence of phenolic hydroxyl (7b) and carbonyl groups (7h–l) was fully tolerated in this transformation, particularly the aldehyde substituent, which is an exceptionally reactive functional group in substitution reactions (7i and 7j). Other substituted benzenes were also examined; however, no reaction occurred with anisole or trimethylbenzene, and a complex mixture was obtained with aniline or naphthylamine. Interestingly, the reaction of 1,2,3-trimethoxy-5-methylbenzene with NFSI yielded a different type of amidated product (7n) as the major product. Conversely, 1,3,5-trimethoxybenzene (7o) and 2-methoxy naphthalene (7p) predominantly produced fluorinated compounds, with no amidation products detected.
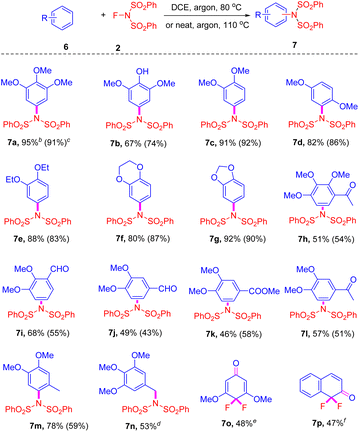 |
| Scheme 2 Scope of reaction between NFSI and electron-rich arenes. a Reaction conditions: 6 (1.0 mmol) and 2 (2.0 mmol) in DCE (5 mL) or in neat under argon atmosphere. b Isolated yields in DCE. c Isolated yields in neat. d Using 1,2,3-trimethoxy-5-methylbenzene as substrate. e Using 1,3,5-trimethoxybenzene as substrate. f Using 2-methoxy naphthalene as substrate. | |
Electrophilic aromatic substitution is a hallmark reaction in synthetic organic chemistry, typically understood as a two-step mechanism involving the formation of a σ-complex followed by the elimination of a small molecule.83 Through a combination of computational and experimental investigations, we propose an alternative three-step mechanism characterized by substitution, addition, and elimination (Scheme 3).
 |
| Scheme 3 Proposed mechanism for the amidation of TMB. | |
Geometry optimizations and frequency calculations of all presented structures were performed at the wB97XD/6-311G** level of theory,84 and the complete mechanism is illustrated in Fig. 1. TMB initially attacks the nitrogen atom of NFSI, resulting in the σ-complex (Int1) via TS1. This step presents an energy barrier of 29.7 kcal mol−1, which can be regarded as the rate-determining step. Notably, the existence of TS1 was further corroborated by Intrinsic Reaction Coordinate (IRC) calculations, confirming that the first-order saddle points identified represent genuine transition states connecting the starting materials and the σ-complex.
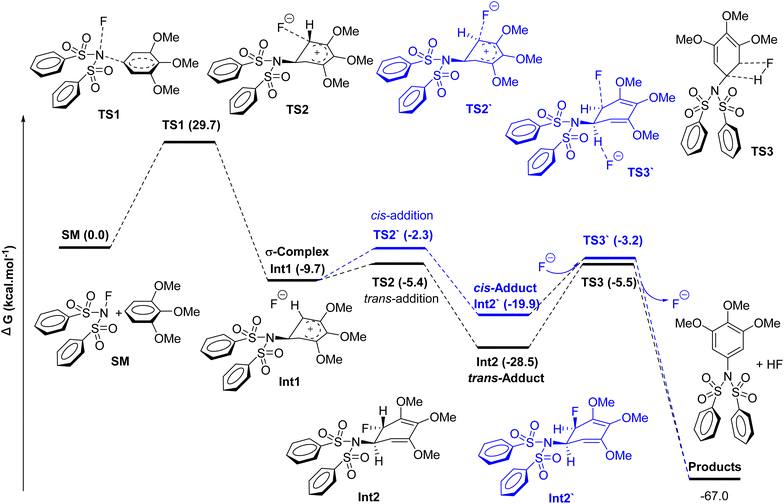 |
| Fig. 1 Free energy profile for the amidation reaction at the wb97xd/6-311++ level of theory. | |
In light of the addition product of NFSI with C60, we propose that the resulting σ-complex does not undergo an elimination reaction directly but rather proceeds through an addition reaction.72,85 Furthermore, extensive calculations indicate that this process occurs as a competition between cis- and trans-addition pathways. The fluorine anion of the σ-complex preferentially adds from the side opposite the nitrogen atom, resulting in the formation of the trans-adduct (Int2). This pathway requires overcoming a modest energy barrier of 4.3 kcal mol−1. Conversely, the fluorine anion may also engage in a cis-addition reaction, yielding the cis-adduct. However, the energy barrier for the cis-addition (Int2′) is 7.4 kcal mol−1 higher than that for the trans-addition. Thus, based on these findings, we can conclude that the reaction proceeds through the kinetically favored trans-adduction pathway that leads to the corresponding trans-adduct.
The loss of aromaticity in both adducts leads to their immediate elimination. In the case of the trans-adduct, fluorine departs in conjunction with the adjacent hydrogen, resulting in the formation of the desired product, 7a, which requires an energy input of 23.0 kcal mol−1. Similarly, the cis-adduct undergoes elimination facilitated by another fluorine anion, yielding the same product.
Conclusions
In summary, we have developed a practical transition metal-free, regioselective amidation of electron-rich arenes using NFSI as the commercially available amino source. This procedure can be conducted in both solvents and neat conditions, and it does not require any external oxidants or additives. The amidation process is compatible with a wide range of functional groups, including hydroxy, alkoxy, and carbonyl groups. DFT calculations support a three-step electrophilic aromatic substitution mechanism for this amidation reaction, wherein the σ-complex is formed first, followed by the production of two adduct isomers, and ultimately yielding the desired product through an elimination reaction. The formation of the σ-complex has been identified as the rate-determining step.
Data availability
The authors confirm that the data supporting this article have been included as part of the ESI.†
Conflicts of interest
There are no conflicts to declare.
Acknowledgements
We appreciate the financial support from the Natural Science Foundation of Chongqing City of China (CSTB2022NSCQ-MSX0847). The computing work in this paper was partly supported by the Supercomputing Center of Chongqing Medical University.
Notes and references
- W. C. Weilin Wang, J. Luo and P. Xie, Chin. J. Org. Chem., 2021, 41, 543–552 CrossRef.
- Q. Gu and E. Vessally, RSC Adv., 2020, 10, 16756–16768 RSC.
- S. Sushmita, T. Aggarwal, S. Kumar and A. K. Verma, Org. Biomol. Chem., 2020, 18, 7056–7073 RSC.
- Y. Li and Q. Zhang, Synthesis, 2015, 47, 159–174 CAS.
- Z.-M. Yan, L. Qi, T.-Y. Cao, J.-L. Liu, H.-J. Du, Y.-C. Dong, W. Li and L.-J. Wang, Org. Lett., 2023, 25, 3910–3915 CrossRef CAS PubMed.
- Y. Ito, A. Adachi, K. Aikawa, K. Nozaki and T. Okazoe, Chem. Commun., 2023, 59, 9195–9198 RSC.
- O. M. Shavrina, P. P. Onys'ko and Y. V. Rassukana, J. Fluorine Chem., 2022, 261–262, 110027 CrossRef CAS.
- L. Jin, X. Zeng, S. Li, G. Qiu and P. Liu, Eur. J. Org Chem., 2022, 2022, e202200399 CrossRef CAS.
- J. G. Hernández, K. J. Ardila-Fierro, D. Barišić and H. Geneste, Beilstein J. Org. Chem., 2022, 18, 182–189 CrossRef PubMed.
- S. Ogasawara, K. Nakano and H. Tamiaki, Tetrahedron, 2020, 76, 131722 CrossRef CAS.
- C. Shi, Q. Miao, L. Ma, T. Lu, D. Yang, J. Chen and Z. Li, ChemistrySelect, 2019, 4, 6043–6047 CrossRef CAS.
- F. Sakurai, T. Yukawa and T. Taniguchi, Org. Lett., 2019, 21, 7254–7257 CrossRef CAS PubMed.
- V. Levchenko, Y. V. Dmytriv, A. V. Tymtsunik, K. Liubchak, A. Rudnichenko, A. V. Melnyk, S. Y. Veselovych, Y. V. Borodulin, O. M. Otsalyuk, A. A. Tolmachev and P. K. Mykhailiuk, J. Org. Chem., 2018, 83, 3265–3274 CrossRef CAS PubMed.
- M. Meanwell, M. B. Nodwell, R. E. Martin and R. Britton, Angew. Chem., Int. Ed., 2016, 55, 13244–13248 CrossRef CAS PubMed.
- F. Bao, Asian J. Org. Chem., 2023, 12, e202300407 CrossRef CAS.
- X. Zhang, Y. Feng, Y. Tuo and Q.-Z. Zheng, Org. Biomol. Chem., 2022, 20, 768–772 RSC.
- Y.-Q. Jiang, Y.-H. Wang, C.-F. Zhou, Y.-Q. Zhang, Y. Ling, Y. Zhao and G.-Q. Liu, J. Org. Chem., 2022, 87, 14609–14622 CrossRef CAS PubMed.
- B. Božić, J. Lađarević, M. Petković, D. Mijin and S. Stavber, Catalysts, 2022, 12, 1413 CrossRef.
- X. Xu, L. Yan, S. Wang, P. Wang, A. X. Yang, X. Li, H. Lu and Z.-Y. Cao, Org. Biomol. Chem., 2021, 19, 8691–8695 RSC.
- L.-Q. Liu, J.-L. Li, Y.-C. Wang and H.-S. Wang, Results Chem., 2021, 3, 100220 CrossRef CAS.
- S. Liu, Y. Huang, X.-H. Xu and F.-L. Qing, J. Fluorine Chem., 2020, 240, 109653 CrossRef CAS.
- G. Liang, Y. Ji, H. Liu, Y. Pang, B. Zhou, M. Cheng, Y. Liu, B. Lin and Y. Liu, Adv. Synth. Catal., 2020, 362, 192–205 CrossRef CAS.
- Q. Lin, Y. Liu, Z. Xiao, L. Zheng, X. Zhou, Y. Guo, Q.-Y. Chen, C. Zheng and C. Liu, Org. Chem. Front., 2019, 6, 447–450 RSC.
- J. H. Jing Lia, G. Meib, W. Xiaohuaa, W. Qiantaoa and W. Yonga, Chin. J. Org. Chem., 2018, 38, 692–697 CrossRef.
- Y. Liu, H. Wu, Y. Guo, J.-C. Xiao, Q.-Y. Chen and C. Liu, Angew. Chem., Int. Ed., 2017, 56, 15432–15435 CrossRef CAS PubMed.
- M. Song, Q. Hu, Z.-Y. Li, X. Sun and K. Yang, Chin. Chem. Lett., 2022, 33, 4269–4272 CrossRef CAS.
- L. Tang, F. Yang, H. Cheng, C. Tan, C. Jin, H. Chen, Y. Huang, S. Zhang, W. Song and J. Tan, Org. Lett., 2020, 22, 8618–8623 CrossRef CAS PubMed.
- Y. Lv, K. Sun, W. Pu, S. Mao, G. Li, J. Niu, Q. Chen and T. Wang, RSC Adv., 2016, 6, 93486–93490 RSC.
- B. He, Z. Xiao, H. Wu, Y. Guo, Q.-Y. Chen and C. Liu, RSC Adv., 2017, 7, 880–883 RSC.
- X. Yi, S. Lei, W. Liu, F. Che, C. Yu, X. Liu, Z. Wang, X. Zhou and Y. Zhang, Org. Lett., 2020, 22, 4583–4587 CrossRef CAS PubMed.
- J. A. Buss, A. Vasilopoulos, D. L. Golden and S. S. Stahl, Org. Lett., 2020, 22, 5749–5752 CrossRef CAS PubMed.
- S. Sushmita, T. Aggarwal, N. Shibata and A. K. Verma, Chem.–Eur. J., 2019, 25, 16063–16067 CrossRef.
- S. Han, X. Gao, Q. Wu, J. Li, D. Zou, Y. Wu and Y. Wu, Org. Chem. Front., 2019, 6, 830–834 RSC.
- R.-J. Tang, C.-P. Luo, L. Yang and C.-J. Li, Adv. Synth. Catal., 2013, 355, 869–873 CrossRef CAS.
- K. Kaneko, T. Yoshino, S. Matsunaga and M. Kanai, Org. Lett., 2013, 15, 2502–2505 CrossRef CAS PubMed.
- P. A. Sibbald and F. E. Michael, Org. Lett., 2009, 11, 1147–1149 CrossRef CAS PubMed.
- B. E. Haines, T. Kawakami, K. Kuwata, K. Murakami, K. Itami and D. G. Musaev, Chem. Sci., 2017, 8, 988–1001 RSC.
- W. Wang, L. Zhao, H. Wu, Y. He and G. Wu, Org. Lett., 2023, 25, 7078–7082 CrossRef CAS PubMed.
- K. Zhou, L. Yin, Y. Guo, C.-H. Ding and B. Xu, Synthesis, 2022, 55, 744–754 Search PubMed.
- A. Zhou, Y. Shao, F. Chen, P.-C. Qian and J. Cheng, Tetrahedron Lett., 2022, 89, 153597 CrossRef CAS.
- S. Yang, C. Liu, X. Shangguan, Y. Li and Q. Zhang, Chem. Sci., 2022, 13, 13117–13121 RSC.
- D. Hao, Z. Yang, Y. Liu, Y. Li, Y. Liu and P. Liu, J. Mol. Struct., 2022, 1267, 133636 CrossRef CAS.
- S.-C. Wang, M.-N. Feng, Y. Ji, W.-W. Han, C.-Y. Ke, Q.-Z. Zhang and X.-L. Zhang, RSC Adv., 2021, 11, 12136–12140 RSC.
- Z. Shao, F. Wang, J. Shi, L. Ma and Z. Li, Org. Chem. Front., 2021, 8, 3298–3307 RSC.
- T. W. Pouambeka, G. C. Enoua, F. C. Bopoundza, H. Makomo, B. W. Loumouamou and Q. Zhan, Adv. J. Chem., Sect. B, 2021, 3, 176–187 CAS.
- Z. Li, L. Ye, Y. Cao, J. Qin, W. Wang and Y. Xie, Tetrahedron Lett., 2021, 83, 153427 CrossRef CAS.
- B. Lei, Q. Miao, L. Ma, R. Fu, F. Hu, N. Ni and Z. Li, Org. Biomol. Chem., 2019, 17, 2126–2133 RSC.
- W.-B. Cao, X.-P. Xu and S.-J. Ji, Adv. Synth. Catal., 2019, 361, 1771–1776 CrossRef CAS.
- F. Bao, Y. Cao, W. Liu and J. Zhu, RSC Adv., 2019, 9, 27892–27895 RSC.
- Y.-c. Wang, L.-q. Liu, G.-m. Wang, H. Ouyang and Y.-j. Li, Green Chem., 2018, 20, 604–608 RSC.
- S. Samanta and A. Hajra, J. Org. Chem., 2018, 83, 13157–13165 CrossRef CAS PubMed.
- C. R. Reddy, S. K. Prajapti and R. Ranjan, Org. Lett., 2018, 20, 3128–3131 CrossRef CAS PubMed.
- W. Yuan and K. J. Szabó, ACS Catal., 2016, 6, 6687–6691 CrossRef CAS.
- D. Yang, M. Sun, W. Wei, J. Li, P. Sun, Q. Zhang, L. Tian and H. Wang, RSC Adv., 2016, 6, 72361–72365 RSC.
- X.-F. Xia, S.-L. Zhu, J.-B. Liu, D. Wang and Y.-M. Liang, J. Org. Chem., 2016, 81, 12482–12488 CrossRef CAS PubMed.
- C. Herrera-Leyton, M. Madrid-Rojas, J. J. López, Á. Cañete, P. Hermosilla-Ibáñez and E. G. Pérez, ChemCatChem, 2016, 8, 2015–2018 CrossRef CAS.
- Z. Ni, Q. Zhang, T. Xiong, Y. Zheng, Y. Li, H. Zhang, J. Zhang and Q. Liu, Angew. Chem., Int. Ed., 2012, 51, 1244–1247 CrossRef CAS PubMed.
- T. Xiong, Y. Li, Y. Lv and Q. Zhang, Chem. Commun., 2010, 46, 6831–6833 RSC.
- P. A. Sibbald, C. F. Rosewall, R. D. Swartz and F. E. Michael, J. Am. Chem. Soc., 2009, 131, 15945–15951 CrossRef CAS PubMed.
- Y. Yin, J. Xie, F.-Q. Huang, L.-W. Qi and B. Zhang, Adv. Synth. Catal., 2017, 359, 1037–1042 CrossRef CAS.
- S. Lu, L.-L. Tian, T.-W. Cui, Y.-S. Zhu, X. Zhu, X.-Q. Hao and M.-P. Song, J. Org. Chem., 2018, 83, 13991–14000 CrossRef CAS PubMed.
- X. Wang, B. Lei, L. Ma, H. Jiao, W. Xing, J. Chen and Z. Li, Adv. Synth. Catal., 2017, 359, 4284–4288 CrossRef CAS.
- S. Wang, Z. Ni, X. Huang, J. Wang and Y. Pan, Org. Lett., 2014, 16, 5648–5651 CrossRef CAS PubMed.
- K. Sun, Y. Li, T. Xiong, J. Zhang and Q. Zhang, J. Am. Chem. Soc., 2011, 133, 1694–1697 CrossRef CAS PubMed.
- W.-M. Gao, L. Hu, F. Gao, G. Hu and X. Zhou, J. Chem. Res., 2024, 48, 17475198231226425 CrossRef CAS.
- X. Jia, X. Tian, D. Zhuang, Z. Wan, J. Gu and Z. Li, Org. Lett., 2023, 25, 2012–2017 CrossRef CAS PubMed.
- G. B. Boursalian, M.-Y. Ngai, K. N. Hojczyk and T. Ritter, J. Am. Chem. Soc., 2013, 135, 13278–13281 CrossRef CAS PubMed.
- M. Singsardar, S. Mondal, R. Sarkar and A. Hajra, ACS Omega, 2018, 3, 12505–12512 CrossRef CAS PubMed.
- Y. Wang, Y. Wang, Z. Guo, Q. Zhang and D. Li, Asian J. Org. Chem., 2016, 5, 1438–1441 CrossRef CAS.
- H.-H. Liu, Y. Wang, G. Deng and L. Yang, Adv. Synth. Catal., 2013, 355, 3369–3374 CrossRef CAS.
- D. Xiang, L. Xia, Y. Zhang, Q. Zhang and D. Li, Synlett, 2018, 29, 1400–1404 CrossRef CAS.
- Y. Li, N. Lou and L. Gan, Org. Lett., 2015, 17, 524–527 CrossRef CAS PubMed.
- R. Van Lommel, P. Geerlings, T. Stuyver, S. L. C. Moors and F. De Proft, in Chemical Reactivity, ed. S. Kaya, L. von Szentpály, G. Serdaroğlu and L. Guo, Elsevier, 2023, pp. 243–275 Search PubMed.
- N. Stamenković, N. P. Ulrih and J. Cerkovnik, Phys. Chem. Chem. Phys., 2021, 23, 5051–5068 RSC.
- M. Mandal, J. A. Buss, S.-J. Chen, C. J. Cramer and S. S. Stahl, Chem. Sci., 2024, 15, 1364–1373 RSC.
- Y. Yang, Y. Yu, Y. Wang, Q. Zhang and D. Li, Tetrahedron, 2018, 74, 1085–1091 CrossRef CAS.
- T. Qin, G. Lv, Q. Meng, G. Zhang, T. Xiong and Q. Zhang, Angew. Chem., Int. Ed., 2021, 60, 25949–25957 CrossRef CAS PubMed.
- L. He, Y. Zhu and Y. Xu, ChemistrySelect, 2021, 6, 13559–13563 CrossRef CAS.
- P. Ghosh and A. Hajra, J. Org. Chem., 2021, 86, 10883–10888 CrossRef CAS PubMed.
- M. Iwasaki, K. Nonaka, S. Zou, Y. Sawanaka, T. Shinozaki, T. Fujii, K. Nakajima and Y. Nishihara, J. Org. Chem., 2019, 84, 15373–15379 CrossRef CAS PubMed.
- C. Li, S. Fan, Z. Wang and Z. Song, Asian J. Org. Chem., 2024, 13, e202300506 CrossRef CAS.
- Q. Miao, Z. Shao, C. Shi, L. Ma, F. Wang, R. Fu, H. Gao and Z. Li, Chem. Commun., 2019, 55, 7331–7334 RSC.
- T. Stuyver, D. Danovich, F. De Proft and S. Shaik, J. Am. Chem. Soc., 2019, 141, 9719–9730 CrossRef CAS PubMed.
- G. W. T. M. J. Frisch, H. B. Schlegel, G. E. Scuseria, M. A. Robb, J. R. Cheeseman, G. Scalmani, V. Barone, B. Mennucci, G. A. Petersson, H. Nakatsuji, M. Caricato, X. Li, H. P. Hratchian, A. F. Izmaylov, J. Bloino, G. Zheng, J. L. Sonnenberg, M. Hada, M. Ehara, K. Toyota, R. Fukuda, J. Hasegawa, M. Ishida, T. Nakajima, Y. Honda, O. Kitao, H. Nakai, T. Vreven, J. A. Montgomery Jr, J. E. Peralta, F. Ogliaro, M. Bearpark, J. J. Heyd, E. Brothers, K. N. Kudin, V. N. Staroverov, T. Keith, R. Kobayashi, J. Normand, K. Raghavachari, A. Rendell, J. C. Burant, S. S. Iyengar, J. Tomasi, M. Cossi, N. Rega, J. M. Millam, M. Klene, J. E. Knox, J. B. Cross, V. Bakken, C. Adamo, J. Jaramillo, R. Gomperts, R. E. Stratmann, O. Yazyev, A. J. Austin, R. Cammi, C. Pomelli, J. W. Ochterski, R. L. Martin, K. Morokuma, V. G. Zakrzewski, G. A. Voth, P. Salvador, J. J. Dannenberg, S. Dapprich, A. D. Daniels, O. Farkas, J. B. Foresman, J. V. Ortiz, J. Cioslowski and D. J. Fox, Gaussian 09, Gaussian, Inc., Wallingford, CT, 2013 Search PubMed.
- B. Galabov, D. Nalbantova, P. v. R. Schleyer and H. F. Schaefer III, Acc. Chem. Res., 2016, 49, 1191–1199 CrossRef CAS PubMed.
Footnotes |
† Electronic supplementary information (ESI) available. See DOI: https://doi.org/10.1039/d4ra07008a |
‡ Both authors contributed equally and should be considered co-first authors. |
|
This journal is © The Royal Society of Chemistry 2024 |
Click here to see how this site uses Cookies. View our privacy policy here.