DOI:
10.1039/D4RA07114J
(Review Article)
RSC Adv., 2024,
14, 38952-38995
Advancements in nanotechnology-driven photodynamic and photothermal therapies: mechanistic insights and synergistic approaches for cancer treatment
Received
3rd October 2024
, Accepted 1st December 2024
First published on 10th December 2024
Abstract
Cancer is a disease that involves uncontrolled cell division triggered by genetic damage to the genes that control cell growth and division. Cancer starts as a localized illness, but subsequently spreads to other areas in the human body (metastasis), making it incurable. Cancer is the second most prevalent cause of mortality worldwide. Every year, almost ten million individuals get diagnosed with cancer. Although different cancer treatment options exist, such as chemotherapy, radiation, surgery and immunotherapy, their clinical efficacy is limited due to their significant side effects. New cancer treatment options, such as phototherapy, which employs light for the treatment of cancer, have sparked a growing fascination in the cancer research community. Phototherapies are classified into two types: photodynamic treatment (PDT) and photothermal therapy (PTT). PDT necessitates the use of a photosensitizing chemical and exposure to light at a certain wavelength. Photodynamic treatment (PDT) is primarily based on the creation of singlet oxygen by the stimulation of a photosensitizer, which is then used to kill tumor cells. PDT can be used to treat a variety of malignancies. On the other hand, PTT employs a photothermal molecule that activates and destroys cancer cells at the longer wavelengths of light, making it less energetic and hence less hazardous to other cells and tissues. While PTT is a better alternative to standard cancer therapy, in some irradiation circumstances, it can cause cellular necrosis, which results in pro-inflammatory reactions that can be harmful to therapeutic effectiveness. Latest research has revealed that PTT may be adjusted to produce apoptosis instead of necrosis, which is attractive since apoptosis reduces the inflammatory response.
1. Introduction
Therapy with light has been practiced for the past three millennia.1 Light was employed by the ancient Egyptian, Indian, and Chinese cultures to treat a variety of ailments, such as vitiligo, rickets, psoriasis, and skin cancer. Numerous therapies exist that take use of the photochemical, photothermal, or photomechanical interactions between light and tissues in the body.2 Thermal therapies have been used to treat cancer cells since the 18th century. A class of disorders known as cancer is defined by abnormal and uncontrolled cell proliferation that can have detrimental effects on health. Depending on the kind and severity of the cancer, many treatment options are available. For example, surgery aids in the removal of cancerous masses or tumours. Drugs are used in chemotherapy to destroy certain cancer cells. Other cancer treatments include immune-therapy, bone marrow transplantation, targeted medication therapy, radiation therapy, hormone therapy, and cryoablation. While there have been some instances when these therapies have been shown to be beneficial, they also have serious negative effects. Finding the most effective cancer medication that has higher efficacy and few to nil side effects is therefore urgently needed.
The raising of temperature over physiological ranges, usually to 40–45 °C, is known as hyperthermia. The major objective of hyperthermia is to provide an environment that makes tumor removal easier while protecting healthy tissues used in cancer therapy. However, the normal tissues are frequently impacted by this treatment. Applying photothermal therapy with laser radiation can make photothermal therapy more applicable for more targeted cancer treatment. The requirement for a powerful laser to kill the tumor cells is a major drawback of this treatment. Modern phototherapy offers advantages including focused therapy, deep penetration, specialized phototherapy, large exposure area, and longer exposure period. It also makes use of nanomaterials like CNT, gold nanoparticles, graphene, and quantum dots. The combination of PDT and PTT is also quickly gaining popularity due to its synergistic benefits, which can help chemotherapy and radiation therapy as an adjuvant treatment method Fig. 1.3
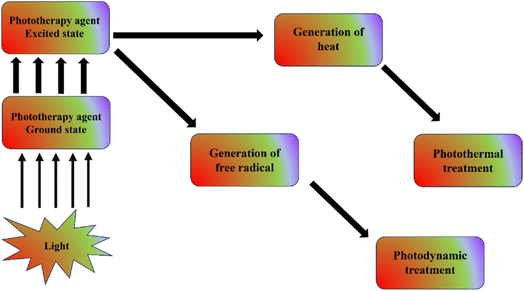 |
| Fig. 1 Simple diagrammatic representation of photodynamic therapy and photothermal therapy mechanism. | |
Photodynamic therapy (PDT) was developed in the nineteenth century by Niels Finsen. The scientists found that mixing chemicals and light may cause more cell death than usual. Using light-activatable chemicals (or, more recently, nanoparticles) to trigger the production of deadly harmful free radicals or reactive oxygen species—most frequently, singlet-state oxygen, or 1O2—photodynamic treatment (PDT) is based on photochemistry.4 The main component of photodynamic treatment (PDT) is the use of photosensitizers (PSs), which are activated by light of a wavelength that is long enough to convert oxygen molecules into ROS (hazardous reactive oxygen species), such as singlet oxygen. This process ultimately results in cell death for the cancer cells by oxidative stress.5
In contrast, photothermal therapy (PTT) kills cells and tissues primarily by heat using just light, often in the near-infrared range for maximal tissue penetration.6 PTT is a more discreet and maybe helpful substitute for treating cancer. Applying pulsed laser irradiation within the near-infrared (NIR) range to activate photosensitizing compounds, PTT produces heat for the thermal destruction of cancer tumours with a restricted invasion into neighbouring healthy tissues.7 Selective heating has been suggested for PTT that contains a photothermal agent. The main requirements for PTT are an NIR light source and a compatible photothermal compound with an exceptionally high absorption co-efficient. The degree of absorption of the NIR spectrum and the light-excitation coefficient determine the temperature increase in the PTT. Photothermal therapy may eradicate cancer cells from the main tumor or from tumors that have already begun to metastasize, either by alone or in combination.3
Cancer is a primary source of illness and mortality globally, necessitating ongoing efforts to innovate and improve treatment options. Traditional cancer treatments, such as chemotherapy, radiation, and surgery, are restricted due to systemic side effects, non-specificity, and, in many cases, treatment resistance. In response, photodynamic therapy (PDT) and photothermal therapy (PTT) have emerged as potential minimally invasive cancer therapies due to their ability to precisely target tumor cells while causing minimum harm to surrounding healthy tissue. PDT uses light-activated photosensitizers to generate reactive oxygen species (ROS), which cause cancer cell death, whereas PTT uses photothermal agents to turn light into heat, resulting in localized hyperthermia and cell ablation. Despite their therapeutic promise, PDT and PTT are restricted by tissue penetration, nonspecific accumulation, and sometimes poor therapeutic effectiveness. These problems have sparked interest in nanotechnology as a way to improve the efficacy and specificity of PDT and PTT.3,8–10
Nanomaterials have distinct physicochemical features, such as variable size, surface charge, large surface area, and increased optical properties, making them excellent for improving PDT and PTT. Nanomaterials, such as gold, silver, silica, and silicon nanoparticles, quantum dots, carbon-based nanomaterials, manganese dioxide (MnO2) nanosheets, and nanoscale metal–organic frameworks (MOFs), have been engineered to enhance the stability, cellular uptake, and therapeutic impact of photosensitizers and photothermal agents. For example, gold nanoparticles have a significant surface plasmon resonance, which improves light absorption and heat production in PTT. Furthermore, their biocompatibility and ease of functionalization make them useful carriers for photosensitizers, facilitating targeted administration and effective ROS generation in PDT. However, the exorbitant cost of gold nanoparticles, as well as their potential cytotoxicity at high concentrations, limit their use, spurring the search for alternatives.11–13
NPs have been discovered to penetrate deep tissues, increasing the increased permeability and retention (EPR) impact. Furthermore, surface features influence bioavailability and half-life by successfully passing through epithelial fenestration.14 For example, NPs coated with polyethylene glycol (PEG), a hydrophilic polymer, reduce opsonization and avoid immune system clearance.15,16 It is also feasible to optimise the release rate of medications or active moiety by altering particle polymer properties. Overall, the unique features of NPs influence their therapeutic efficacy in cancer care and therapy.
Drug-targeting pathways are techniques for delivering therapeutic compounds directly to disease locations, boosting therapy efficacy while avoiding unwanted effects. These pathways are classified as passive and active targeting. Passive targeting is based on the spontaneous accumulation of medications in certain tissues due to biological processes such as the Enhanced Permeability and Retention (EPR) effect, which is especially important in malignancies. The EPR effect arises because tumor tissues have leaky vasculature with big holes that allow nanoparticles and drug carriers to collect, and tumors have inadequate lymphatic drainage, which helps to keep these medications for longer. This method is frequently used in cancer therapies, where drug-loaded nanoparticles passively aggregate in tumor tissues without the need for particular targeting mechanisms. Passive targeting simplifies drug carrier design since it does not require specialized ligands to target receptors; nevertheless, its shortcoming is a lack of specificity, as it relies on physiological parameters rather than precision targeting.17–19
Active targeting, on the other hand, entails modifying drug carriers with particular molecules such as antibodies, peptides, or ligands that bind preferentially to receptors on target cells' surfaces. By using ligands that bind to receptors overexpressed on sick cells, such as cancer cells, active targeting guarantees that the drug carrier is delivered specifically to the designated region. This method is particularly effective in targeted cancer therapy, as certain cells overexpress identified receptors, allowing for more accurate drug administration. Active targeting has higher specificity and fewer off-target effects, making it highly effective for diseases with distinct cellular markers. However, it requires detailed knowledge of target cell receptors and careful engineering of drug carriers, which can be complex and costly. Both passive and active targeting are critical in developing drug delivery systems, particularly in nanomedicine, since they allow for increased drug accumulation at disease locations and better therapeutic effects.20,21
Nanoparticles play an important role in both passive and active drug-targeting techniques because they have unique features that improve drug delivery accuracy and efficiency in modern biomedical research. Nanoparticles used in passive targeting take advantage of tumors' Enhanced Permeability and Retention (EPR) effect. Nanoparticles, because to their tiny size and ability to be tailored to match ideal size and surface features, may easily pass through tumor vasculature and aggregate more efficiently than standard therapeutic molecules. This has been established in several studies employing nanoparticles such as liposomes, polymeric nanoparticles, and lipid-based nanocarriers to carry chemotherapeutic drugs to malignant cells. Researchers increased medication stability and bioavailability by encapsulating pharmaceuticals in nanoparticles, resulting in more concentrated and extended drug release at the target location.
Nanoparticles are an appropriate platform for active targeting because they may be functionalized with particular ligands, antibodies, or peptides that bind selectively to cell surface receptors on sick cells, such as cancer cells overexpressing certain biomarkers. Gold nanoparticles, quantum dots, and polymeric nanoparticles, for example, have been functionalized with targeting molecules such as folic acid, transferrin, or antibodies to specifically target cancer cells with overexpressed receptors, resulting in more effective and selective delivery. Current research indicates that nanoparticles with these surface changes have the potential to improve drug delivery accuracy, reduce off-target effects, and allow for lower drug dosages, hence lowering possible toxicity to healthy tissues.22,23
Furthermore, nanoparticles provide extra functions in targeted treatment due to their inherent features. Certain metallic nanoparticles, such as gold or magnetic nanoparticles, can perform both drug delivery and imaging, enabling for real-time tracking and monitoring of medication distribution inside the body. Others, such as photothermal or photodynamic agents, are intended to release therapeutic chemicals in response to certain stimuli, such as light or heat, allowing for spatiotemporally regulated treatment at the target region. As a result, nanoparticles are crucial to current research on passive and active medication targeting, allowing for a new degree of personalization in treatment techniques that are highly precise, minimally intrusive, and tuned for optimal therapeutic efficacy in disease management.24–27
Nanoparticles have considerable benefits in cancer therapy, both as independent medicines and in conjunction with photodynamic therapy (PDT), because of their unique features that allow for targeted, regulated, and multi-functional therapeutic methods. As individual agents, nanoparticles improve drug delivery by allowing both passive and active targeting, resulting in increased drug accumulation in tumors while sparing healthy cells, thereby boosting therapeutic efficacy and lowering adverse effects. Additionally, nanoparticles improve therapeutic solubility and stability, particularly for hydrophobic medicines that are difficult to administer efficiently in traditional forms. They also allow for regulated and prolonged medication release at the tumor site, which reduces the need for frequent dosing and increases patient compliance. Some nanoparticles, such as gold and magnetic nanoparticles, have intrinsic therapeutic capabilities, such as causing localized heating (hyperthermia) in response to specific stimuli, which can either directly kill cancer cells or sensitize them to subsequent therapies. Furthermore, nanoparticles have theranostic capabilities, which means they may perform both therapeutic and diagnostic tasks. For example, iron oxide nanoparticles can act as MRI contrast agents while also providing heat, allowing for real-time monitoring of the therapy's progression.28–32
Silver nanoparticles have also demonstrated potential owing to their antibacterial characteristics and significant light absorption in the near infrared (NIR) region, making them suited for both PDT and PTT. However, worries about their long-term biocompatibility and potential toxicity have yielded inconsistent outcomes in clinical trials. Silica and silicon nanoparticles, on the other hand, offer a biocompatible and cost-effective platform with great thermal stability and drug encapsulation capability, which improves the therapeutic index of PDT and PTT treatments. Quantum dots (QDs) have unique optical characteristics and great photostability, allowing for real-time imaging and monitoring of photosensitizers in vivo, enhancing PDT accuracy. However, the toxicity of heavy metals in QDs has limited their usage in clinical applications.33,34
Carbon-based nanomaterials, such as graphene and carbon nanotubes, have a high surface area and unique heat conductivity, making them ideal photothermal agents in PTT. These materials have high photothermal conversion efficiency, but they require careful surface modification to increase dispersibility and biocompatibility. Manganese dioxide (MnO2) nanosheets and nanoscale metal–organic frameworks (MOFs) have received interest for their flexibility in PDT and PTT. MnO2 nanosheets have catalytic activity that increases intracellular oxygen levels, reducing hypoxia in the tumor microenvironment, a recognized obstacle to PDT effectiveness. MOFs, with their high porosity and flexible frameworks, provide variable release profiles for photosensitizers and other therapeutic agents, hence improving both PDT and PTT results. However, there are still hurdles in synthesizing MOFs of uniform quality and stability under physiological circumstances.35
In addition to these individual nanoparticles, great progress has been made in mixing numerous nanomaterials to form hybrid systems with complimentary features. For example, incorporating gold nanoparticles into carbon-based materials can improve photothermal stability and efficacy, whereas silica-coated nanoparticles can minimize toxicity and increase circulation times. The combination of PDT and PTT with traditional treatments such as chemotherapy, radiation, and immunotherapy has shown further synergistic effects in cancer treatment, leveraging the strengths of each modality to improve overall therapeutic results. Such combination medicines address several areas of cancer pathophysiology, possibly overcoming challenges such as treatment resistance and immune evasion.36–38
When used with PDT, nanoparticles provide additional benefits that increase the therapy's efficacy. They increase photosensitizer transport to tumor locations by raising the concentration of the photosensitizer in cancer cells, improving PDT efficacy while decreasing undesired accumulation in healthy tissues. Encapsulating or attaching photosensitizers to nanoparticles shields them against degradation, increasing photostability and extending their active duration. Furthermore, certain nanoparticles allow for regulated activation via dual-modal treatments, in which PDT is paired with photothermal effects, therefore boosting the therapeutic effectiveness. Nanoparticles also increase reactive oxygen species (ROS) formation in PDT, enhancing the deadly effect on cancer cells. Combining PDT with other nanoparticle features, such as magnetic hyperthermia, allows for multi-modal therapy that can overcome tumor resistance and minimize recurrence. Finally, nanoparticles enable real-time imaging and monitoring, which is useful for tracking photosensitizer location and improving therapy progress. Overall, nanoparticles employed alone or in combination with PDT provide novel options in cancer therapy, providing focused, regulated, and synergistic techniques that may enhance patient outcomes by overcoming the limits of current therapies.39–41
The need for this study stems from the potential yet fragmented research environment in nanomaterial-enhanced PDT and PTT. While various studies have looked at individual nanomaterials for these therapies, there is an urgent need to consolidate and critically analyze the benefits, limits, and synergistic potential of these nanomaterials in the context of combined cancer treatments. This study seeks to bridge knowledge gaps and highlight future possibilities in cancer nanomedicine by giving a complete overview of PDT and PTT molecular processes, nanomaterial advances, and combination techniques. This topic was chosen because of its potential to enlighten and inspire focused, effective, and patient-centered cancer treatment tactics, resulting in the development of more resilient and individualized oncological medicines.
2. Mechanism of action
2.1. Mechanism of action at molecular level of PDT and PTT
Three primary constituents typically necessary for PDT: light, a light-activatable substance (called a photosensitizer, or PS), and, in the case of many PS, oxygen. These three ingredients combine to undergo a photodynamic reaction that results in the production of cytotoxic ROS. A PS molecule absorbs incident light, which changes it from its from ground state to excited singlet state with a short half-life (∼ns). This singlet state subsequently crosses the intersystem to become an excited triplet state, which is comparatively more stable (∼ms). Photosensitizer molecules can undergo a type I or type II photodynamic reaction to return from the triplet to the ground state. In the former, different ROS, such as O2˙− and
, are formed when activated photosensitizers transfer an electron to substrate. A type II reaction produces energy quickly shifted to ground-state molecular oxygen. Numerous biological reactions, such as direct cytotoxicity, vascular events and inflammation, are triggered by the reactive species. Significantly, the diffusion distance of ROS within cells and tissues is remarkably small (less than 50 nm), meaning the photosensitizers often required to be positioned in close range to the target location Fig. 2.42,43 PDT frequently produces a distinct border separating the necrotic sections that received the TPDT dosage and the viable tissue regions because of the shortened ROS distance of diffusion and targeted light delivery.44 Furthermore, as photosensitizers prefer to cluster inside cancer cells, PDT produces extremely minimal effects on adjacent neurons and ECM, although when they are in the treatment area.45 Because of this, PDT is appropriate for tumors that are encircled by vital tissues, such as malignancies of the bladder, oesophagus, lung, prostate, pancreatic, and head and neck.46
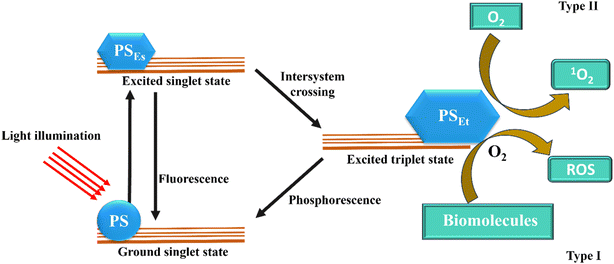 |
| Fig. 2 The PDT's mode of action displays both type I and type II responses. ROS denotes reactive oxygen species, whereas PSEs and PSEt denote PS excited singlet and triplet states (Calixto et al. 2016).42 | |
Using light, usually in the near-infrared (NIR) spectrum, photothermal treatment (PTT) raises tissue temperature and causes local photocoagulation. PTT uses comparatively high light intensities to reach temperatures that are either coagulative (55–100 °C) or subcoagulative (43–55 °C), which causes fast cell death by producing disruption to the cell membrane and denaturation of proteins.47 Milder heating protocols (41–43 °C) are categorized as hyperthermia, which, unless administered for extended periods of time (>1 h), is not tumoricidal on its own. Although hyperthermia is not utilized therapeutically as an independent modality, it may be used to induce HSP and change the tumor perfusion and metabolic status, which can improve the selectivity and effectiveness of other modalities like radiation treatment or chemotherapy.48
2.2. Mechanisms of cell death via PDT
Due to the short-lived ROS that primarily drives PDT-induced cytotoxicity, the prevailing cell death pathways are determined by the chemical structure of the photosensitizer, its subcellular location, and the parameters of light delivery. It is well acknowledged that lysosomal photosensitizers cause protease release, whereas photosensitizers that localize to the mitochondria and endoplasmic reticulum cause apoptosis by release of cytochrome C. It is interesting to note that PDT efficacy is greatly increased by successive targeting of lysosomes as well as mitochondria. This effect may be attributed to increased radical generation within mitochondria as a consequence of photochemically driven iron release by lysosomes.49 Utilizing this approach, a liposomal PS composition that targets lysosomes, endoplasmic reticulum and mitochondria all at once was created.50 Photo damage that degrades the Bcl-2 (an antiapoptotic protein), which triggers a series of downstream pro-apoptotic events, is another mechanism of PDT cytotoxicity. PDT can also induce paraptosis and autophagy along with necrosis and apoptosis.49,51 Last but not least, some photosensitizers have the capacity to elicit immunogenic cell death (ICD), as demonstrated by the externalizing of calreticulin (CRT) as well as the ATP, HMGB1, HSP70 and HSP90 are therefore released.52
2.3. Mechanisms of cell death via PTT
Depending on the temperature, time frame, and the usage of external photo-absorbers like nanoparticles, PTT can work through diverse cell death pathways Fig. 3.53 The main mechanism of PTT cytotoxicity is protein denaturation via induction of heat, so the higher the temperature, the more rapidly and efficiently the death of cell occurs. Mild hyperthermia (∼41–43 °C) has been shown to induce HSP response, however, it is less damaging if continued over an extended length of time (∼1 h).54,55 It is believed that apoptosis is induced by PTT (55–60 °C), which will be followed with secondary necrosis.47 The study showed that melanoma cells exposed with gold nanorods exhibited patterns of temperature-dependent cell death. A comparatively minor amount of cell viability was lost with mild heating (43 °C), with 10.2% through apoptosis, 18.3% by necroptosis, and 17.6% of cells via necrosis, respectively. Raising the temperature over the coagulative threshold (about 55–60 °C) usually results in tissue necrosis right away because of proteins denaturation and a breakdown of integrity of cell membrane.56 The real-time visualization of these tissue changes by MRI, ultrasound, or optical modalities is beneficial for therapy adjustment and monitoring.57
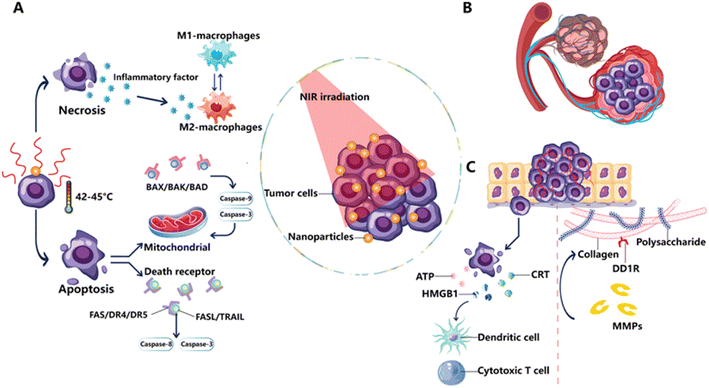 |
| Fig. 3 PTT mechanics as perceived via a medical viewpoint. (A) Tumor cell necrosis and apoptosis: PTT induces necrosis by breaking the tumor cell membrane, which causes the release of inflammatory cytokines and encouraging macrophages to adopt an M2-like phenotype. When a cell goes through apoptosis, the cell envelope remains intact while the death receptor and mitochondrial mechanisms cause cell death. (B) Tumor hypoxia and vascular disruption: PTT disrupts the tumor vasculature, making the tumor hypoxic and encouraging cell death. (C) Extracellular matrix changes and ICD: PTT induces the synthesis of DAMPs, which impact cytotoxic T cells and DCs, resulting in ICD. PTT also promotes ECM rearrangement by damaging collagen, the major component of the ECM, via DDR1 and MMPs (Xiong et al. 2023).53 | |
2.4. PDT in tumor cell death
In order to kill target tumor cells, photodynamic therapy (PDT) primarily uses the excited state of a photosensitizer to produce singlet oxygen. PDT is applicable in a number of cancerous conditions.58 It is a method that makes use of certain substances that function as photosensitizers.59 These substances are dormant unless exposed to a certain kind of light. Additionally, oxygen must always be present for them to function. ROS are produced by the photosensitizer (PS), that is triggered by light. Consequently, ROS are in charge of effector processes like tumor cell death.60 An electron moves to its initial excited singlet state when the PS is activated by light. An intersystem crossover that comes next produces a triplet state. Reactive singlet oxygen (1O2) is produced when the triplet PS transmits energy to the triplet oxygen. 1O2 has a wide variety of effects, including the direct destruction of cancer cells, vascular damage, and immune response induction. PDT can also cause necrosis and apoptosis in cancer cells.61 Furthermore, PDT may damage the tumor's vascular systems, depriving cancer cells of essential nutrients and causing them to become hypoxic.62,63 Recent research has demonstrated that ROS have a variety of biological impacts. While direct tumor cell death is one of PDT's most powerful effects, immunological responses generated by PDT also have the ability to significantly impact the treatment's overall efficacy.60 PDT may ultimately result in either immune system activation or inhibition.64
The use of optic fibers, which could penetrate interstitial tumor tissues, solved the challenge of delivering light to the cancer location other than surface ones. These developments made it possible to employ PDT to treat a variety of cancers, such as those of the pancreas, prostate, head and neck, and others.65 For therapy of interstitial tumors, the PS can be given intravenously or orally. Then, at the targeted tissue region, it is triggered using a certain light wavelength. This may be accomplished with a laser. Through optical fibers contained within clear plastic needles, light may be administered to the desired tissue.59 PDT can make use of particular kinds of laser or light-emitting diodes (LEDs). The type of light that is employed depends on the type of tumor tissue and its location inside the body. The placement of the guides, which are often done under general anesthesia, uses ultrasonography to assist the targeting of the tumor tissue accurately. There is a lag time between the patient receiving the medication and the light treatment. Drug-to-light interval refers to this time frame, which can range from hours to days according on the PS being used (e.g., pharmacokinetic properties). The majority of PDT applications are typically carried out in an outpatient environment and do not necessitate hospitalization.58
The overall tumor-eradication impact of PDT involves many unique pathways.61,66 The immune system of the host may be boosted by PDT to combat tumor cells.60 While most traditional anti-cancer treatment techniques, like radiation and chemotherapy, suppress the immune system, PDT can stimulate inflammation, draw leukocytes to the affected area, and help activate T cells that fight cancer. PDT also reduces the microvasculature of the tumor, depriving the malignant tissue of nutrition and oxygen.60,67 Finally, but just as importantly, PDT can directly destroy tumor cells by causing necrosis or apoptosis by the use of active O2. Most likely, there are connections between these systems. Certain processes may hold significance in varying therapeutic contexts, contingent upon the tumor type and PS. Not only do tumor tissues have parenchyma, or cancer cells, but they also contain stroma.68 The components of the tumor microenvironment include extracellular matrix, fibroblasts, vascular structures, and immune cells. The majority of stromal components serve to promote the development of tumors. PDT can have an impact on the majority of tumor milieu components.58 To a certain extent, PDT can directly eradicate tumor cells, however this kind of removal can't be accomplished by a single method.69 PDT has the ability to lower the quantity of tumor cells that are clonogenic. It was observed that immediately following the conclusion of light illumination of photosensitizer-treated tumors, a reduction of up to roughly 72% may be achieved in the overall number of clonogenic cells. PDT directly causes target tumor cells to undergo a combination of necrosis and apoptosis.61 PDT has the ability to cause apoptosis quickly. Furthermore, it was claimed that PDT might cause autophagy, a crucial conserved cellular recycling system, to cause cancer cells to die.70 Also, these pathways may manifest simultaneously, contingent upon the kind and concentration of the photosensitizer.71
Agarwal et al. showed that PDT might cause apoptosis and cause DNA to break down into fragments. Time and dosage had an impact on the DNA fragmentation. They also discovered impairment of cytoplasmic structures and the appearance of chromatin condensation at the nucleus's perimeter.72 A number of photosensitizers have an impact on the mitochondria, and PDT has been shown to be a powerful inducer of apoptosis under a variety of circumstances. Many studies have looked at the processes underlying PDT-induced cell apoptosis along with the effect of signaling pathways on response to PDT.73–79 Apoptosis induction mediated by PDT involves caspases and members of the Bcl-2 protein family. On the contrary, while the apoptotic process is blocked or occurs concurrently with apoptosis, autophagy can occur without the involvement of Bax.71 It is well known that a number of cancer treatments, such as PDT, target apoptotic pathways.80–86 PDT has the capacity to trigger many apoptotic pathways. PDT-induced apoptosis is linked to both the intrinsic (facilitated by mitochondria) and extrinsic (facilitated by death receptors) pathways. The kind of apoptotic pathway depends on the photosensitizer and cancer cell types. PDT mostly activates caspase-3 or caspase-7 by activating the intrinsic route.87 The intrinsic route, which causes caspase-dependent and caspase-independent apoptosis in PDT, depends critically on mitochondria. It should be mentioned that PDT mainly initiates apoptotic pathways that are dependent on caspase.71 The photosensitizer's intracellular location influences the effectiveness of inducing apoptosis in PDT as well. Accordingly, localized photosensitizers on mitochondria are highly effective apoptosis inducers.61 Numerous investigations have revealed significant information on the critical processes linked to mitochondrial events and the apoptotic pathways which are activated in response to PDT. In the context of PDT, a number of apoptotic mediators and signaling pathways are being discovered.88 Apart from in vitro investigations, other research works shown the in vivo apoptotic consequences of photodynamic tumor treatment.89 The perturbation of the mitochondrial transmembrane potential is one of the early processes in PDT-mediated apoptosis that has been documented. Loss of potential for the mitochondrial membrane may occur quickly after PDT.90,91 The hypothesis put forth by Kowaltowski et al. was that the PDT agent's effects on inner membrane permeation to protons could lead to impairment of mitochondrial membrane potential.92 According to a number of studies, photosensitizers targeting the mitochondria may effectively cause apoptosis in PDT.93–99
PDT has been linked to mechanisms such as cytochrome c release and caspase activation, particularly caspase-9 and caspase-3.100,101 These results are consistent with the numerous investigations that have examined the function of caspase-3 in PDT-mediated apoptosis using a range of photosensitizers.102 Additionally, it has been found that PDT modifies a number of signaling pathways linked to oxidative stress responses.103 In conclusion, it is clear that PDT has a strong apoptosis-inducing effect.61 However, PDT's ability to totally eradicate malignancies in a clinical context is still lacking. The current status has been explained in a number of ways. According to some research, the effectiveness of photodynamic therapy (PDT) in killing tumor cells declines as the cells get farther away from the blood supply. This might have serious consequences due to the uneven distribution of photosensitizer inside the tumor tissue.104 Furthermore, the oxygen quantity and accessibility in the tumor tissue might affect PDT's effectiveness. Many tumor tissues demonstrate hypoxia. Furthermore, PDT employs oxygen in photodynamic processes. Not to add, PDT can injure vascular tissues and cause oxygen deficit. PDT has been observed to produce abrupt decreases in oxygen levels of tissue. According to Pogue et al., the hypoxic tissue regions showed abrupt reduction in partial oxygen pressure following treatment, but the greater partial oxygen pressure regions showed heterogeneity and some parts retained their partial oxygen pressure value. On the other hand, in a situation whereby the photosensitizer was administered three hours before to the administration of light, another study found that the partial oxygen pressure in the tumor tissue rose after the light exposure was completed.105,106 In actuality, tissue hypoxia could restrict PDT's therapeutic efficacy. The PDT irradiation process may fragment, allowing oxygen to be replenished in the tissue.107
2.5. PTT in tumor cell death
In photothermal treatment (PTT), laser energy is converted into the heat to destroy cancer cells using nanoparticles placed inside tumors as exogenous energy absorbers. PTT can be a viable substitute for traditional cancer treatment, but in some cases of radiation exposure, it can cause necrosis of cell. This necrosis may elicit pro-inflammatory responses that are detrimental to the treatment's progression. It is desirable when PTT can be adjusted to cause apoptosis instead of necrosis since apoptosis inhibits an inflammatory response, as evidenced by recent research. Several NP compositions are being investigated by researchers as PTT transducers. The ability to capture NIR light, with an extensive absorption cross section to improve light-to-heat exchange, diameter around 30 and 200 nm that promote lengthy circulation and increased tumor growth, and lowest toxicity/maximum biocompatibility are among the main design factors. Although PTT has advanced at an astounding rate from idea to clinical testing, there aren't many in-depth research that look at the fundamental biological response to PTT. To optimize treatment efficacy and reduce the risk of unfavourable side effects, it is critical to comprehend the kinetics and causes of cell death brought on by this method.108
Necrosis and apoptosis are the two types of cell death that PTT may cause. The breakdown of plasma membrane stability and consequent leakage of intracellular components such as DAMPs (damage-associated molecular patterns), within the external to cells environment are hallmarks of necrosis. Because of the potential for harmful inflammatory and immunogenic reactions brought on by this aberrant release, necrosis is an undesirable mode of cell death. In contrast, during apoptosis, the reliability of the cell membrane is preserved, and signals such as phosphatidylserine (PS) are sent to the extracellular domain of the membrane, designating a cell for phagocytosis. When apoptotic cells come into contact with phagocytes, they undergo a transformation that inhibits inflammation, which is a different and preferable result than what happens during necrosis. Nevertheless, an apoptotic cell may potentially lose its membrane integrity and discharge its internal contents, including DAMPs, if phagocytes do not quickly remove it. When phagocytes are not present, a process referred to as secondary necrosis can be shown in in vitro experiments.109 Currently, necrosis is the most often documented in vitro cellular responses to photothermal therapy (PTT); nevertheless, other research has indicated that, in specific light exposure settings, apoptosis may be the major cause of cell death.110 The overview of apoptosis by PTT is supported by the following data: (i) Western blotting after PTT shows loss of Bid and increased synthesis of tBid; (ii) flow cytometry after PTT shows impairment of mitochondrial membrane potential; and (iii) FACS after PTT shows activation of caspase-3. First, while PTT activates Bid, the exact method of activation is yet unknown. In the present investigation, administering cells along with caspase-8 inhibitor failed to avert apoptosis following PTT. Normally, caspase-8 activates Bid into the extrinsic apoptosis pathway.111 The mechanism of Bid–tBid separation is unknown, hence caspase-8 is not needed for PTT-mediated cell death. The most plausible reason is because during PTT, partial penetration or full lysosomal rupture caused by heating of nanoparticles which releases lysosomal cathepsins into the cytosol, that have been demonstrated to directly breakdown Bid.112 PTT causes cathepsin release and lysosomal disruption, which splits Bid and triggers apoptosis. It will be necessary to do further research on cathepsins to verify this theory. To put it briefly, after laser irradiation, lysosome rupture triggers Bid, which causes Bax/Bak oligomerization along with pore creation in outer membrane of the mitochondria. Apaf-1 facilitates the assembly of apoptosomes once cytochrome c is released. After that, the apoptosome triggers caspase-9, which in turn triggers caspase-3. Subsequently, caspase-3 initiates apoptotic cell death by acting as the executioner.108
Even though PTT is now undergoing clinical studies, not much is understood about the mechanisms of cell death that are triggered.113 The number of research using PTT as a cancer treatment is constantly rising, but there are relatively few publications carrying out a thorough investigation, making it challenging to identify a common mechanism of cell death.114 Depending on the properties associated with the tumor and the nanoparticles, the degree of internalization, the amount of energy absorbed, and the duration of the radiation, heat produced by PTT can trigger many pathways. As a result, a complicated biological reaction to PTT is to be expected.115 It has been demonstrated that apoptosis is induced by moderate temperatures up to 42–47 °C.116 Necrosis was caused by temperatures over 55 °C.117 The laser's intensity, the kind of cell, the qualities of the NP (composition, size, shape, and degree of internalization), and the surrounding environment (vascularization) all affect the temperature that is reached during irradiation.118,119 The simple existence of NPs may also change cell processes; therefore, the action of PTT cannot be solely caused by heat shock.
Numerous investigations have been conducted, concentrating on several mechanisms that may result in cellular death.120 Excessive light intensity can induce skin burns and subsequently inflammation in both healthy and tumorous cells.121 Certain nanoparticles (NPs) during PTT increase heat stress, which intensifies the generation of (ROS) that may cause damage to nearby healthy tissues.122,123 The reaction can be varied by the heat shock's duration and rate of heating (rapid or slow). When comparing radiation dose and duration, shorter radiation exposure times cause a little rise in body temperature and trigger apoptosis. Quite the opposite extending the radiation's duration raises the temperature more quickly, causing skin damage and necrosis. According to one research, PTT alters the phenylalanine route, making cells more susceptible to apoptosis. According to other studies, PTT causes the mitochondria to depolarize.124 A more thorough investigation has identified the intrinsic apoptotic pathway in which a proapoptotic protein Bid plays an essential part during the permeabilization of mitochondria as the mechanism causing cell death triggered by moderate PTT.111 Additional research revealed a reduction in Akt and Erk activation, which lowers the action of several proapoptotic protein inhibitors and promotes mitochondrial membrane permeabilization.124
2.5.1. PTT and autophagy. The stimulation of autophagy as a protective mechanism towards oxidative stress has been linked to gold nanoparticles. Nevertheless, another research on autophagy, which examined the production of autophagosomes as well as the p62 degradation, revealed a buildup of autophagosomes together with a blockage in autophagic flux.125,126 Damage to proteins and organelles brought on by elevated temperatures triggers autophagy, a defense mechanism against PTT. According to some studies, autophagy inhibition boosts PTT's effectiveness. As a result, designing dual chemo-PTT with autophagy inhibitors might offer a novel way to boost PTT's effectiveness.127 But taking into account not only individual cells but the entire body, autophagy plays a role in presenting antigens and may thus enhance the immune response to tumors, indicating that autophagy shouldn't be suppressed.128
2.5.2. PTT and heat shock proteins. HSPs are usually expressed when the temperature rises. The conserved family of chaperones known as HSPs binds to denatured proteins under heat stress to stop them from aggregating and to help them refold when the temperature returns to normal.129 In non-stressful circumstances, HSF1, a heat shock factor (HSF), binds to HSP90 and HSP70. HSF1 gets released and migrates to the nucleus after which it binds to DNA to activate HSP genes when HSP90 and HSP70 connect to unfolded proteins during heat shock. Consequently, it is anticipated that HSPs will engage in processes that PTT initiates.130 Inhibiting apoptosis and protecting against high temperatures, HSP70 binds to unfolded proteins therefore plays a protective function in cellular recovery. Certain malignancies have been shown to overexpress HSPs, and some research lends credence to the theory that blocking them might increase the susceptibility of the tumor to PTT.131 As previously stated, the immune system cannot be disregarded, though, as HSP70 can convey antigen to DCs and its expression may thus trigger an antitumoral immune response.132
2.5.3. PTT and immune activity. A potentially effective next-generation therapeutic strategy is cancer immunotherapy. Numerous research has demonstrated the effectiveness of this strategy. However, creating a common approach for many cancer types is challenging due to the intricate immune response. Thankfully, a novel method for eliciting an anticancer immune response by PTT with inorganic NPs has been found.133 Tumor ablation produces cell residues which can function as tumoral antigens produced in situ, which is why PTT is advantageous when used in conjunction with immune treatment. As a result, a single, distinctive method produces several distinct immune responses to a wide range of solid tumors. On the other hand, these tumor antigens have the potential to stimulate an inflammatory response, which might result in either acceleration or repression of tumor development.134 Furthermore, in order for the immune response to be triggered, a stimulation signal is required. Necrosis cells emit constituents of their own that may be antigens or DAMPs that stimulate DCs and stimulate the immune system as a whole. However, apoptotic cells often do not produce DAMPs and instead display “tolerate me” signals. They additionally deliver antigens to DCs containing phagocyted apoptotic material. As a result, the immunological reaction is inhibited.135 Co-stimulators enhance the stimulation of the antitumoral immune response when used with PTT. This is the reason why some works include an adjuvant—a material that boosts the immune system's reaction to an antigen—in the construction of the NPs.136 However, gold nanoparticles have the ability to stimulate DCs and macrophage's immune systems, which increases the release of proinflammatory cytokines. As a result, gold nanoparticles could have advantageous properties that might be used.137 In order to preserve immunological homeostasis and guard against improper immune response activation, the immune response features checkpoints. PD-1 (Programmed death-1) and CTLA-4 (cytotoxic T lymphocyte-associated antigen-4) are negative regulators of the immune response; hence, blocking them strengthens the immunological response.138 It is vital to notice that necrosis promotes tumor development whereas apoptosis is thought to be an immunosuppressive method of cell death. As a result, there are differences in the methods used to control the immune response triggered by each cell death pathway. There has to be more research done on the immune response's balance between tumor necrosis and apoptosis since the immune response that is triggered after PTT is not well understood.111
3. Photosensitizers in PDT
The existence of photosensitizers is essential components of photodynamic therapy (PDT), along with oxygen and light. These elements are described as materials that have the ability to absorb light at a certain wavelength and cause photophysical or photochemical processes.2
It is possible to identify a collection of traits and circumstances that characterize the perfect photosensitizer: (i) extremely pure chemical composition, (ii) steadiness at ambient temperature, (iii) the photosensitive effect is only present when a certain wavelength is present, (iv) the photochemical reactivity is high, and the optimal range of wavelengths for maximal light absorption is between 600 and 800 nm. Light having a wavelength greater than 800 nm cannot be sufficiently absorbed to produce further reactive oxygen species or to excite oxygen in its singlet form, (v) a minimum amount of absorption between 400 and 600 nm. By doing this, sunlight-induced hyper photosensitivity is potentially avoided, (vi) no chemical in the human system, including endogenous pigments like melatonin, haemoglobin, or oxyhaemoglobin, should have its absorption bands overlapped, (vii) easy solubility in bodily tissues, (viii) reduced cell death in the dark, (ix) high affinity for neoplastic tissues: to reduce the photo toxic adverse effects of the therapy, the photosensitizer must be rapidly withdrawn from healthy tissues but gradually removed from problematic regions, where it should remain for at least a few hours, (x) easy availability and synthesis at a low cost.139
3.1. 1st generation photosensitizers
Oscar Raab, a Munich-based medical student, is credited with pioneering the use of light with a photosensitizing chemical. Raab discovered that fluorescence happens in protozoa that have been dye-treated and subsequently exposed to radiation while conducting studies with acridine dyes. This occurrence caused oxygen to be consumed and had a toxic impact, which killed protozoa. In 1904, Raab showed Professor von Tappeiner his data, and he clarified and labeled this phenomenon as a “photodynamic effect”.140 Soon after, in 1905, the first successful effort to cure skin cancer with 5% eosin dye was conducted. But this treatment was not widely adopted and was ignored for many years.141 It was in the 1970s when Dr Thomas Dougherty along with others first commercialized photosensitizers for use in medical therapy.142 They were evaluating a porphyrin combination known as the “hematoporphyrin derivative” (HpD), which is soluble in water. Separation and chemical treatment of hematoporphyrin (Hp), the very first porphyrin used as PS, produced HpD. When it came to malignancies, HpD exhibited superior tissue selectivity and less photosensitizing capability on the skin than Hp. Later, a blend of dimers and oligomers of porphyrin that were separated from HPD was sold under the brand name “Photofrin.” As of right now, sodium porfimer, also referred to as Photofrin, is still the most widely used PS Fig. 4.6,143 Even though PDT is widely used, the therapeutic uses are limited by its low purity of chemicals (it is a combination of over 60 compounds) and poor tissue penetration because of its greatest absorption at 630 nm, a very short wavelength. In addition, a few weeks following PDT, skin becomes hypersensitive to light due to the prolonged half-life of PS and the elevated levels in the skin. The shortcomings associated with the first-generation PS made it necessary to look into other substances, which sparked the creation of a second-generation photosensitizers.144
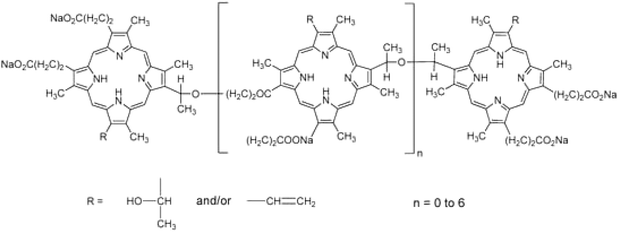 |
| Fig. 4 First generation photosensitizer – Photofrin (Chen et al., 1995).6 | |
3.2. 2nd generation photosensitizers
Research on the upcoming generation of photosensitizers started as early as the 1980s. Only a small number of the several hundred compounds with possible photosensitizing qualities that had been suggested have been employed in clinical studies. Even fewer compounds have regulatory approval for clinical use during photodynamic therapy (PDT) against cancer.145 Derivatives of hematoporphyrin and synthetic photosensitizers, such as texaphyrins, 5-aminolevulinic acid, thiopurine derivatives, benzoporphyrin derivatives, chlorin, and bacteriochlorin analogues and phthalocyanines, are currently included in the category of second-generation photosensitizers Fig. 6.146 Utilizing 5-aminolevulinic acid (ALA), the precursor to protoporphyrin IX, proved to be a significant finding. ALA is a type of a prodrug that requires transformation into a protoporhyrin in order to become an active PS. Because of this, ALA and its esters have a wide range of therapeutic uses, both topically and orally Fig. 5.147
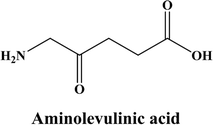 |
| Fig. 5 Structure of aminolevulinic acid (Morton et al., 2002).147 | |
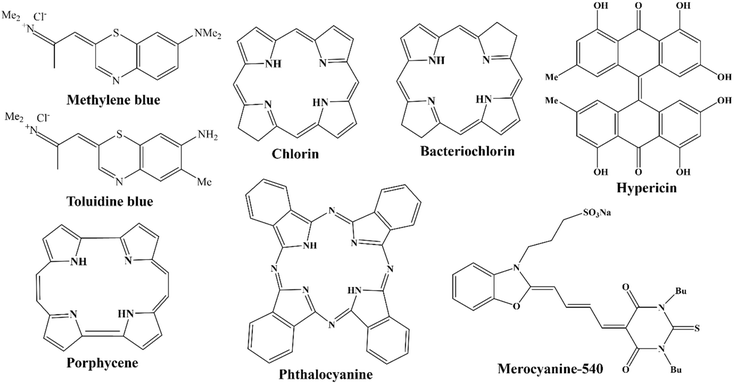 |
| Fig. 6 Second generation photosensitizers (Chen et al., 1995).6 | |
The photosensitizers of the second generation exhibit enhanced chemical purity, higher levels of generation of singlet oxygen, and superior penetration into deeply situated tissues because of their greatest absorption in the 650–800 nm wavelength range. Because of their increased selectivity for malignant tissues and quicker bodily removal of the photosensitizer, they also show less adverse effects. The primary drawback of second-generation PS is its low water solubility, which severely restricts their use via IV and necessitates the development of novel drug delivery strategies.148
3.3. 3rd generation photosensitizers
The production of materials with a greater affinity for tumor tissue provides the foundation for the creation of third-generation photosensitizers, which lessen harm to nearby, healthy tissues. Preparing a pharmacological process that would allow photosensitizers to be administered parenterally is another obstacle to the broad clinical implementation of photodynamic therapy in cancer. The photodynamic method's bioavailability can be efficiently increased by new drug delivery techniques that are on the rise.149
To improve the drug's selectivity, photodynamic treatment is modified in the following ways: (i) combination of targeting agents with second-generation photosensitizers, (ii) mixtures of photosensitizers and low-density lipoprotein, as the growing tumor cells require higher levels of cholesterol in production of their cell walls, (iii) using tumor surface indicators like growth factor receptors, hormones (like insulin), as well as transferrin receptors Fig. 7. (iv) In combination with a photosensitizer and a monoclonal antibody that recognizes cancer cell antigen.150,151
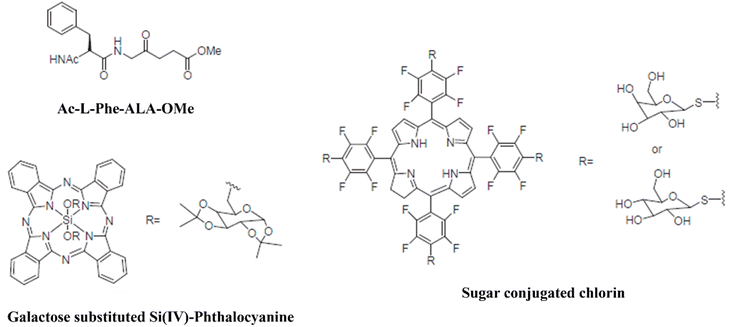 |
| Fig. 7 Several examples of third-generation PSs that have been coupled with targeting agents (Lee et al., 2005).150 | |
4. PDT and nanotechnology
In the past ten years, there has been a notable acceleration in the advancement of nanotechnology. The field known as “nanomedicine” employs nanomaterial systems for diagnostic and therapeutic procedures, which makes it possible to precisely deliver drugs to target tissues and enhance the efficacy of cancer treatment. The incorporation of photosensitizers along with nanomaterials may improve the efficiency of photodynamic therapy and as well get rid of its side effects. The application of nanoparticles allows for the achievement of a targeted method that is focused on particular receptors, which in turn enhances the specificity of photodynamic therapy. PS's can be encapsulated within or immobilized upon nanoplatforms through covalent, noncovalent interactions.152
4.1. Photosensitizers in photodynamic therapy
The therapeutic uses of traditional organic photosensitizers (PSs) have been mainly restricted by their poor stability and hydrophobicity in the PDT environment, and reduced cell/tissue selectivity, despite the fact that PSs are still frequently employed and have made considerable advancements in PDT. As a result, intriguing nano-agents with optical and physicochemical qualities have surfaced as a compelling substitute to get over these limitations of conventional photosensitizers. Although certain first-generation PSs has been granted approval for clinical use, their limitations such as hydrophobicity and shallow penetration depth-as well as the decreased cell and tissue specificity of second-generation PSs continue to impede their potential as clinical therapeutics. A lot of focus has been on the study of nanomaterial-based photodynamic therapy (PDT) during the last few years. This is a novel treatment approach that use nanomaterial as a PS or carrier.
Nanomaterials have several special qualities which render them more effective for PDT as compared to organic PSs.6 Nanomaterials are radiation-stable and have exceptional optical characteristics that enhance PDT's penetration and effectiveness.153 Furthermore, molecule-modified nanocarriers can more accurately distribute PS to the targeted cells than conventional PSs, which translates into a better therapeutic benefit and fewer adverse effects.154,155 Because of their unique light absorption characteristics, certain nanomaterials, such as semiconducting nanoparticles, have the innate ability to create ROS through the transfer of energy or electron transfer process.156 Because of their vast surface area and adaptable surface changes, nanomaterials are increasingly used as PS carriers, enabling targeted distribution of photosensitizers toward the tumor location through improved drug loading efficiency as well as better uptake by the cancer cells.157
4.1.1. Gold nanoparticles. The simplicity of surface modification using gold-thiol chemistry, biocompatibility, and huge surface areas of these gold nanoparticles have made them the subject of much investigation for years as a means to induce PDT.158 Since gold nanoparticle's optical dispersion and absorption may be adjusted, they are also being investigated in great detail for diagnostic purposes.159 The Hwang group reported in 2014 the first application of gold nanorods (Au NRs) for PDT on their own (without using an organic PS). It was discovered that gold nanorods could create singlet oxygen under the stimulation of near-infrared light (915 nm, λ1), which caused the B16F0 melanoma cancers in mice to be destroyed. Heat shock protein (HSP 70) generation indicates the activation of gold nanorods at 780 nm (λ2) which can raise the temperature surrounding tumor tissues in addition to generating 1O2. This is because photon energy can be converted to heat. Gold nanorods primarily produced the so-called photothermal treatment (PTT) impact in this procedure, which caused cancer cells to undergo apoptosis. PDT and PTT are the two dominant phototherapeutic effects that may be altered by varying the stimulation wavelengths (λ1 or λ2) Fig. 8.160
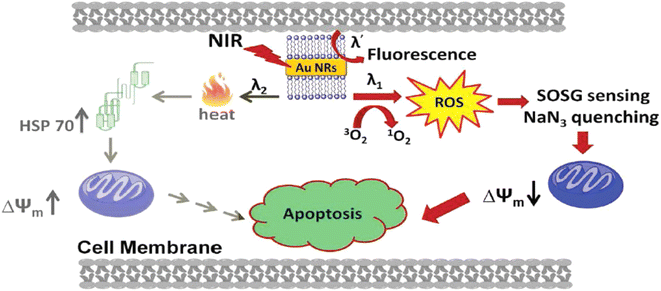 |
| Fig. 8 Various cellular pathways involved in cellular death produced by PDT/PTT effects caused by gold nanorods after photoexcitation (Vankayala et al., 2014).160 | |
Prior research has demonstrated that metal nanoparticles may produce singlet oxygen using the one-photon excitation method. Nevertheless, the surrounding tissues near the tumor site may sustain photodamage as a result of one-photon stimulation. In this regard, two-photon excitation with accurate treatment dosage modification is more desirable.160,161 Gold nanorod and gold nanosphere aggregates have been evaluated as photosensitizers for two-photon PDT in the work of Jiang and colleagues. Either, individual or clustered gold nanoparticles were used to demonstrate two-photon induced production of singlet oxygen upon exposure to femtosecond laser pulses at 800 nm. Aggregated gold nanoparticles, however, typically improved the 1O2 production capabilities. In other words, aggregated gold nanospheres were 8.3 times more capable of generating 1O2 than unaggregated ones.162
4.1.2. Silver nanoparticles. These days, antimicrobial treatments also make extensive use of silver nanoparticles. The fact that silver nanoparticles often have an extraordinarily high specific surface area – meaning that the surface area of silver that is in contact with microorganisms can be very large is one of its fascinating characteristics. The capacity of silver nanoparticles to produce singlet oxygen has also been discovered, which has led to a lot of research into their application in PDT.33 According to Hwang et al., silver nanoparticle-mediated excitation and singlet oxygen production are closely correlated with their shape, just like in the situations of gold nanoparticles. For example, using silver nanocubes produced either a little or absence of 1O2 phosphorescence signal, whereas stimulation of silver decahedrons as well as silver triangular nanoplates could generate singlet oxygen. A notable singlet oxygen production was seen during silver decahedrons photo-excitation (approximately 520 nm LSPR band) in NIR wavelength of 885 nm. Similarly, at 544 nm excitation, silver triangular plates (about 590 nm LSPR band) produced a robust 1O2 phosphorescence signal, whereas radiating silver nanocubes (about 500 nm LSPR band) in 525 nm produced only a faint singlet oxygen production.163 Silver nanoparticles have a variety of shapes and compositions that can result in highly adjustable optical characteristics, which makes them appealing for use in photodynamic therapy. Furthermore, cancers can experience oxidative stress from silver nanoparticles, which triggers the death of tumor cells.164In a work by Mahajan et al., a new porphyrin derivative 5,10,15,20-tetrakis(2,4-dihydroxyphenyl) porphyrin (POR) was synthesized and loaded on the surface of readily manufactured MSA-capped silver nanoparticles. The efficiency of singlet oxygen quantum yield was found to be greater when POR was integrated into MSA-AgNPs rather than in its free form. Furthermore, the produced nanocomposite might be a promising option for cell imaging applications against the cancer cell line A375, with non-toxic properties.165 Khoza et al. evaluated the complex's in vitro photodynamic activity using a metastatic melanoma cancer cell line. When exposed to red light in the presence of varying amounts of phthalocyanine (complex 3) and silver nanoparticles, cell viability decreased proportionally. The photodynamic activity of complex 3 increased in the presence of AgNPs.166
Montaseri et al. studied the notion of photodynamic therapy (PDT) using silver-based nanohybrids to treat A375 melanoma cancer cells. Ag-PEG NPs and core/shell Ag@mSiO2 NPs were synthesized and attached to zinc phthalocyanine tetrasulfonate (ZnPcS4) photosensitizers (PS). Folic acid (FA) was also used as a targeting moiety on the surface of nanohybrids to specifically target the overexpressed folate receptors in A375 cells. Cells subjected to 674 nm laser irradiation after incubation with ZnPcS4/Ag@mSiO2-FA had a strong PDT impact, with ∼92%* ± 1.1 cell death compared to ∼70%* ± 2.9 cell death for ZnPcS4/Ag-PEG-FA nanohybrids. This was due to the increased formation of ROS in the former nanohybrids. As the nanohybrids successfully localized in mitochondria, the bulk of cell death was caused by apoptosis rather than necrosis.167
4.1.3. Silica and silicon nanoparticles. Due to its reduced toxicity, chemical inactivity, and optical transparency, nanoparticles of silica can be utilized to encapsulate PSs in PDT even though silica doesn't seem active for PDT itself. Because silica has hydroxyl groups on its surface, chemical functionalization of the material is also possible. In these kinds of investigations, silica nanoparticles are typically employed for drug delivery.153 Yan and colleagues used a modified Stöber sol–gel technique in 2007 to embed the PS m-THPC (meta-tetra(hydroxyphenyl)-chlorin) on matrix-based sol–gel silica nanoparticles.168 The ability of organically manipulated silica (ORMOSIL) nanoparticles to effectively conjugate with hydrophilic and hydrophobic compounds makes them a viable vehicle for PS drug delivery.169 When PS molecules were covalently bonded to ORMOSIL nanoparticles in 2007, Ohulchansky and colleagues observed that the molecules could produce singlet oxygen with stability upon excitation while maintaining their functional and spectroscopic characteristics.170 The unique qualities of mesoporous silica nanoparticles (MSNs), such as a large surface area, pore volume, and chemical stability, make them a frequent choice for PS distribution. Hydrophobic PSs, such zinc picolinate (ZnPc), which have a tendency to self-aggregate in aqueous media, can be transported by MSNs Fig. 9.171,172 In 2012, MSNs modified with galactose on their surface were reported for use as PDT agents and delivery vehicles.173,174 Galactose functionalization can boost absorption by cancer cells via galactose receptor-mediated endocytosis, which leads to the aggregation of the nanomaterials in the lysosomal and endosomal compartments, according to confocal microscopy tests. As the synergistic anticancer effects of porphyrin (first generation PS) and camptothecin anti-cancer medication were evaluated on MSNs, the results demonstrated a significant increase in cancer cell death under illumination at 650 nm as compared to individual treatments.175 Different synthetic techniques may be used to create a range of silicon and silica nanoparticles with ideal sizes and porosities for PDT. Additionally, by surface functionalizing the silicon-based nanoparticles, cancer-specific medicines may be added, enhancing their capacity to target tumours. Furthermore, their potential applications in two-photon PDT and NIR for profound cancer treatment have been demonstrated by recent investigations.172
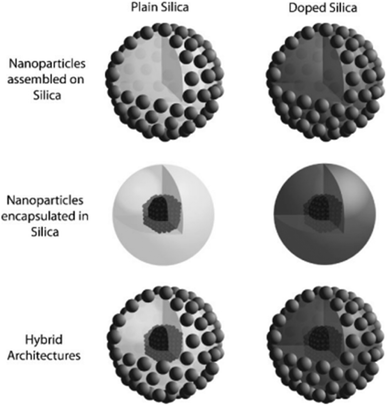 |
| Fig. 9 Several topologies for generating multipurpose silica nanoparticles are demonstrated, including employing the silica matrix as a center, shell, or hybrid design, and adding components such as molecules and atoms (Piao et al., 2008).171 | |
Li et al. used mesoporous silica nanoparticle (MSN) as a nanoplatform. SiNPs and the photosensitizer 5,10,15,20-tetrakis (1-methyl 4-pyridinio) porphyrin tetra (p-toluenesulfonate) (TMPyP) were first embedded in the MSN and was further modified with folic acid (FA) to obtain the mesoporous silica nanocomposite (MSN@SiNPs@TMPyP-FA) for targeted two-photon-excited fluorescence. The integrated TMPyP could create singlet oxygen for PDT under light irradiation, and the anticancer medication doxorubicin (DOX) could be loaded for chemotherapy. Furthermore, due to SiNPs' two-photon stimulated fluorescence, the nanocomposite enabled targeted two-photon fluorescence cellular imaging at near-infrared (NIR) laser excitation, effectively avoiding interference from biological auto-fluorescence. In vitro cytotoxicity studies demonstrated that the combination treatment of PDT plus chemotherapy had a high therapeutic effectiveness against cancer cells.176
In another work, a new photo-responsive hollow silica nanoparticle (HNP)-based gene and photosensitizer (PS) co-delivery nanovehicle was developed for dual-wavelength light-triggered synergistic gene and PDT treatment. The resulting HNP coupled with PDMAEMA polycation via a 405 nm light-cleavable Cou-linker, known as HNP-Cou-PD, has great gene condensation capacity, good biocompatibility, exceptional PS loading ability, and light-triggered gene release features. HNP-Cou-PD with Chlorin e6 (Ce6) loaded inside the silica cavity and a plasmid encoding caspase-8 gene (CSP8) attached to the PDMAEMA outside layer (Ce6–HNP-Cou-PD/CSP8) has been shown to have better antitumor effects under pre-405 nm and post-670 nm light irradiation both in vitro and in vivo due to light-triggered intracellular gene release and reactive oxygen species (ROS) generation.177
Dina Aggad et al. described ethylene-based periodic mesoporous organosilica nanoparticles (PMOs) for PDT and the autonomous administration of gemcitabine hydrochloride, an FDA-approved chemotherapeutic medication, in cancer cells. Depending on the nature of the photosensitizer (tetrasiylated or monosilylated porphyrin) and its aggregation state, they may create two-photon PDT. The synergistic impact of two-photon irradiation with gemcitabine increased the percentage of cell death by approximately 20% compared to the delivery procedure without irradiation.178 Özge Er et al. developed mesoporous silica nanoparticles loaded with zinc(II) 2,3,9,10,16,17,23,24-octa (tert-butyl phenoxy phthalocyaninato (2-)-N29,N30,N31,N32 (ZnPcOBP) as a photosensitizer to determine the production of singlet oxygen and achieve in vitro PDT against pancreatic cancer cells. When ZnPcOBP was integrated into silica nanoparticles, it demonstrated a strong phototoxic impact, which was exacerbated by cetuximab. Cetuximab is a monoclonal antibody that primarily targets the epidermal growth factor receptor. Thus, the imidazole was a good carrier for the selective delivery of ZnPcOBP to pancreatic cancer cells (ASPC-1, PANC-1, MATpaca-2) in vitro.179
Qi Sun et al. were the first to disclose that gold (Au) nanorods were capped to chlorine e6-doped mesoporous silica nanorods for a single wavelength of NIR light-triggered combination phototherapy (PDT and PTT). This single wavelength of light, along with the rod form of the nanosystem for combination phototherapy, exhibited the following unique characteristics: (1) the impact of PDT and PTT could be realized under a single wavelength of near-infrared light (660 nm), making the therapeutical procedure viable and straightforward; (2) chlorin e6 is doped into a mesoporous nanorod but remains in the nanocarrier throughout delivery. AuNRs-Ce6-MSNRs are not only capable of producing single oxygen for PDT based on chlorin e6 after uncapping gold nanorods under single NIR irradiation, but they can also create heat to execute the PTT effect based on AuNRs.180 In a separate investigation, the ICG was combined with doxorubicin hydrochloride to form hollow mesoporous silica nanoparticles and dopamine-modified hyaluronic acid (DA-HA) to serve as targeting agents and gatekeepers for HMSNs via boronate ester linkages. In vitro cell culture investigations revealed that the ID@HMSNs-B-HA nanoplatform (where ID represents both DOX and ICG) may suppress murine mammary cancer cells (4T1) using a combination of PDT and chemotherapy.181
4.1.4. Quantum dots (QDs). QDs, a different kind of nanomaterial, have also been thoroughly researched for use in PDT. QDs are potential nanocarriers because of their unique optical features, which include high emission quantum yield, adjustable release based on size and composition, and easy surface modification Fig. 10.183,184 QDs also contribute to fluorescence resonance energy transfer (FRET), a popular biomolecular dynamics approach.182 In a study by Qi et al. (2010) where biocompatible CdSe QDs containing water-soluble porphyrin was produced for PDT using two-photon excitation. The results showed that the 1O2 quantum yield from porphyrin-conjugated QDs following two-photon stimulation was two times greater than from the porphyrin solution alone.185 Shen and colleagues recently described a QD-based hybrid nanocomposite that targets tumors by encapsulating a QD-Zn-porphyrin (TMPyPZn-QD) nanocomplex, an extremely fluorescent photosensitizer rhodamine 6G (R6G), and NIR775, an NIR fluorophores found in FA-decorated phospholipid polymers. These nanoparticles have a significant capacity to absorb light and may be significantly loaded with porphyrin. Consequently, an efficient dual energy transfer technique was used to generate an exceedingly high level of singlet oxygen quantum yield of around 0.91. Furthermore, the folate receptor may play a role in the highly targeted distribution of as-prepared nanoparticles to malignant tissues, enabling effective photodynamic tumor elimination in vivo and non-invasive fluorescent imaging without harming the nearby healthy cells.186
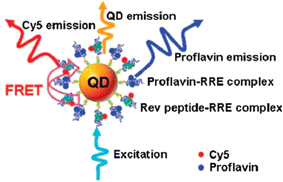 |
| Fig. 10 A single-QD-based nanosensor can detect Rev peptide-RRE association and proflavin inhibitory efficacy using FRET between 605QD and Cy5 (Zhou et al. 2005).182 | |
Murali et al. created hematoporphyrin (HP) photosensitizer-encapsulated carbon quantum dots (CQDs) (HP-CQDs) utilizing a well-controlled one-step microwave process with the HP monomer as a precursor. The HP-CQDs as synthesized preserved all of HP's fundamental optical and chemical features while demonstrating exceptional water solubility. Importantly, HP-CQDs' exceptional capacity to generate reactive oxygen species under deep red light suited its application in PDT-assisted effective eradication of human breast cancer cells (MCF-7). In comparison to HP, HP-CQDs displayed very significant phototoxicity and minimal dark toxicity towards MCF-7 cells.187 Zeng et al. used microwave-assisted synthesis to create CQDs with green fluorescence as a targeted carrier for medication delivery in mice models to treat hepatocellular carcinoma. Researchers observed non-covalent coupling of DOX with CQDs. The CQD-DOX combination increased in vivo tumor stability and delivery efficacy.188 Alaghmandfard et al. demonstrated that CQDs work as a tool for gene delivery, conjugating with polyamidoamine (PAMAM) for the treatment of triple-negative breast cancer.189
4.1.5. Carbon-based nanomaterials. Carbon-based nanomaterials are popular in PDT research because to their unique optical and mechanical characteristics, tunable chemical functionalization, excellent biocompatibility, and low toxicity. Because carbon exists in so many allotropic forms, there are a wide variety of carbon-based nanomaterials. The ones that are most frequently used in PDT include graphene-based nanomaterials, fullerenes, and carbon nanotubes (CNTs).190
4.1.5.1 Carbon nanotubes. With an average inner diameter ranging from one to ten layers, carbon nanotubes (CNTs) are essentially rolled-up single or multilayered graphene in one dimension.33 Murakami et al. demonstrated that with NIR light irradiation (808 nm), semi-conducting and metallic SWCNTs may produce ROS (singlet oxygen and O2˙−). In this investigation, it was discovered that SWCNTs had more photodynamic aftermath than MWCNTs.191 In another study, Wang and colleagues developed two modified SWCNTs by covalently functionalizing SWNTs with PEI or noncovalently functionalizing them with polyvinylpyrrolidone (PVPk30). The photodynamic ability was found to be influenced by the functionalization technique, and both of in vivo and in vitro the PEI functionalization demonstrated a greater photodynamic performance than the PVPk30 alteration.192 Recently Zhang et al. described the usage of Fe3O4@CQDs coated on PEGylated SWCNTs to create a multifunctional nanoplatform. This nanoplatform may be employed concurrently for drug release, combined photodynamic/photothermal treatment, and magnetic resonance imaging (MRI) by loading the chemotherapeutic medication doxorubicin (DOX) and combining with sgc8c aptamer Fig. 11.193
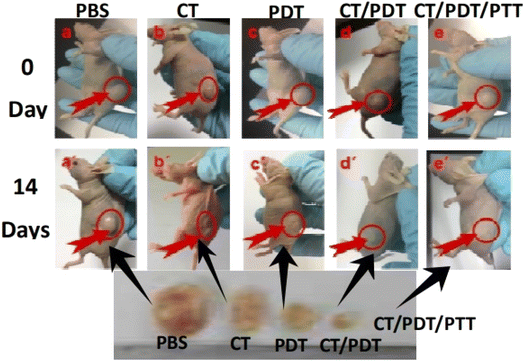 |
| Fig. 11 Image of tumor-bearing mice following various treatments (Zhang et al., 2018).193 | |
4.1.5.2 Fullerenes. Fullerenes are all-carbon materials having 3D spherical, tubular or ellipsoidal structures; the best researched example is C60. While fullerenes on their own are intrinsically poisonous and poorly soluble in water, several surface modifications have been created to address these problems. Furthermore, it has been demonstrated that fullerenes are more biocompatible than other materials made from carbon like graphene and carbon nanotubes (CNTs) Fig. 12.195 A multifunctional nanocomposite based on fullerene was described by Li et al. in 2015. Phenylalanine was used to modify C60, and polylactic acid (PLA) was used to attach it. When exposed to visible light, this as-prepared nanoplatform may very specifically deliver the anticancer medication mitoxantrone into the tumors in C57BL mice, leading the combined effects of chemotherapy and photodynamic treatment with minimal harm to the normal, healthy organs.194 Graphene oxide (GO) and C60 were conjugated, according to Li et al., to create a GO-C60 hybrid that demonstrated high stability in physiological settings. In addition to mediating the photothermal effects, GO may also transfer acquired light energy to C60, causing C60 to produce ROS when exposed to near-infrared radiation. Consequently, HeLa cells can experience synergistic PDT/PTT effects from the prepared GO-C60 hybrid in vitro.196
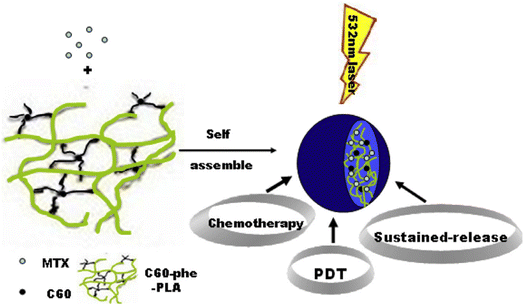 |
| Fig. 12 Schematic of fullerene-based multifunctional sustained-release microsphere and its bio-functions (Li et al., 2015).194 | |
4.1.5.3 Graphene-based nanomaterials. PDT and other forms of cancer therapy have made graphene-based nanomaterials, including GO (graphene oxide) and GQDs (graphene quantum dots), and reduced graphene oxide (rGO) are widely used because of their excellent thermal and optical characteristics, large surface area, and good biocompatibility Fig. 13.197 Ge et al. has shown that GQDs are an effective PDT agent, producing an outstanding quantum yield of 1O2 (up to 1.3). The graphene quantum dots were created utilizing the hydrothermal technique with polythiophenes serving as the carbon precursors. The killing of HeLa cells and breakdown of tumor in BALB/nu mice having breast cancer were seen during the in vitro and in vivo testing of the PDT effects of the graphene based QDs. Regretfully, photodynamic activity was only seen by the GQDs in our investigation when they were stimulated by visible light.198 When nitrogen as well as an amino group were added to the GQDs in 2018, Kuo et al. showed that the resulting amino-N-GQDs could produce exceptional amounts of singlet oxygen in the near-infrared (NIR) range (800 nm). Crucially, amino-N-GQDs were more potent PDT agents with better two-photon excitation than unmodified GQDs.199 In order to create a light-sensitive medication delivery system, Ding and colleagues put PS hypocrellin A (HA) as well as TiO2 nanoparticles over the GO surface in 2016. A mutual sensitization process was used to achieve increased ROS generation capacity. The stable complex HA-TiO2 was the source of the sensitization effect. Concurrently, adding GO to TiO2 can potentially cause it to produce ROS when exposed to light. In vitro tests that demonstrated the obtained HA-TiO2-GO nanocomposite had significantly greater capacity to eliminate cancer cells compared to the TiO2–GO or HA–TiO2 complex validated the enhanced efficacy of PDT. Additionally, the produced ROS has the ability to degrade GO, suggesting that this drug delivery method may be used in clinical PDT with regard to metabolism.200
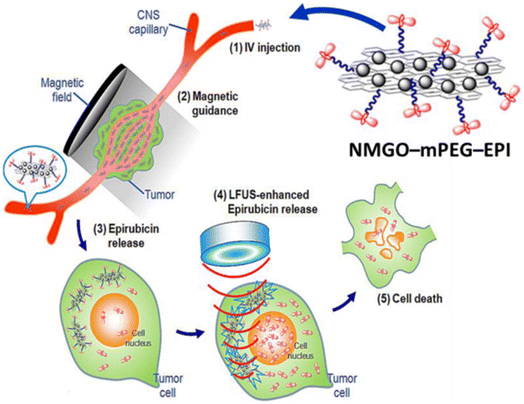 |
| Fig. 13 The molecular mechanism of effect of NMGO-mPEG-EPI in cancer therapy and LFUS-induced hyperthermia. NMGO-mPEG-EPI functions as a heat-conducting basis, causing the local temperature to increase when LFUS is employed for deep targeting.197 | |
Apart from GO; rGO has been studied for theranostic application in cancer. Zhang et al. reported on a nanosystem consisting of a PEG infused Ru(II) complex (Ru-PEG) and rGO nanosheet, used in conjunction with photothermal/photodynamic treatment and lysosome-targeted phosphorescent imaging. Using hydrophobic interactions as well as π–π stacking, the Ru-PEG complex-which functions as both as an imaging agent and PS – was adhered to rGO. Ru-PEG produced a higher quantum yield of radicals (0.06 and 0.31, respectively) under 450 nm irradiation than rGO-Ru-PEG. This difference was most likely due to the quenching action of rGO. It was shown that A549 cells with particular localization to lysosomes could better absorb Ru-PEG when exposed to rGO. When rGO-Ru-PEG was used in conjunction with PTT and PDT, the anticancer effectiveness was greater than when each therapy was used alone. In addition to causing ROS, cathepsin-induced lysosomal damage by a combination photothermal and photodynamic action has also been discovered to aid in the death of cancer cells. Additionally, photothermal imaging of rGO-Ru-PEG under 808 nm light demonstrated the strong anticancer effectiveness of the constructed nanosystem in vivo.201
A team of scientists created a BODIPY-based phototheranostic nanoparticle with photo-chemo synergistic properties, and the nanoparticle MMC-Graphene@BODIPY-mPEG (MGBP) has excellent ROS production ability, high photothermal conversion efficiency (48%), and excellent therapy efficiency (HeLa cell viability was reduced to 17% after treatment) in vitro. Furthermore, because to the strong fluorescence and anti-photobleaching ability of BODIPY and the photothermal conversion capacity of graphene, MGBP demonstrated excellent fluorescence and photothermal imaging performance in the agar model.202 Cheng et al. devised and manufactured the Fe3O4/g-C3N4@PPy-DOX nanocomposite, which contains magnetic iron oxide (Fe3O4) nanoparticles (NPs), a lamellar structure of graphite-like carbon nitride (g-C3N4), and a polypyrrole (PPy) shell with the anti-tumor medication doxorubicin hydrochloride (DOX). It is intriguing that Fe3O4 NPs have photosensitizer properties for photodynamic treatment (PDT). The use of g-C3N4 sheets in photocatalysis to degrade water and generate O2 might successfully relieve solid tumor hypoxia and raise PDT efficiency. Furthermore, PPy has a high light-to-heat conversion efficiency, making it ideal for cancer photothermal treatment (PTT). Finally, an anticancer medication (DOX) was loaded onto the nanocomposite due to its mesoporous structure. Thus, the produced Fe3O4/g-C3N4@PPy-DOX nanocomposites have a synergistic chemotherapy/PTT/enhanced PDT anticancer activity.203 Qin et al. (2018) created a nanocomposite composed of GO, magnetic nanoparticles (Fe3O4), chitosan, and a new photosensitizer HNPa (3-[1-hydroxyethyl]-3-divinyl-131-b,b-dicyano-methylene-131-deoxopyropheophorbide-a). They demonstrated a higher singlet oxygen quantum yield than the single HNPa, at 62.9% and 42.6%, respectively. Furthermore, they found that the presence of GO-Fe3O4 enhanced HNPa penetration into the nucleus of the human hepatocellular carcinoma cell line (HepG2). Furthermore, an MTT experiment conducted under 698 nm of irradiation confirmed that HNPa increased photodynamic cancer cell mortality.204
4.1.6. Two-dimensional (2D) nanomaterials. 2D nanomaterials have a huge surface area, extremely thin thickness, variable composition, and ease of surface modification, which allow them to display certain distinct and chemical and physical characteristics.205 These characteristics make them extremely desired for a wide range of possible uses, including energy storage, optoelectronics, catalysis, device manufacturing, sensing, and the detection and treatment of illness.206
4.1.6.1 Manganese dioxide (MnO2) nanosheets. One problem with PDT-based tumor therapy is that solid tumors, which are primarily hypoxic all the time, consume the ROS that are produced. Furthermore, the ROS generated by PDT drugs might also be consumed by the tumor cells attributed to the overexpression of glutathione (GSH).207 It has been demonstrated that MnO2 nanosheets may quickly raise the oxygen content of tumor tissues by interacting with H2O2 in the surrounding tissue microenvironment to regenerate oxygen and reduce GSH.208 Moreover, MnO2 nanosheets have several desirable characteristics for PS transport, including significant PS adsorption capacity owing to electrostatic interaction as well as Mn–N coordinate bonds, that might facilitate PS endocytosis in the intracellular PDT.208 Furthermore, MnO2 nanosheets exhibit strong biocompatibility because nontoxic manganese plays a crucial role in physiological metabolism.209MnO2 nanosheets were employed as a PS Ce6 nanocarrier, whereas they also served as an oxidant to deplete intracellular GSH and increase photodynamic efficiency. This nanosystem, Ce6 has been effectively loaded onto the MnO2 nanosheets also shielded from self-destruction by MnO2 when exposed to light, facilitating the efficient delivery of PS to the cytoplasm. Surprisingly, the as-prepared Ce6–MnO2 nanocomplex performed better in killing MCF-7 cells under the author's circumstances than other nanomaterial-based composites (such Ce6-GO and Ce6-MSN). Over 95% of cells were killed by the Ce6–MnO2-mediated PDT, compared to 36% and 21% for the Ce6-GO and Ce6-MSN nanosystems, respectively. These findings showed that MnO2 nanosheets have a great deal of promise for raising PDT efficacy in a situation that is clinically relevant Fig. 14.208 Recently, PEG-cyclic arginine-glycineaspartic acid tripeptide (PEG-cRGD) was used to modify MnO2 nanosheets in another work by Zeng and coauthors. PS Ce6 was then coupled with PEG-cRGD with high loading efficacy. Because of the cRGD-mediated tumor-targeting capacity, the produced MnO2-PEG-cRGD/Ce6 nanocomplex dramatically raise the concentration of oxygen and was particularly taken up by PC3 cells. This led to the achievement of good therapeutic results in vitro following irradiation at 660 nm.211
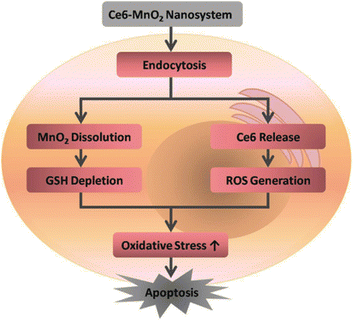 |
| Fig. 14 The activation mechanism of the Ce6–MnO2 nanosystem improves the photodynamic therapy efficiency (Fan et al., 2016).210 | |
4.1.6.2 2-Dimensional TMD nanosheets. TMD nanosheets have a large specific surface area, excellent biocompatibility, ease of customization, and extremely light and heat conversion efficiencies, making them an effective tool for tumor treatment and drug administration.212 In 2014, Liu et al. treated MoS2 nanosheets with lipoic acid-terminated PEG (LA-PEG) to develop a PEGylated MoS2-PEG nanosystem, which exhibited remarkable stability in physiological conditions. When compared to cells incubated with free Ce6, the cells treated with MoS2-PEG/Ce6 exhibited much greater intracellular Ce6 fluorescence, indicating a considerably improved PS Ce6 delivery effectiveness. In vitro tests also showed that MoS2-PEG/Ce6 had significantly higher PDT efficacy than free Ce6 in eliminating 4T1 cancer cells when exposed to 660 nm of light. This difference in efficacy may have resulted from the PS's enhanced cellular uptake, which increased the production of singlet oxygen inside the cells Fig. 15.213 The p-MoS2/n-rGO-MnO2-PEG nanocomposite was created by Kapri and colleagues employing p–n heterojunction to incorporate p-type MoS2 glycosheets onto n-type nitrogen-modified reduced GO. This was then organized using PEGylated modified MnO2 nanoparticles. When subjected to NIR light, the p–n heterojunction found in this nanosystem promotes electron and hole mobility, resulting in improved separation of electron–hole pairs and increased ROS generation via photocatalysis. Furthermore, MnO2 caused intracellular H2O2 to disproportionate, producing additional O2, which enhanced ROS generation even further. Studies conducted in vitro demonstrated that this nanocomposite has a potent PDT impact on HeLa cell death.214
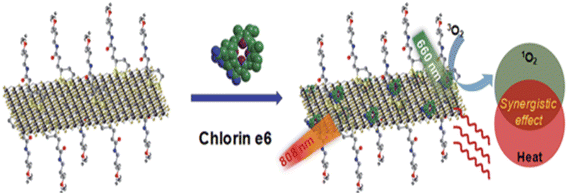 |
| Fig. 15 A schematic of MoS2-PEG/Ce6 nanosheets that enable Ce6 loading and combined PTT and PDT (Liu et al., 2014).213 | |
4.1.6.3 Graphitic-phase carbon nitride nanosheets. Due of its high biocompatibility, high stability, low toxicity, and high photoluminescence quantum yields, g-C3N4 nanosheets, emerging 2D nanomaterials have been applied in a number of biomedical applications.212 g-C3N4 nanosheets have been used as PS to create singlet oxygen, effectively killing HeLa cells following light revelation with a modest power density (20 mW cm−2) between 440–450 nm. Lin et al. published the first example of its application in PDT in 2014. Furthermore, because of their large surface-to-volume ratio, g-C3N4 nanosheets may operate as a carrier with a significant loading capacity of 18
200 mg g−1 when loaded with the anticancer medication DOX. Because DOX was more soluble and enhanced protonation in an acidic environment, its release was increased in an acidic pH environment. Furthermore, the g-C3N4 nanosheet's strong photoluminescence quantum yield made it feasible to utilize them for imaging, which allowed researchers to follow the delivery process Fig. 16.215
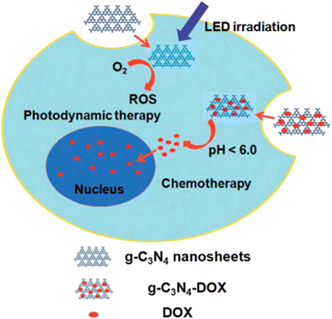 |
| Fig. 16 g-C3N4 nanosheets can function as photosensitizers as well as pH-responsive carriers of drugs for cancer therapy, as indicated in the schematic diagram (Lin et al., 2014).215 | |
Dai et al. recently used photo-excitation of the g-C3N4 solutions having spherical gold nanoparticles to cover the g-C3N4 nanosheets added to gold nanoparticles. Due to the improved electron/hole separation caused by the added gold nanoparticles, the absorption efficacy of 670 nm light may be improved, hence boosting the efficiency of 1O2 generation by photocatalytic water-splitting. The g-C3N4–AuNPs nanocomposites have shown good photodynamic therapy (PDT) results in inducing apoptosis or necrosis in three cancer cell lines (MCF-7, A549, and HeLa) that was test, when exposed to a 670 nm laser. Furthermore, in mice carrying MCF-7 tumors, efficient inhibition of tumor development has also been accomplished.216
4.1.6.4 Black phosphorus (BP). Because of its remarkable structural, optical, and chemical qualities, BP has developed as a unique kind of two-dimensional semiconductor that spurred a considerable lot of study in PDT after its first successful ablation from bulk to one or few layers of BP in 2014.216 Gold nanoparticles and Iron oxide (Fe3O4) were assembled on BP nanosheets using a standard electrostatic attraction technique to create a unique BPs@Au@Fe3O4 nanocomposite. This nanosystem demonstrated better therapeutic impact with superior targeting abilities by combining the PTT response of gold nanoparticles, cancer localization and MRI targeting efficiency of Fe3O4 nanoparticles, and the PDT effect of black phosphorus nanosheets. Moreover, the U14 tumor-bearing mice treated with BPs@Au@Fe3O4 at 650 nm light demonstrated a notable inhibition of tumor development. The potential applications of BP nanosheets in biomedicine were unveiled by this work Fig. 17.217
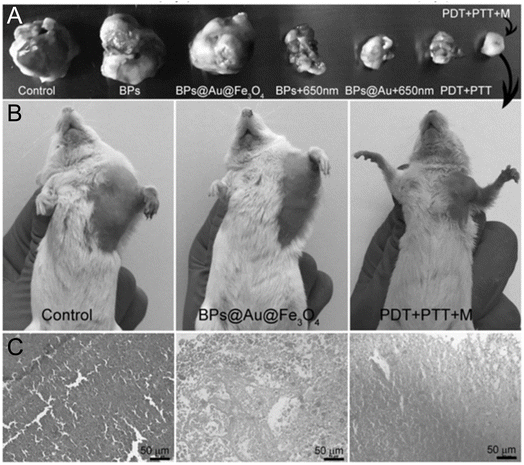 |
| Fig. 17 On day 14, (A) tumor-bearing animals treated normal saline, BPs, BPs@Au@Fe3O4, BPs and BPs@Au in 650 nm irradiation, BPs@Au@Fe3O4 with two stimuli, and BPs@Au@Fe3O4. (B) Photograph ordinary mice on day 14. (C) H&E-stained tumor slices from panel (C) groups (Yang et al., 2017).217 | |
In a different study, Liu and colleagues created a BP/MnO2 nanoplatform by electrostatically assembling fluorescein isothiocyanate (FITC)-designed FBP (BP with peptide modification) and Rhodamine B (RhB)-confined MnO2 (R-MnO2). R-MnO2 serves as the O2 supply and indicator in this R-MnO2-FBP nanocomposite. Endocytosis mediated by the target FA receptor (FR) can cause the production of O2 in an acidic and H2O2 rich atmosphere. Additionally, due to the emitted dyes RhB and Mn2+, fluorescence and magnetic resonance imaging (MRI) may be used to track the process of oxygen production. Both in, in vitro and in vivo, the FBP moiety could mediate very effective PDT in its capacity as the theranostic agent. Furthermore, by employing the activated caspase which is in the process of cell death brought on by oxygen-generating PDT, a self-feedback mechanism for the therapeutic response may be constructed.218
4.1.7. Nanoscale metal–organic frameworks (MOFs). Nanoscale metal–organic frameworks (NMOFs) have emerged as a potential delivery system for photodynamic therapy (PDT) owing to their diverse activities arising from their chemical compositions, unique crystalline structures, substantial porosity, and tunable framework stability. In a study by Meie He and his colleagues, they described a Mn-porphyrin based MOF made of biocompatible Zr4+ ions and Mn-porphyrin ligands. The Mn-porphyrin shown a strong catalytic capacity to convert H2O2 to O2, achieving oxygen self-supplementing PDT. Additionally, the special porous MOF frameworks may speed up the diffusion of produced O2 and H2O2 and inhibit Mn-porphyrin from self-aggregating Fig. 18.219,220
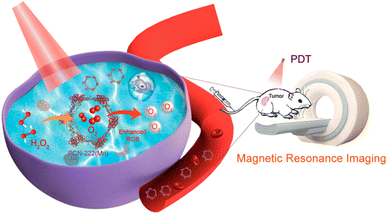 |
| Fig. 18 Illustration of PCN-222(Mn) for MRI-guided oxygen self-supporting photodynamic therapy (He et al., 2019).219 | |
4.1.8. Organic nano-agents. PDT has also made use of several newly created nano-agents, like porphysomes and nanostructured phthalocyanine self-assemblies Fig. 19.221 Li et al. 2017 reported on nanostructured phthalocyanine self-assemblies made of zinc(II) phthalocyanine building blocks decorated with triethylene glycol (TEG) as target molecules. The unique noncovalent interactions among the targeting agents, phthalocyanines, lead to the produced nanoassemblies demonstrating targeted protein-induced partial disintegration. The nano-phthalocyanine ensembles exhibit switchable photoactivity, facilitating the generation of reactive oxygen species (ROS) and enabling tumor-specific photodynamic treatment.222 In summary, a growing number of novel nanomaterials are predicted to arise as a result of the biomedical field's tremendous advancement in nanoscience over the past several decades, presenting promise for the development of viable alternative cancer therapy techniques.197
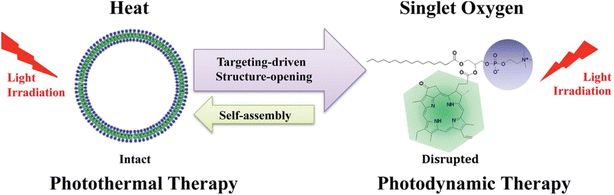 |
| Fig. 19 Porphysomes are targeted for photodynamic activation in this illustration (Jin et al., 2014).221 | |
4.1.9. Nanofibers. Localized cancer treatment is a very successful strategy for destroying solid tumors in early stages, minimizing adverse effects from cancer therapies. Electrospun nanofibers are a viable implantable platform for targeted cancer treatment. They allow for on-site delivery of therapeutic components while reducing negative effects on normal tissues. Paclitaxel was successfully loaded into electrospun PLGA nanofibers with over 90% efficiency. The study found that medication release can persist up to 60 days and kill up to 70% of C6 glioma cells within 72 hours.223 Severyukhina et al. studied photosensitizer-loaded electrospun fibers for photodynamic cancer treatment. Electrospinning was used to produce chitosan/PEO nanofibers containing various photosens. The physically adsorbed photosens were released with the swelling of the nanofibers. The breakdown of the nanofiber complex caused a delay in release. 675 nm laser irradiation dramatically reduced metabolic activity in scaffold-treated human mammary gland T-47D cancer cells, with no recovery. In addition, limiting the lighted region can reduce the phototoxic impact, leading to improved topical photodynamic cancer treatment.224Wu et al. found that electrospun PLLA nanofibers containing purpurin-18 were biocompatible. Human esophageal cancer ECA109 and human hepatocellular carcinoma SMMC 7721 cells interacted and integrated well with the surrounding fibers. MTT experiments showed that cancer cells were killed promptly after PDT with a 702 nm laser light.225 Costa et al. created core–shell electrospun nanofibers from biodegradable polymers such as poly(vinyl alcohol) (PVA) and gelatin (Gel) to function as a localized DDS for the treatment of cervical cancer with PDT. The synthesized porphyrin (Por) produced singlet oxygen (ΦΔ = 0.62) and exhibited stronger phototoxicity against tumor cells than healthy cells (Table 1). The Por release profile from nanofibers demonstrated an initial rapid release phase, followed by continual release for at least 9 days. PVA-Gel + Por core–shell nanofibers inhibited cancer cell growth more effectively under light irradiation than under dark irradiation, and they had a greater phototoxic impact on tumor cells than non-tumor cells.226
Table 1 Some of the nanoparticles studied for photodynamic therapy (PDT)
Type of nanoparticle |
Material composition |
In vivo and in vitro studies |
Advantage |
Reference |
Gold nanoparticles |
Gold nanorods |
B16F0 melanoma tumor in mice |
Single photon-induced fluorescence – for visualization in in vivo condition |
160 |
Gold and silver nanostructures |
HeLa cells |
Promising dual functional nanomaterials with capabilities of simultaneously serving as photodynamic therapy and photothermal therapy |
163 |
Silver nanoparticles |
Porphyrin-mercaptosuccinic acid-capped silver nanoparticles (POR-MSA-AgNPs) |
Cancer cell line – A375 |
Significant singlet oxygen generation efficiency and cell imaging |
165 |
ZnPcS4/Ag-PEG-FA and ZnPcS4/Ag@mSiO2-F |
A375 melanoma cancer cells |
Cell death induced via apoptosis rather than necrosis |
167 |
Silica based nanomaterials |
Mesoporous silica nanoparticle – SiNPs – 5,10,15,20-tetrakis (1-methyl 4-pyridinio) porphyrin tetra (p-toluenesulfonate) (TMPyP) (MSN@SiNPs@TMPyP-FA) |
Human breast carcinoma cell lines (MCF-7) and human lung cancer cell lines (A549) |
Targeted two-photon fluorescence cellular imaging at the near-infrared (NIR) laser excitation, this could effectively avoid the interference of biological auto-fluorescence |
176 |
Hollow silica nanoparticle (HNP) – with PDMAEMA polycation – chlorin e6 – caspase-8 gene (CSP8), (Ce6-HNP-Cou-PD/CSP8) |
Hepatocellular carcinoma cancer cell line (HepG2) and the human cervical cancer cell line (HeLa) |
Relatively high sensitizing efficiency, remarkable singlet oxygen generation efficiency, and rapid elimination ability from the body |
177 |
Quantum dots |
Hematoporphyrin (HP)-encapsulated carbon quantum dots (CQDs) |
MCF-7 cells |
Excellent solubility in water, high phototoxicity and low dark toxicity |
187 |
Carbon based nanomaterial |
MMC-Graphene@BODIPY-mPEG (MGBP) |
HeLa cell |
Excellent ROS production ability, high photothermal conversion efficiency (48%), and excellent therapy efficiency in vitro |
202 |
Graphene oxide (GO) coupled with magnetic Fe3O4 nanoparticles and chitosan (CS) (MCGO) |
Human hepatoma cell lines HepG-2 |
High stability, good water solubility and biocompatibility, expected magnetic targetability, and good photostability for PDT |
204 |
PEG 2000N modified Fe3O4@carbon quantum dots (CQDs) coated single-walled carbon nanotubes (SWNTs), SWCNTs-PEG-Fe3O4@CQDs |
HeLa cells |
Imaging-guided collaborative treatment of cancer |
193 |
Fullerene (C60) L-phenylalanine derivative attached with poly (lactic acid) (C60-phe-PLA) with mitoxantrone (MTX) |
C57BL mice |
High antitumor efficacy without toxic effects to normal organs, increased MTX tumor retention time, low MTX levels in normal organs and strong photodynamic activity |
194 |
Two dimensional nanomaterials |
MnO2 nanosystem – chlorin e6 (Ce6) |
MCF-7 breast cancer cells |
Efficiently deliver it into cells, inhibits extracellular singlet oxygen generation by Ce6, leading to fewer side effects |
210 |
PEGylated MoS2 (MoS2-PEG) |
4T1 cells |
Synergistic cancer killing with utilizing both PDT and PTT |
213 |
g-C3N4 nanosheets |
HeLa cells |
Ultrahigh drug-loading capacity, pH-responsive release property, visualization of the delivery |
215 |
4.2. Photothermal agents in photothermal therapy
Stimulation-responsive nano-systems are being proposed as a method of precisely controlling nanomedicine expression. Because tumors can be deep-rooted, it is difficult to activate nano-systems in lesions while avoiding overexpression in healthy tissues. Because of this limitation, a suitable illumination source is required for efficient PTT. NIR light is regarded as a suitable excitation source as low-power NIR rays may enter tissues deeply while avoiding major harm or absorption by bodily fluids.227,228 As a result, NIR-induced imaging and targeted therapy have lately received a great deal of interest. Because of its strong penetration through tissues, NIR fluorescence is currently used for diagnostics and labelling.229 Nanomaterials have many distinct characteristics than their macroscopic analogue. It is vital to highlight that the effect of photothermal energy is linked to the nanoscale characteristics of agents. For example, gold nanoparticles (NPs) may transform excited state photon energy to heat via surface plasmon resonance (SPR).230
Nanomedicines also provide a benefit in cancer treatment for passive tumor targeting by the mechanism of nanoparticle produced endothelial leakage (NanoEL) or enhanced EPR of nanoparticles for both immature and adult cancers, respectively. During leakage of endothelium, NPs of particular density and size react with the protein of adherens junction of endothelial cells, causing micro-sized gaps between them, boosting nanomedicine permeability and accumulation.231 The size, surface chemistry and shape associated with nanoparticles have a significant impact on their biocompatibility and thermal performance.232 Noble metal nanoparticles, being one of the earliest PTT ablation agents, have been extensively investigated in the past. Novel nanomaterials, including as nanoarchitectured conducting polymers, have been developed for PTT usage in recent years.233
4.3. Noble metal nanoparticles for photothermal treatment
Nanoparticles composed of noble metals, such as gold and platinum, have dominated research on PTT agents in the last few decades.234 Unlike organic dyes, which often cause quick photobleaching when used as ablation agents, noble metal nanoparticles have a reasonable photothermal interaction efficiency and a considerable optical absorption capacity.235 To convert light into heat, noble metal nanoparticles must have oscillations that resonantly match the practical frequency of the light.236
4.3.1. Gold nanoparticles. Noble metal nanomaterials have recently taken an attention in studies investigating the potential of gold nanoparticles for PTT. For instance, in the visible light spectrum, unaltered gold nanoparticles display SPR activity. It was illuminated by 20 ns bursts of 532 to 565 nm laser energy, according to Pitsillides et al. Short bursts of laser light were used to stop heat from diffusing, even though these wavelengths may not fall squarely inside the biological window.237 If the gold nanoparticles are large and irregularly shaped, the Mie theory predicts, the SPR effect will be strong, and the SPR wavelength will be moved into the near-infrared spectrum.238 To alter the SPR and enhance photothermal performance, many gold nanomaterials have been created, such as nanohexapods, nanorods (NRs), and nanoshells.239,240The length and diameter of the rod determine the optical absorptions in gold NRs, which are transverse and longitudinal, respectively. Additionally, the SPR zone may be shifted to the near-infrared (NIR) area for PTT by adjusting the aspect ratio. Link et al. looked at how aspect ratio relates to longitudinal plasmon resonance absorption maximum. Their research shows that whereas the transverse plasmon absorption of typical gold NP occurs in the visible light region, the longitudinal plasmon absorption moves to 740 nm when the average aspect ratio approaches 3.3.241 The prospective uses of dendritic gold nanoparticles in photothermal therapy are also being explored, since their properties vary according to the hyperbranched structures they possess. A correlation between the degree of branching and the photothermal characteristics of gold nanodendrites has been studied. The optical characteristics of gold nanodendrites were found to be changeable. In the second near-infrared region (Fig. 20), photothermal treatment efficiency was better for gold nanodendrites with fewer branches.242 Gold nanoparticles which have been created as drug transporters and image contrast agents, hinting that they might play a multifunctional role in medicine. A potential PTT ablation agent with further medical uses might be a hollow kind of gold nanomaterial, such a gold nanocage. In addition to their unique hollow nanostructure, which likely enables them to serve as drug carriers and contrast molecules for imaging, the hollow gold nanoparticles exhibit outstanding near-infrared absorption for photothermal therapy (PTT).243 Yavuz et al. modified gold nanocages by coating them with a polymer blend of poly(N-isopropylacrylamide) and polyacrylamide. In order to facilitate the circulation of medications contained inside the gold nanocages, a polymer coating goes through a phase shift and produces open holes when exposed to the photothermal activity of the nanocages.244 Nanoparticles of silica and iron oxide encased in gold have recently been suggested. These composites exhibited robust near-infrared optical absorption due to the nanostructure of the gold nanoshells.245
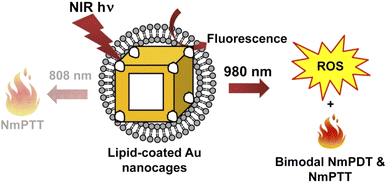 |
| Fig. 20 Photo-induced lipid-coated gold nanocages' working mechanism is illustrated schematically (Vankayala et al., 2014).240 | |
A group of scientists evaluated the effect of PEG-Cur-Au NPs on the C540 (B16/F10) cell line and implanted (bearing) melanoma tumors in inbred C57 mice after being exposed to an 808 nm laser. PEG-Cur-Au NPs exhibited dose-dependent cytotoxicity against the C540 (B16/F10) cell line at concentrations >25 μg mL−1, with an IC50 value of 42.7 μg mL−1 in dark (and no toxicity at 10 μg mL−1). For 10 minutes of 808 nm laser irradiation (without PEG-Cur-Au NPs), the C540 (B16/F10) cell line was killed in a laser power-dependent manner at power density >0.5 W cm−2 (no toxicity at 0.5 W cm−2). However, PTT employing PEG-Cur-Au NPs was extremely visible following laser illumination. Even at a power intensity of 0.5 W cm−2 of PEG-Cur-Au NPs concentrations <10 μg mL−1, the cells showed significant PTT.246
A group of scientists created hybrid albumin nanoparticles using AuNCs (∼88 nm) and AuNPs (∼4.5 nm). After 808 nm laser irradiation (1.5 W cm−2, 10 min), AuNCs/BSA-NPs significantly inhibited tumor development (17.8 ± 16.9 mm3 vs. PBS and AuNCs/BSA-NPs (formula E): ∼1850 and ∼1250 mm3, respectively).247 Wang et al. produced a multifunctional responsive drug carrier by loading resveratrol (Res) into chitosan (CTS) modified liposomes and coating them with gold nanoshells. The resulting GNS@CTS@Res-lips have a broad near-infrared (NIR) absorbance, high capability, stability, and photothermal conversion ability, making them suitable for effective photothermal treatment (PTT). In addition, the GNS@CTS@Res-lips have on-demand pH/photothermal-sensitive drug release and a high-Res loading capacity. The drug delivery device might greatly improve drug uptake by cells when exposed to NIR laser irradiation. More notably, compared to single chemotherapy or PTT, carriers with NIR irradiation had a greater therapeutic impact on HeLa cells.248
Xia et al. reported a simple method for fabricating gold nanostars (GNS) attached with matrix metalloproteinases (MMP2) polypeptides (Ac-GPLGIAGQ) and IR-780 iodide via bovine serum albumin (BSA) for targeted dual-modal photoacoustic (PA)/near-infrared (NIR) fluorescence imaging and enhanced photothermal therapy (PTT)/photodynamic therapy (PDT) for lung cancer. MMP2 polypeptides were used as a targeting ligand, IR-780 iodide as an NIR fluorescence imaging and PTT/PDT agent, and GNS as a carrier of IR-780 molecules for PA imaging and PTT. In vitro investigations confirmed that GNS@BSA/I-MMP2 nanoparticles (NPs) were successfully absorbed by A549 cancer cells, resulting in exceptional anticancer activity. GNS@BSA/I-MMP2 NPs could selectively target the tumor and greatly inhibit tumor development, and their anticancer effects were mostly due to the synergistic effects of PDT and PTT based on IR-780 and GNS.249
4.3.2. Semiconductor nanomaterials used in PTT. Due to their affordability and cytotoxicity, a number of semiconductor nanomaterials, including semiconductor copper chalcogenide nanomaterials, have drawn interest as PTT ablation agents in addition to noble metal nanoparticles.250 Nevertheless, under standard NIR light at around 808 nm, the relatively poor photothermal interaction efficiency of certain copper-based nanomaterials may need increased NIR power to facilitate tumor ablation. To overcome this challenge, NIR light at 980 nm is frequently used as a replacement for certain Cu-based NPs. This light is a rather high threshold for exposure to human skin, with penetration across biological tissues potentially reaching several centimeters. With this regard, the strength of the NIR laser might be lowered while the treatment's effectiveness was maintained.251 Hu's group successfully synthesized CuS nanoparticles exhibiting a consistent three-dimensional floral shape, enhancing photothermal efficiency. It was suggested that these superstructures may serve as laser-cavity mirrors for a 980 nm laser, enhancing reflectivity and hence photothermal efficiency. They also synthesized hydrophilic Cu9S5 plate-like nanocrystals with a photothermal efficiency of up to 25.7%, surpassing that of gold nanorods under analogous 980 nm light irradiation.252CuS NPs are being employed in studies on the detrimental impact of PTT upon HeLa cells. The dependent impact of laser dosage and CuS concentration of particles was then investigated. In this study, an NIR laser (808 nm) with a power of up to 24 W cm−2 was used to accomplish relatively selective cancer treatment. The NIR laser as well as CuS NPs alone had a moderate effect on cell death. Furthermore, the minimal cytotoxicity of this NP was demonstrated individually in this study, meaning that copper sulfide NP-induced PTT was harmless to normal cells. Moreover, modified Cu7.2S4 nanocrystals with photothermal efficiencies as high as 56.7% were developed. These Cu7.2S4 nanocrystals effectively eradicated cancer cells using a low dose concentration and a diminished power 980 nm laser. CuS nanoparticles has the potential to serve as an exceptional ablation agent for photothermal therapy due to their targeted ablation effect, low cytotoxicity, and cost-effectiveness.253
4.3.3. Carbon-based nanomaterials used in PTT. CNTs have recently been investigated in the medical area for application in thermal therapy due to their optical characteristics and outstanding thermal behavior. CNTs have been used in medical applications such as medication transporters and bioimaging probes. They can be covalently or noncovalently treated with various chemical groups. CNTs may readily be functionalized with other medicinal molecules, such as magnetic NPs and anti-cancer medicines, by binding or wrapping. This might increase the photothermal efficiency of CNTs and allow for the development of synergic medicines.254 PEG coatings often enhance biocompatibility, inhibit aggregation, and prolong blood circulation duration, all of which are considered advantageous in nanoparticles used as ablation agents.255 Burke et al. examined the response of BCSCs to hyperthermia by a water bath or the photothermal effect induced by MWNTs to elucidate the PTT mechanism mediated by CNTs. BCSCs exhibited resistance to hyperthermia throughout a broad temperature spectrum; yet, the photothermal action induced by MWCNTs may surmount this resistance by enhancing necrotic cell death. The hypothesis was formulated based on the interaction between cancer cell membranes and NIR-stimulated MWNTs exhibiting increased surface temperatures.256Graphene-based nanomaterials, just like CNTs, have a substantial optical absorption in the near infrared area along with high surface activity, which makes them a prospective PTT ablation agent with synergic therapeutic applications Fig. 21.257 Markovic et al. examined the sort of cell death caused by graphene-induced PTT. Based to their results, cells perished through combination of both necrosis and apoptosis. Though PTT causes necrosis, heat would increase oxidative stress/superoxide generation and, eventually, apoptosis.258
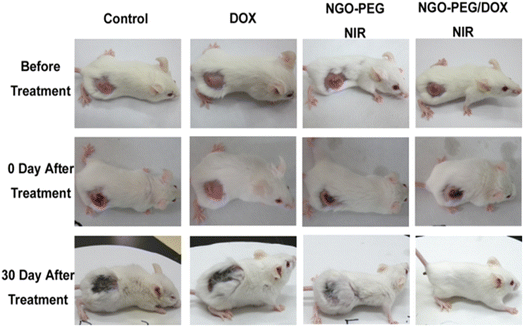 |
| Fig. 21 Representative photographs of tumours on mice after different therapies are shown. The laser irradiated tumors on the NGO-PEG-DOX-injected mouse was fully destroyed (Zhang et al., 2011).257 | |
Lim et al. (2018) created a ∼155 nm nanocomposite containing GO, folic acid, and manganese dioxide (MnO2). In cancer, MnO2 decomposes hydrogen peroxide into oxygen, alleviating hypoxia. The results demonstrate that the composite has a higher heat capacity than a single GO. Under 808 nm laser illumination for 3.5 minutes, the nanocomposite reaches the target temperature of 47 °C, whereas GO only reaches 35 °C.259 Xie et al. (2019) created a composite with high stability and dispersibility using GO, magnetic nanoparticles (Fe3O4), chitosan, sodium alginate, and doxorubicin hydrochloride. They tested the composite PTT qualities using an MTT assay using a human lung cancer cell line (A549) and 808 nm irradiation for 5 minutes, exhibiting good intracellular uptake characteristics and a temperature-dependent rise in concentration. The dosage of 100 μg mL−1 resulted in a 14.36% drop-in survival rate.260 Gulzar et al. (2018) created a combination of GO, amino-modified upconversion nanoparticles (NaGdF4:Yb3+/Er3+@NaGdF4:Nd3+/Yb3+), polyethylene glycol (PEG), and chlorin e6 (Ce6). Singlet oxygen production was confirmed using the DPBF (1,3-diphenyliso-benzofuran) chemical probe (PDT effect). In addition, an in vivo anticancer property was assessed in mice utilizing an U14 (murine hepatocarcinoma) cell line and 808 nm irradiation. As a result, the fluctuation in the relative volume of the tumor (V/Vo) was decreased by half after 14 days as a result of the PTT effect, while this value in the control group rose by ninefold.261
De Paula et al. (2020) used red LED (640 nm) ablation to drastically reduce the tumor mass in mice (melanoma in B16F10 lineage cells) with a rGO-based therapy. The tumor volume was around 70 mm3 on the first day, but decreased to around 40 mm3 after 8 days of therapy. Furthermore, the immunological response was validated by measuring the development of CD8+ T cells.262 Zhang et al. treated A549 lung cancer cells with two distinct light sources, 808 nm and 450 nm, to PTT and PDT, respectively. They employed an r-GO composite with a PEG-modified Ru(II) complex as the PS. This combination improved cytotoxicity and reduced tumor volume, as demonstrated by in vivo experiments. The PTT-PDT therapy slows tumor development, lowering the relative tumor volume value (V/Vo) to near-zero. In contrast, PTT and PDT alone increased this value to around 1.5 and 2.5, respectively.201
Marangon et al. developed a nanosystem based on multi-walled carbon nanotubes (MWCNT) and the photosensitizer m-tetrahydroxyphenylchlorin (mTHPC) for cancer treatment using photodynamic (PDT) and photothermal (PTT) therapy. The photothermal and photodynamic cytotoxicity of these mTHPC/MWCNT on/off complexes was evaluated at the cell level using viability tests, imaging flow cytometry, confocal microscopy, and transmission electron microscopy, as well as at the molecular level using a proteomic analysis of apoptosis-related proteins and a genomic analysis of 84 oxidative stress genes. At the cell level, cytotoxicity was associated with mTHPC/MWCNT absorption, whereas PDT and PTT treatment generated distinct signaling pathways that led to cell death. For the first time, the mechanisms of PDT/PTT synergy in cancer cell eradication were investigated, indicating that different cell responses to PDT and PTT undermine the cell's oxidative stress defense.263
4.3.4. Conducting polymers used in PTT. PEG coatings often enhance biocompatibility, inhibit aggregation, and prolong blood circulation duration, all of which are considered advantageous for nanoparticles used as ablation agents.255 Burke et al. examined the response of BCSCs to hyperthermia by a water bath or the photothermal effect induced by MWNTs to elucidate the PTT mechanism mediated by CNTs. BCSCs exhibited resistance to hyperthermia throughout a broad temperature spectrum; yet, the photothermal impact induced by MWCNTs might potentially surpass this resistance by enhancing necrotic cell death. The hypothesis was formulated based on the interaction between cancer cell membranes and NIR-stimulated MWNTs exhibiting increased surface temperatures.256A multitude of nanoparticles has been included into photothermal therapy (PTT), signifying substantial promise for this focused and less deleterious cancer treatment. Although the experiments remain in preliminary phases, several biological investigations on PTT have shown encouraging outcomes. Despite the variability in the processes of various ablation agents, it was shown that the effects of photothermal radiation could generally be regulated by modifying the shape and dimensions of these PTT agents (Fig. 22). Given the multitude of potential alternatives and ablation chemicals for photothermal therapy (PTT), together with the use of nanotechnology, researchers may manufacture a molecule exhibiting enhanced photothermal effectiveness for PTT.33
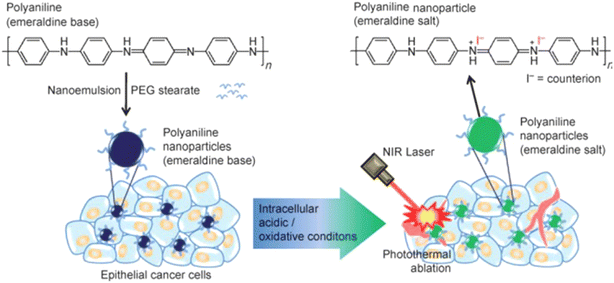 |
| Fig. 22 Scheme for producing naturally occurring photothermal agents using polyaniline nanoparticles and using NIR laser irradiation to kill epithelial cancer cells (Yang et al., 2011).264 | |
4.3.5. Tungsten based nanomaterial. Zhou et al. developed a simple thermal decomposition method to produce tungsten oxide nanorods (WO2.9 NRs) measuring 13.1 ± 3.6 nm in length and 4.4 ± 1.5 nm in diameter for tumor theranostic applications. The generated WO2.9 NRs were treated with methoxypoly(ethylene glycol) (PEG) carboxyl acid by ligand exchange to improve water dispersibility and biocompatibility. PEGylated WO2.9 NRs exhibit high photothermal conversion efficiency and superior X-ray attenuation compared to clinically used CT contrast agent Iohexol. They effectively inhibit cancer cell growth in vitro and tumor growth in vivo, allowing for effective CT imaging.265Sharker and his colleagues reported a dopamine-conjugated hyaluronic acid (HA-D), a mussel-inspired simple capping material that can make tungsten oxide (WO3) nanoparticles biocompatible and targetable, enabling for precision delivery (WO3-HA) to a tumor location. Near-infrared (NIR) irradiation WO3-HA demonstrated a quick and significant increase in photothermal heat, resulting in full in vitro thermolysis of malignant MDAMB and A549 cancer cells, but was shown to be less susceptible to normal MDCK cells. Long-term in vivo study of WO3-HA nanoparticles with ∼10 nm HA thickness showed effective photo-thermal conversion and time-dependent tumor target accumulation. This long-term in vivo survival study of WO3-HA demonstrated good biocompatibility, with full recovery from malignant tumors.266
4.3.6. Nanofibers. In recent years, life scientists have focused on precise spatiotemporal regulation of physiologically important species delivery. Light, particularly near-infrared (NIR) light, inside the “transparency window” for biological tissues, is an elegant on/off stimulus that meets these characteristics.267 Cheng et al. introduced PEG-modified gold nanorods (PEGGNRs) into an electrospun PLGA/PLA-b-PEG membrane. This platform's polymer membrane provided both a physical barrier against surgical damage and a biocompatible carrier for PEG-GNRs. When incubated with cancer cells, the PEG-GNRs were released from the fibrous mat and eventually absorbed by the cells. PEG-GNRs released under 850 nm NIR irradiation generate heat. An in vitro investigation found that the PEG-GNR-incorporated mesh may selectively kill cancer cells and limit their growth.268 In the work by Wang, doxorubicin (DOX) and indocyanine green (ICG) co-loaded mesoporous silica nanoparticles (DIMSN) were produced. The nanoparticles were then electrospun into chitosan/poly(vinyl alcohol) (CS/PVA), resulting in multifunctional composite nanofibers (DIMSN/F). Under the mimic erosion of vaginal discharge, DIMSN/F demonstrated site-specific drug release, however local administration of a thermosensitive DIMSN-loaded gel (DIMSN/gel) did not. When compared to systematic DIMSN injection, vaginal implantation of DIMSN/F might optimize drug accumulation in mice's vaginas. DIMSN/F photothermalchemotherapy (PTCT) effects were investigated in both subcutaneous and orthotopic cervical cancer models in mice, although drug penetration in the hard nodular tumor offered a significant barrier. The tumor inhibition rate (TIR) for orthotopic cervical/vaginal cancer remained as high as 72.5%, indicating a good promise for cervical cancer therapy.269 Paclitaxel, an anticancer medication, and graphene oxide/gold nanorods (GO/Au NRs) were loaded into poly (tetramethylene ether) glycol-based polyurethane (PTMG-PU) (core)/chitosan (shell) nanofibers generated by coaxial electrospinning. The potential of the produced nanofiber as a pH/temperature dual responsive carrier was examined for the controlled release of paclitaxel against A549 lung cancer using the PTT/CHT combination technique. The cell survival of manufactured nanofibers treated with A549 lung cancer cells was studied using the alone CHT, alone PTT, and PTT/CHT methods. In vivo investigations suggested that the PTT/CHT approach displayed an optimum therapeutic impact on tumor inhibition without changing body weight.270
5. Stimuli responsive nanomaterials
Although their clinical applications are still in the early stages, as compared to traditional chemotherapy, multiple significant pre-clinical investigations have indicated that stimuli responsive techniques provide superior therapeutic success with fewer adverse effects.271,272 Specific triggers/stimuli can be roughly classed as intrinsic or external stimuli. Intrinsic stimuli are limited to the interior of the body/organism system, with the tumor microenvironment serving as an excellent example of creating local stimuli such as pH or interstitial pressure, among other things. A collection of characteristics known as ‘external-stimuli’ exist in addition to the host's internal system and include magnetic fields, ultrasound, light, and so on.273 Light-responsive drug release systems have been developed using methods such as photoisomerization, photocrosslinking/decrosslinking, photosensitised oxidation, light-triggered polarity flipping, and photo- or photodegradation of the polymer backbone.274 Cholesteryl succinyl silane (CSS) nanomicelles encapsulating DOX, Fe3O4 magnetic NPs, and Au nanoshells were created as a multicomponent/functional drug delivery system. These composite nanomicelles show an 808 nm NIR laser-induced temperature rise, which causes progressive DOX release. These CSS nanomicelles have a high Tm value, requiring a high temperature to destabilize them (Table 2). This is especially useful when considering the on-demand release of DOX only after NIR irradiation of the Au nanoshells, which raises the local temperature, loosens the micelles, and effects drug release, hence reducing undesirable drug leakage throughout circulation.285
Table 2 Some of the nanoparticles studied for photothermal therapy (PTT)
Type of nanoparticle |
Nanoparticle studied |
Irradiation conditions |
Temperature reached upon irradiation |
Cancer studied in vivo |
Advantage of nanocomposite |
Study outcome |
Ref |
Gold nanosphere |
Gold nanocluster-loaded hybrid albumin nanoparticles |
808 nm, 1.5 W cm−2 for 10 min |
70 °C |
HCT116 tumor |
Facilitate an increase in temperature up |
A decrease in size of tumor from 150 mm3 to 17.8 mm3 |
247 |
Gold nanoshell coated thermo-pH dual responsive liposomes for resveratrol delivery |
808 nm, 2 W cm−2 for 5 min |
66.7 °C |
HeLa cells |
The photothermal effect created by such nanomaterials remained consistent over 5 cycles of irradiation under a NIR laser |
HeLa cell viability is reduced by up to 57.3% |
248 |
Anti-EGFR antibody linked thiol chitosan-layered gold nanoshells |
808, 1.2 W cm−2 for 5 min |
61.9 °C |
MDA-MB-231 cells |
The produced heat might be used to stimulate the release of paclitaxel from the chitosan layer on the outermost layer of the gold nanoshells |
97.43% tumor inhibition rate |
275 |
Gold nanorods |
DNA-conjugated gold nanorods |
808 nm, 5 W cm−2 for 30 min |
45 °C |
MCF-7/ADR cells |
Strong photothermal effect. The gold nanorods have a pH and NIR sensitive drug release due to DOX intercalation alongside the DNA coated on the particles surface. When irradiated with an NIR laser, around 60% of the drug is released |
81% inhibition of MCF-7/ADR cell proliferation was observed |
276 |
Gold nanostars |
Matrix metallopeptidase 2 targeted delivery of gold nanostars decorated with IR-780 iodide |
808 nm, 0.8 W cm−2 for 5 min |
63 °C |
A549 cells |
Strong absorption band |
93% reduction of the tumor volume |
249 |
Gold nanocages |
Folic acid-functionalized gold nanocages for the targeted delivery of anti-miR-181b |
808 nm, 1.25 W cm−2 for 10 min |
55 °C |
SMMC-7721 |
Targeted delivery |
Reduced the growth of liver cancers and raised mice's median survival periods from 36 to 60 days |
277 |
Carbon nanotubes |
Photosensitizer/carbon nanotube complexes |
808 nm, 2.3 W cm−2, 200 seconds |
50 °C |
SKOV3 cancer cells |
The cytotoxic impact of nanotubes was enhanced by mixing them with m-tetrahydroxyphenylchlorin |
Only 10% of the SKOV3 cells remained alive |
263 |
Doxorubicin-loaded and folic acid-conjugated carbon nanotubes@ poly (N-vinyl pyrrole) |
808 nm, 1.5 W cm−2 for 6 min |
45 °C |
HeLa cells |
The capability of producing same level of increased temperature was maintained even after 5 cycles |
Only around 20% of the cells remained alive |
278 |
Graphene |
Hyaluronic acid functionalized green reduced graphene oxide |
808 nm, 1.7 W cm−2 for 5 min |
33 °C |
MCF-7 cancer cells |
The produced nanomaterial has a mean size of 108 nm and an NIR absorbance higher than that of graphene oxide |
Cell viability to ≈6% |
279 |
Poly (allylamine hydrochloride)-functionalized reduced graphene oxide |
808 nm, 6 W cm−2 for 6 min |
45 °C |
MCF-7 cells |
Broad absorption |
6% of viable cells was observed |
280 |
Tungsten |
PEGylated nonstoichiometric WO2.9 nanorods |
980 nm, 0.25 W cm−2 for 10 min |
20.1 °C |
HeLa cells |
Biocompatible |
Less than 20% cell viability |
265 |
Dopamine-conjugated HA tungsten oxide nanoparticles |
808 nm laser, 2 W cm−2 for 5 min |
44 °C |
MDA-MB-231 and A549 |
Broad absorption |
Less than 3% cell viability |
266 |
Molybdenum disulfide |
Molybdenum disulfide modified with hyperbranched polyglycidyl |
808 nm, 2 W cm−2 for 10 min |
34 °C |
B16 cells |
Increased absorbance in NIR region |
Cell viability to less than 30% |
281 |
Molybdenum oxide |
PEGylated plasmonic MoO3–x hollow nanospheres |
808, 1 W cm−2 for 10 min |
48 °C |
Hela cells |
Strong absorption band |
Almost total tumor elimination with no recurrences by day 15 |
282 |
Iron oxide |
Superparamagnetic iron oxide nanoparticles loaded with HA |
808 nm, 1 W cm−2 for 9 min |
18 °C |
MDA-MB-231 cells |
— |
≈70% decrease of the cellular viability |
283 |
Copper sulfide |
Hollow porous copper sulfide nanoparticles filled with artesunate and modified with transferrin |
808 nm, 2 W cm−2 for 5 min |
40 °C |
MCF-7 cells |
Iron-dependent artesunate action |
Death of 92.6% of cells |
284 |
Thermal ablation is becoming one of the most often reported forms of solid tumor treatment, and super paramagnetic iron oxide NPs (SPIONs) have been intensively studied in this area. HT treatment with iron oxide NPs (IONPs) entails administering an IONPs fluid to the tumor, followed by AMF application, which causes NPs heating and ablation. A temperature change of 41–46 °C can cause significant consequences on cells and tissues, including increased heat-shock protein production, protein denaturation/folding, and apoptosis. Specifically in tissues, the temperature surge stimulates pH alteration, perfusion, and oxygenation of the tumor microenvironment, with persistently elevated temperatures leading to necrosis.286–288 Matsumine et al. used HT in patients with metastatic bone tumors. Following the first surgical surgery, a biocompatible bone replacement composed of ‘Bare’ magnetite NPs and calcium phosphate cement was implanted. Patients received a 15 minutes treatment every alternate day commencing on the eighth day after surgery. The results showed a 32% reduction in lesions with apparent bone growth, 64% without any progressing lesions for more than 3 months, and only 4% with a negative treatment response.289
Longer wavelength radiofrequency (10 kHz to 900 MHz) can also be used for cancer cell ablation via the HT effect. RF offers more tissue penetration than NIR light, allowing for the therapy of deep-seated cancers.290 Elsherbini et al. used Au-coated magnetics for dual-mode HT against subcutaneous Ehrlich cancer in mice using laser and radiofrequency irradiation. Results analysis indicated that more than half of the tumors totally vanished after light/RF irradiation.291
6. Photodynamic combination therapy in cancer treatment
PDT includes activating a photosensitizer with a certain wavelength of light, resulting in transitory amounts of ROS. However, the use of PDT targeting deep tumors has been severely limited due to inadequate luminous flux and possibility of peripheral damage to tissues. As a result, researchers have begun to investigate if combining PDT with other therapies might increase its efficacy.292
6.1. PDT in conjunction with chemotherapy
Chemotherapy, which is among the most common cancer treatment procedures, is used to manage a wide range of cancers. Chemotherapeutic medicines are thought to attach to tumor cell's DNA, inhibiting cell proliferation and replication, ultimately leading to cancer cell death.293 Chemotherapy has the capacity to kill cancer cells, but its non-specific nature and susceptibility to resistance restrict its therapeutic usage. Several efforts have been undertaken to integrate PDT with chemotherapy in order to overcome the side effects and resistance and boost the therapeutic benefit. PDT coupled with chemotherapy may have a synergistic anti-tumor impact, reducing the therapeutic dosage of the chemotherapeutic medicines.292 Numerous studies have shown that integrating PDT with chemotherapy may enhance treatment efficacy, while the reasons behind this augmentation are uncertain.294 Xiaojun Wang discovered that employing the 1O2− reactive nano-carrier NOP-DOX@BSA-FA as a delivery strategy in PDT combined chemotherapy allows DOX to swiftly reach tumor regions, successfully destroying cancer cells and decreasing chemotherapy's harmful effects on the body.295
Employing PDT along with chemotherapy may overcome multi-drug resistance (MDR) caused by tumor treatment. MDR is often caused by overexpression of P-glycoprotein on tumor cells, which can be triggered by short-term chemotherapy treatment.292 Khdair discovered that combining methylene blue mediated PDT with adriamycin resulted in substantial toxicity against MDR tumor cells. This was due to a high concentration of doxorubicin in the MDR following PDT. Drug-resistant tumor cells undergo necrosis or apoptosis as P-glycoprotein expression decreases and ROS levels increase in the tissue.296 Bano found that coupling doxorubicin (DOX) along with nickel oxide nanoparticles (NOPs) with the form of NOP-DOX@BSA-FA, a potential PDT agent, resulted in a higher rate of cell death than utilizing NOPs alone Fig. 23.297
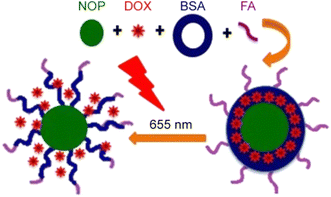 |
| Fig. 23 Mechanism of NOP-DOX@BSA-FA in PDT (Bano et al., 2016).297 | |
6.2. PDT in conjunction with radiotherapy
Local tumor irradiation using X-rays constitutes one of the most effective anti-tumor treatments. Approximately 60–70% of individuals with malignant malignancies require radiation therapy. Radiotherapy for advanced cancer patients can improve symptoms, reduce discomfort, and boost survival rates.292 Non-specific radiotherapy can cause harm to normal tissue in the radiation field. Furthermore, hypoxia cells in tumor tissues may be resistant to radiation. For individuals with advanced cancers, PDT coupled radiation can considerably enhance quality of life, reduce symptoms, decrease pain, and increase survival.298 Yi-shan Wang studied the effectiveness of PDT in combination with radiation therapy in 90 incidents of gastric cancer, 12 incidents of esophageal cancer, 24 incidents of rectal cancer, 8 incidents of bladder cancer, 6 incidents of cervical cancer, and 8 incidents of superficial tumors. The study found that combining PDT with intensity-modulated radiation (IMRT) improved the quality of life for patients with advanced malignant tumors, particularly those with cavity viscera. Combination treatment promotes palliative care for individuals with malignant tumors who have failed radiation and chemotherapy due to obstructive symptoms. Studies have indicated that combining PDT with radiotherapy might increase tumor susceptibility to irradiation and improve therapeutic efficacy. At the identical time, it can cut the exposure period or lower the radiation dosage.299
6.3. PDT in conjunction with immunotherapy
PDT-induced immune response is critical for avoiding tumor spread and recurrence. Designing PDT drugs specific to the immunological target can improve its anti-tumor impact while minimizing immune response suppression.292 Yuanhong Zheng discovered that tumor cells adjust to immunological pressure and demonstrate improved tumorigenic and stemlike characteristics following PDT immunization.292 Hisataka Kobayashi employed a photosensitizer coupled to MAbs binding epidermal growth factor receptors (EGFRs) for deeper tissue infiltration and selective targeting, resulting in tumor elimination Fig. 24.300 When paired with checkpoint inhibitors (PD-L1/PD1), PDT induces a robust tumor-specific immune response, this results in a reduction of both light-irradiated original tumors and non-irradiated distant malignancies.301 Laser immunotherapy (LIT) is a new approach that combines PDT with an immunological adjuvant. Recent research has shown that indocyanine green and glycated chitosan, when combined with PDT, have superior therapeutic results than PDT alone. Furthermore, GC has a better immune stimulating power than some other commonly used immune adjuvants.302,303 Utilizing PDT along with an immunological adjuvant can boost its efficiency. Korbelik discovered that combining PDT with mycobacterium extract (MCWE) significantly improves immune cell activation.304
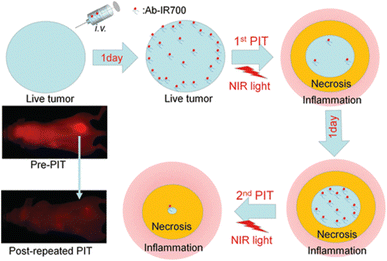 |
| Fig. 24 Fractionated dosing of mAb-IR700 conjugate and NIR light (Mitsunaga et al., 2012).300 | |
7. Photothermal combination therapy in cancer treatment
PTT offers several advantages, including good temporospatial control, cheap cost, and little invasiveness. This can also be employed alone to eliminate the underlying tumor or lymph metastases in superficial tissues. But illumination has a limit on penetrating depth. Even while NIR light are able to penetrate deep than UV as well as visible light, but can reach only a depth of around 3 cm in tissue.305 Due to the limited penetration into tissue at NIR light in deep layers of tissues, it is difficult for PTT alone to eliminate metastatic cancer cells and metastatic nodules in organs that are far away. As a result, in order to achieve the desired effectiveness against cancer metastasis, PTT must be combined with already known medicines.7
7.1. PTT in conjunction with chemotherapy
Multiple studies have revealed that CHT causes medication resistance, intrinsic cytotoxicity to normal cells, and distinct patient behavioral problems.32 Synergistic treatment with PTT may address these issues. When combined with nanocarriers, these agents may deliver CHT drugs to tumors without exposing normal cells, reducing drug dosage and perhaps reducing side effects and chemotherapy-resistant cancer cell growth. It takes less medicine to have the intended effect since drug release is slow without NIR irradiation and fast when photothermal agents are activated.306 If the dispensing mechanism is sufficient, turning off NIR laser may halt smart drug-releasing nanoparticles. Meng et al. created a smart G-CuS-DOX MEO2MA@MEO2 MA-co-OEGMA composite. PTT agents were CuS nanoparticles, CHT medications were DOX, and thermosensitive nanogel was MEO2MA@MEO2MA-co-OEGMA (G). Nanocapsule hyperthermia shrank nanogels and released DOX under NIR irradiation. Turning off NIR irradiation eliminated PTT and CHT effects. Combined PTT and CHT showed controllable and efficient advantages in vivo Fig. 25.307 Liu and Wang created hydrogel-based nanoplatforms for PTT and CHT tumor therapy. The chemical was injected into malignant tissue at room temperature, and the hydrogel formed instantly at 37 °C. Hydrogel matrix controlled chemotherapeutic drug release and had photothermal effects. They found that the composite hydrogel was an efficient tumor therapeutic platform.308,309
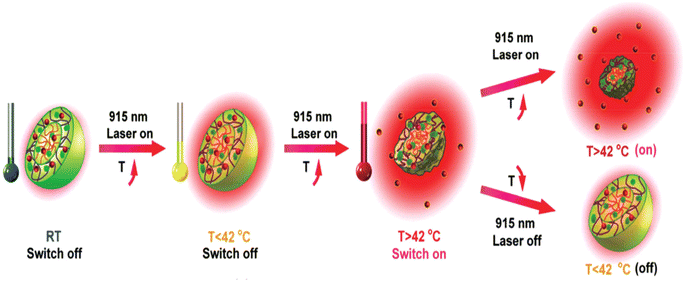 |
| Fig. 25 An ex vivo NIR laser switches the intelligent DOX release “on” or “off” (Meng et al., 2016).307 | |
7.2. PTT in conjunction with radiotherapy
Anticancer medications are unable to reach tumors because of the abnormal microenvironment that surrounds them. Nanoparticles face many obstacles on their way to the tumor site, such as growth-induced solid stress, complex tumor vascular networks, increased interstitial fluid pressure, and tangled interstitial structures.310 Arterial perfusion, vascular permeability, and interstitial fluid pressure are all positively impacted by malignancies that have had radiation treatment.311 Increased tumor perfusion, vascular permeability, and nanoparticle absorption are equivalent outcomes of mild hyperthermia produced by PTT.312 The penetrating depth of NIR light is limited, but X-ray and γ-ray irradiation do not have such limitations. However, PTT successfully kills hypoxic cancer cells due to hyperthermia and may improve oxygenation situation in the tumor, but RT has poor therapeutic efficacy for these cells.313 Accordingly, the therapeutic efficacy of both PTT and RT may be enhanced when administered together.36 In order to provide both PTT and RT treatments at the same time, Cheng et al. modified FeSe2/Bi2Se3 nanosheets with PEG. Important for RT and PTT, respectively, are the intrinsic properties of FeSe2/Bi2Se3-PEG, such as high NIR absorption and substantial X-ray attenuation. In addition, the composite nanostructure might be used as a contrast agent for in vivo computer tomography, photoacoustic (PA), positron emission tomography (PET) tetra-modal imaging, magnetic resonance (MR), and radioisotope 64Cu tagging. According to a study.314 FeSe2/Bi2Se3-PEG effectively killed tumors and inhibited their development. Yi et al. created PEG-improvised, iodine-131-doped CS (CuS/[131I]I-PEG) nanomaterials for PTT and RT. This nanomaterial employed high NIR absorption for PTT, but doped 131I-radioactivity in internal CT, which differed from the exterior CT used by previous two nanomaterials. Yi's research found that CuS/[131I] I-PEG had excellent synergistic therapeutic effectiveness in both in vitro as well as in in vivo trials. Importantly, when combined PTT and RT are administered to lymph nodes to aid in the surgical excision of primary tumors, they can limit cancer spread and prolong animal survival Fig. 26.315
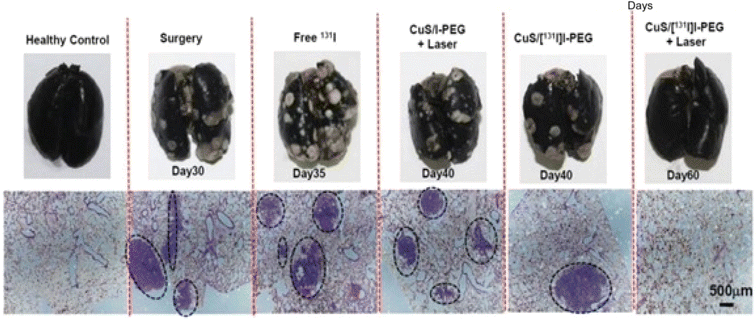 |
| Fig. 26 Photographs of complete lungs stained with India ink and micrographs of H&E-stained lung slices from various mouse groups. Dashed circles highlight tumor metastatic locations (Yi et al., 2015).315 | |
7.3. PTT in conjunction with immunotherapy
In comparison with gold-standard cancer therapies such as surgery, chemotherapy and radiotherapy, immunotherapy is a newer treatment that trains or stimulates the host's immune systems to eliminate tumor cells.316 IT has demonstrated amazing potential, but it still has significant limitations, including expensive, immunotoxicity, and wide individual variance.134 PTT-induced hyperthermia can kill cancer cells directly while also inducing the liberation of tumor-associated antigens, that are required for antigen processing and presentation. If immunotherapy and PTT are conjugated into a single therapy, IT may have a higher therapeutic efficacy against tumors due to the released antigens and immune adjuvants.317 Tao et al. used PEG and polyethyleneimine (PEI) dual polymer-operationalized graphene oxide (GO) as a carrier to transport CpG. GOPEG-PEI-CpG nanocomplexes have been shown to significantly increase proinflammatory cytokine production and immunostimulatory activity when exposed to NIR light. Furthermore, immunological responses are significantly boosted because GO's photothermal action promotes intracellular transport of CpG ODNs Fig. 27.318
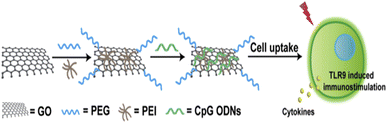 |
| Fig. 27 Schematic depicting the production of GGIC and its immunostimulatory function (Tao et al., 2014).318 | |
7.4. PTT in conjunction with PDT
PTT and PDT are both light responsive nanomaterial-based therapies, however PTT kills cancer cells by hyperthermia, whilst PDT kills tumor cells through reactive oxygen species (ROS). Despite their distinct treatment processes, PTT and PDT share comparable light triggering circumstances. Thus, photothermal and photodynamic therapy can function separately and in tandem under identical NIR laser irradiation.319 Tham et al. described a new nanomaterial zinc phthalocyanine (ZnPc) embedded silica-coated gold nanorods (AuNRs) for combinations of PDT and PTT. Imbedded ZnPc acts as a PDT precursor that produces singlet oxygen. AuNR is a PTT agent with LSPR, which means that hyperthermia caused by AuNRs can increase oxygenation within the tumor and blood flow, hence increasing PDT. In Tham's work, NIR light stimulated both ZnPc and AuNR, causing hyperthermia and ROS near the tumor location, resulting in synergistic PTT and PDT Fig. 28.320
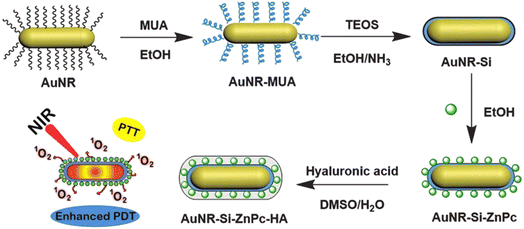 |
| Fig. 28 Schematic representation of AuNR-Si-ZnPc-HA (Tham et al., 2016).320 | |
Kalluru et al. created a GO-PEG-folate nanocomposite with PTT and PDT therapeutic effects on tumors. The nanomaterial's core, GO, has inherent photothermal characteristics and can produce a singlet oxygen for PDT. In vitro experiments showed that PDT and PTT both caused cellular death, and in vivo experiments supported that the combinatorial therapy was more efficient and powerful than PDT or PTT alone.321 Wang et al. created a multifunctional nanoplatform by covalently introduced upconversion nanoparticles (UCNPs) using nanographene oxide (NGO) and loading ZnPc on its surface. In UCNPs-NGO/ZnPc, ZnPc were employed as a PS for PDT, while UCNPs served as PTT agents for PTT. NGO then served as a carrier of ZnPc and UCNPs owing to its strong NIR absorption and huge surface area. Based on the results of this research, the combination treatment performed much better in cancer therapy.322 A combinatorial therapy by synergistic work of PTT along with PDT will be advantageous for killing cancer cell with minimal side effects to healthy cells and tissues.
8. Barriers and challenges in clinical translation of nanomaterials for PDT and PTT
Current investigations on nanotechnology-driven photodynamic therapy (PDT) and photothermal therapy (PTT) for cancer treatment have significant limitations that prevent its clinical use. One significant constraint is that most research is limited to preclinical phases, with just a few nanomaterials progressing to human clinical trials. This disparity is mostly owing to obstacles such as possible toxicity, uncertain immunological responses, and rigorous regulatory procedures. Furthermore, the variability of tumor microenvironments is a difficulty, since these medicines frequently show uneven effectiveness across cancer kinds and stages. Another major concern is the lack of real-time monitoring tools that allow for exact management of therapeutic effects while minimizing injury to adjacent healthy tissues. Furthermore, scalability and reproducibility in nanomaterial fabrication remain a challenge, resulting in heterogeneity in performance.323,324
To address these concerns, additional extensive in vivo studies on long-term safety, pharmacokinetics, and biodistribution are required. Standardizing techniques for nanomaterial fabrication and functionalization would increase repeatability and ease regulatory approval. Integrating real-time imaging tools might improve therapeutic precision, but creating patient-specific approaches could assist overcome tumor heterogeneity. Furthermore, combining PDT and PTT with other treatment methods, including as immunotherapy and chemotherapy, has the potential to improve therapeutic results and widen these techniques' clinical application.
Despite substantial advances in nanotechnology-driven photodynamic treatment (PDT) and photothermal therapy (PTT), only a few nanomaterials, including liposomal photosensitizers and gold-based nanoparticles, have made it to clinical trials. However, most nanomaterials are still in the preclinical stage due to a variety of obstacles. One major concern is their possible toxicity and long-term biocompatibility, since many nanomaterials can persist in healthy tissues, causing undesirable side effects or immunological reactions. Regulatory barriers also play an important role, with high safety and effectiveness standards that are difficult to achieve due to unpredictability in nanomaterial manufacturing and performance. Furthermore, the variability of tumor microenvironments hinders their efficacy, because different malignancies respond differently to various medicines. Another impediment is the absence of standardized testing methodologies, which makes it difficult to compare preclinical data and construct a strong case for clinical trials. Finally, exorbitant development costs and limited financing keep many promising nanomaterials from moving forward. To address these problems, more predictive preclinical models, standardized evaluation methodologies, better nanomaterial designs for increased safety, and greater coordination among researchers, industry, and regulatory authorities will be required to shorten the approval process.324,325
9. Conclusion
To summarize, the use of nanomaterials into photodynamic therapy (PDT) and photothermal therapy (PTT) is a potential area in cancer treatment. Nanomaterials such as gold, silver, silica, quantum dots, carbon-based nanomaterials, and manganese dioxide nanosheets have demonstrated remarkable therapeutic efficacy in PDT and PTT by improving photosensitizer and photothermal agent targeting, stability, and bioavailability, respectively. These advancements make cancer therapy more accurate and effective, with fewer side effects.
However, there are still obstacles in improving the design of nanomaterial-based delivery vehicles for cancer treatment. One major concern is the possible toxicity of several nanoparticles, especially at high concentrations or after extended exposure, which may restrict their therapeutic utility. Furthermore, the complicated biological milieu of tumors, including hypoxia and diverse vascularization, frequently impairs the efficiency of these treatments. Furthermore, the possibility of nanomaterial degradation and the immune system's reaction to these materials are major challenges that must be addressed for their effective clinical translation.
Future methods should concentrate on creating more biocompatible and biodegradable nanomaterials, increasing the specificity and selectivity of nanoparticle distribution to tumor locations, and improving the controlled release of therapeutic chemicals. Combining PDT and PTT with other modalities, such as chemotherapy, radiation, and immunotherapy, has considerable potential for synergistic benefits, allowing for more complete cancer treatment. Furthermore, advances in nanomaterial functionalization, real-time imaging, and personalised treatment regimens will be critical to improve the overall clinical results of nanomaterial-based cancer treatments. Finally, more study into molecular interactions, nanoparticle formulation optimization, and integration with multi-modal therapy will pave the way for more effective and safer cancer treatments.
Data availability
No new data sets were generated during the study.
Conflicts of interest
The authors declare no conflict of interests regarding the publication of this paper.
Acknowledgements
The authors are thankful to the management of Saveetha school of engineering, SIMATS, Chennai, Tamil Nadu, India.
References
- D. W. Felsher and J. M. Bishop, Reversible tumorigenesis by MYC in hematopoietic lineages, Mol. Cell, 1999, 4(2), 199–207 CrossRef CAS PubMed.
- P. Agostinis, K. Berg, K. A. Cengel, T. H. Foster, A. W. Girotti, S. O. Gollnick, S. M. Hahn, M. R. Hamblin, A. Juzeniene, D. Kessel and M. Korbelik, Photodynamic therapy of cancer: an update, Ca-Cancer J. Clin., 2011, 61(4), 250–281 CrossRef PubMed.
- R. Bhole, C. Bonde, P. Kadam and R. Wavwale, A comprehensive review on photodynamic therapy (PDT) and photothermal therapy (PTT) for cancer treatment, Turk. J. Oncol., 2021, 36(1), 125 Search PubMed.
- V. I. Group1A, Verteporfin therapy of subfoveal choroidal neovascularization in age-related macular degeneration: two-year results of a randomized clinical trial including lesions with occult with no classic choroidal neovascularization—verteporfin in photodynamic therapy report 2, Am. J. Ophthalmol., 2001, 131(5), 541–560 CrossRef.
- C. J. Gomer and N. J. Razum, Acute skin response in albino mice following porphyrin photosensitization under oxic and anoxic conditions, Photochem. Photobiol., 1984, 40(4), 435–439 CrossRef CAS.
- W. R. Chen, R. L. Adams, S. Heaton, D. T. Dickey, K. E. Bartels and R. E. Nordquist, Chromophore-enhanced laser-tumor tissue photothermal interaction using an 808-nm diode laser, Cancer Lett., 1995, 88(1), 15–19 CrossRef CAS PubMed.
- L. Zou, H. Wang, B. He, L. Zeng, T. Tan, H. Cao, X. He, Z. Zhang, S. Guo and Y. Li, Current approaches of photothermal therapy in treating cancer metastasis with nanotherapeutics, Theranostics, 2016, 6(6), 762 CrossRef CAS PubMed.
- U. Anand, A. Dey, A. K. Chandel, R. Sanyal, A. Mishra, D. K. Pandey, V. De Falco, A. Upadhyay, R. Kandimalla and A. Chaudhary, JK Dhanjal, Cancer chemotherapy and beyond: Current status, drug candidates, associated risks and progress in targeted therapeutics, Genes Dis., 2023, 10(4), 1367–1401 CrossRef CAS PubMed.
- G. Mohan, A. H. TP, J. AJ, S. D. KM, A. Narayanasamy and B. Vellingiri, Recent advances in radiotherapy and its associated side effects in cancer—a review, J. Basic Appl. Zool., 2019, 80(1), 1 CrossRef.
- S. Tan, D. Li and X. Zhu, Cancer immunotherapy: Pros, cons and beyond, Biomed. Pharmacother., 2020, 124, 109821 CrossRef PubMed.
- B. Nasseri, E. Alizadeh, F. Bani, S. Davaran, A. Akbarzadeh, N. Rabiee, A. Bahadori, M. Ziaei, M. Bagherzadeh, M. R. Saeb and M. Mozafari, Nanomaterials for photothermal and photodynamic cancer therapy, Appl. Phys. Rev., 2022, 9(1), 011317 CAS.
- S. L. Li, P. Jiang, F. L. Jiang and Y. Liu, Recent advances in nanomaterial-based nanoplatforms for chemodynamic cancer therapy, Adv. Funct. Mater., 2021, 31(22), 2100243 CrossRef CAS.
- S. Liu, X. Pan and H. Liu, Two-dimensional nanomaterials for photothermal therapy, Angew. Chem., 2020, 132(15), 5943–5953 CrossRef.
- R. A. Petros and J. M. DeSimone, Strategies in the design of nanoparticles for therapeutic applications, Nat. Rev. Drug Discovery, 2010, 9(8), 615–627 CrossRef CAS PubMed.
- W. K. Shin, J. Cho, A. G. Kannan, Y. S. Lee and D. W. Kim, Cross-linked composite gel polymer electrolyte using mesoporous methacrylate-functionalized SiO2 nanoparticles for lithium-ion polymer batteries, Sci. Rep., 2016, 6(1), 26332 CrossRef CAS.
- A. Prokop and J. M. Davidson, Nanovehicular intracellular delivery systems, J. Pharm. Sci., 2008, 97(9), 3518–3590 CrossRef CAS.
- J. K. Patel and A. P. Patel, Passive targeting of nanoparticles to cancer, Surface Modification of Nanoparticles for Targeted Drug Delivery, 2019, pp. 125–143 Search PubMed.
- Z. Chen, R. K. Kankala, L. Long, S. Xie, A. Chen and L. Zou, Current understanding of passive and active targeting nanomedicines to enhance tumor accumulation, Coord. Chem. Rev., 2023, 481, 215051 CrossRef CAS.
- R. P. Das, V. V. Gandhi, B. G. Singh and A. Kunwar, Passive and active drug targeting: role of nanocarriers in rational design of anticancer formulations, Curr. Pharm. Des., 2019, 25(28), 3034–3056 CrossRef CAS PubMed.
- B. Dutta, K. C. Barick and P. A. Hassan, Recent advances in active targeting of nanomaterials for anticancer drug delivery, Adv. Colloid Interface Sci., 2021, 296, 102509 CrossRef CAS PubMed.
- M. H. Akhter, M. Rizwanullah, J. Ahmad, M. J. Ahsan, M. A. Mujtaba and S. Amin, Nanocarriers in advanced drug targeting: setting novel paradigm in cancer therapeutics, Artif. Cells, Nanomed., Biotechnol., 2018, 46(5), 873–884 CrossRef CAS PubMed.
- M. Alavi and M. Hamidi, Passive and active targeting in cancer therapy by liposomes and lipid nanoparticles, Drug Metab. Pers. Ther., 2019, 34(1), 20180032 Search PubMed.
- S. M. Narum, T. Le, D. P. Le, J. C. Lee, N. D. Donahue and W. Yang, S. Wilhelm, Passive targeting in nanomedicine: fundamental concepts, body interactions, and clinical potential. In Nanoparticles for Biomedical Applications, Elsevier, 2020, pp. 37–53 Search PubMed.
- F. S. Anarjan, Active targeting drug delivery nanocarriers: Ligands, Nano-Struct. Nano-Objects, 2019, 19, 100370 CrossRef.
- A. K. Pearce and R. K. O'Reilly, Insights into active targeting of nanoparticles in drug delivery: advances in clinical studies and design considerations for cancer nanomedicine, Bioconjugate Chem., 2019, 30(9), 2300–2311 CrossRef CAS PubMed.
- T. D. Clemons, R. Singh, A. Sorolla, N. Chaudhari, A. Hubbard and K. S. Iyer, Distinction between active and passive targeting of nanoparticles dictate their overall therapeutic efficacy, Langmuir, 2018, 34(50), 15343–15349 CrossRef CAS.
- M. F. Attia, N. Anton, J. Wallyn, Z. Omran and T. F. Vandamme, An overview of active and passive targeting strategies to improve the nanocarriers efficiency to tumour sites, J. Pharm. Pharmacol., 2019, 71(8), 1185–1198 CrossRef CAS.
- Y. Yao, Y. Zhou, L. Liu, Y. Xu, Q. Chen, Y. Wang, S. Wu, Y. Deng, J. Zhang and A. Shao, Nanoparticle-based drug delivery in cancer therapy and its role in overcoming drug resistance, Front. Mol. Biosci., 2020, 7, 193 CrossRef CAS.
- Y. Dang and J. Guan, Nanoparticle-based drug delivery systems for cancer therapy, Smart Mater. Med., 2020, 1, 10–19 CrossRef.
- D. Mundekkad and W. C. Cho, Nanoparticles in clinical translation for cancer therapy, Int. J. Mol. Sci., 2022, 23(3), 1685 CrossRef CAS PubMed.
- Y. Gao, K. Wang, J. Zhang, X. Duan, Q. Sun and K. Men, Multifunctional nanoparticle for cancer therapy, MedComm, 2023, 4(1), e187 CrossRef CAS.
- D. Peer, J. M. Karp, S. Hong, O. C. Farokhzad, R. Margalit and R. Langer, Nanocarriers as an emerging platform for cancer therapy, Nano-Enabled Med. Appl., 2020, 23, 61–91 Search PubMed.
- J. Chen, T. Fan, Z. Xie, Q. Zeng, P. Xue, T. Zheng, Y. Chen, X. Luo and H. Zhang, Advances in nanomaterials for photodynamic therapy applications: Status and challenges, Biomaterials, 2020, 237, 119827 CrossRef CAS.
- Z. Yang, Z. Sun, Y. Ren, X. Chen, W. Zhang, X. Zhu, Z. Mao, J. Shen and S. Nie, Advances in nanomaterials for use in photothermal and photodynamic therapeutics, Mol. Med. Rep., 2019, 20(1), 5–15 CAS.
- Z. Cheng, M. Li, R. Dey and Y. Chen, Nanomaterials for cancer therapy: current progress and perspectives, J. Hematol. Oncol., 2021, 14, 1–27 CrossRef.
- J. Wang, X. Wu, P. Shen, J. Wang, Y. Shen, Y. Shen, T. J. Webster and J. Deng, Applications of inorganic nanomaterials in photothermal therapy based on combinational cancer treatment, Int. J. Nanomed., 2020, 19, 1903–1914 CrossRef.
- G. Chen, Y. Qian, H. Zhang, A. Ullah, X. He, Z. Zhou, H. Fenniri and J. Shen, Advances in cancer theranostics using organic-inorganic hybrid nanotechnology, Appl. Mater. Today, 2021, 23, 101003 CrossRef.
- A. A. Ali, W. H. Abuwatfa, M. H. Al-Sayah and G. A. Husseini, Gold-nanoparticle hybrid nanostructures for multimodal cancer therapy, Nanomaterials, 2022, 12(20), 3706 CrossRef CAS PubMed.
- P. G. Calavia, G. Bruce, L. Pérez-García and D. A. Russell, Photosensitiser-gold nanoparticle conjugates for photodynamic therapy of cancer, Photochem. Photobiol. Sci., 2018, 17(11), 1534–1552 CrossRef.
- Y. Zheng, Z. Li, H. Chen and Y. Gao, Nanoparticle-based drug delivery systems for controllable photodynamic cancer therapy, Eur. J. Pharm. Sci., 2020, 144, 105213 CrossRef.
- J. Li, J. Li, Y. Pu, S. Li, W. Gao and B. He, PDT-enhanced ferroptosis by a polymer nanoparticle with pH-activated singlet oxygen generation and superb biocompatibility for cancer therapy, Biomacromolecules, 2021, 22(3), 1167–1176 CrossRef CAS.
- G. M. Calixto, J. Bernegossi, L. M. De Freitas, C. R. Fontana and M. Chorilli, Nanotechnology-based drug delivery systems for photodynamic therapy of cancer: a review, Molecules, 2016, 21(3), 342 CrossRef.
- M. S. Baptista, J. Cadet, P. Di Mascio, A. A. Ghogare, A. Greer, M. R. Hamblin, C. Lorente, S. C. Nunez, M. S. Ribeiro, A. H. Thomas and M. Vignoni, Type I and type II photosensitized oxidation reactions: guidelines and mechanistic pathways, Photochem. Photobiol., 2017, 93(4), 912–919 CrossRef CAS PubMed.
- U. Chitgupi, Y. Qin and J. F. Lovell, Targeted nanomaterials for phototherapy, Nanotheranostics, 2017, 1(1), 38 CrossRef.
- H. Barr, C. J. Tralau, P. B. Boulos, A. J. MacRobert, R. Tilly and S. G. Bown, The contrasting mechanisms of colonic collagen damage between photodynamic therapy and thermal injury, Photochem. Photobiol., 1987, 46(5), 795–800 CrossRef CAS.
- F. J. Civantos, B. Karakullukcu, M. Biel, C. E. Silver, A. Rinaldo, N. F. Saba, R. P. Takes, V. Vander Poorten and A. Ferlito, A review of photodynamic therapy for neoplasms of the head and neck, Adv. Ther., 2018, 35, 324–340 CrossRef CAS PubMed.
- V. P. Pattani, J. Shah, A. Atalis, A. Sharma and J. W. Tunnell, Role of apoptosis and necrosis in cell death induced by nanoparticle-mediated photothermal therapy, J. Nanopart. Res., 2015, 17, 1 CrossRef CAS.
- S. Stapleton, M. Dunne, M. Milosevic, C. W. Tran, M. J. Gold, A. Vedadi, T. D. Mckee, P. S. Ohashi, C. Allen and D. A. Jaffray, Radiation and heat improve the delivery and efficacy of nanotherapeutics by modulating intratumoral fluid dynamics, ACS Nano, 2018, 12(8), 7583–7600 CrossRef CAS.
- D. Kessel, Y. Luo, Y. Deng and C. K. Chang, The role of subcellular localization in initiation of apoptosis by photodynamic therapy, Photochem. Photobiol., 1997, 65(3), 422 CrossRef CAS.
- I. Rizvi, G. Obaid, S. Bano, T. Hasan and D. Kessel, Photodynamic therapy: Promoting in vitro efficacy of photodynamic therapy by liposomal formulations of a photosensitizing agent, Lasers Surg. Med., 2018, 50(5), 499–505 Search PubMed.
- H. R. Choi Kim, Y. Luo, G. Li and D. Kessel, Enhanced apoptotic response to photodynamic therapy after bcl-2 transfection, Cancer Res., 1999, 59(14), 3429–3432 Search PubMed.
- S. Nath, G. Obaid and T. Hasan, The course of immune stimulation by photodynamic therapy: bridging fundamentals of photochemically induced immunogenic cell death to the enrichment of T-cell repertoire, Photochem. Photobiol., 2019, 95(6), 1288–1305 CrossRef CAS PubMed.
- Y. Xiong, Y. Rao, J. Hu, Z. Luo and C. Chen, Nanoparticle-Based Photothermal Therapy for Breast Cancer Noninvasive Treatment, Adv. Mater., 2023, 10, 2305140 CrossRef PubMed.
- B. Chen, Y. Xu, P. Agostinis and P. A. De Witte, Synergistic effect of photodynamic therapy with hypericin in combination with hyperthermia on loss of clonogenicity of RIF-1 cells, Int. J. Oncol., 2001, 18(6), 1279–1285 CAS.
- L. O. Svaasand, D. R. Doiron and T. J. Dougherty, Temperature rise during photoradiation therapy of malignant tumors, Med. Phys., 1983, 10(1), 10–17 CrossRef CAS.
- Y. Zhang, X. Zhan, J. Xiong, S. Peng, W. Huang, R. Joshi, Y. Cai, Y. Liu, R. Li, K. Yuan and N. Zhou, Temperature-dependent cell death patterns induced by functionalized gold nanoparticle photothermal therapy in melanoma cells, Sci. Rep., 2018, 8(1), 8720 CrossRef.
- R. Geoghegan, G. Ter Haar, K. Nightingale, L. Marks and S. Natarajan, Methods of monitoring thermal ablation of soft tissue tumors–A comprehensive review, Med. Phys., 2022, 49(2), 769–791 CrossRef.
- G. Gunaydin, M. E. Gedik and S. Ayan, Photodynamic therapy—current limitations and novel approaches, Front. Chem., 2021, 9, 691697 CrossRef CAS PubMed.
- C. M. Moore, D. Pendse and M. Emberton, Photodynamic therapy for prostate cancer—a review of current status and future promise, Nat. Clin. Pract. Urol., 2009, 6(1), 18–30 CrossRef CAS PubMed.
- A. P. Castano, P. Mroz and M. R. Hamblin, Photodynamic therapy and anti-tumour immunity, Nat. Rev. Cancer, 2006, 6(7), 535–545 CrossRef CAS.
- N. L. Oleinick, R. L. Morris and I. Belichenko, The role of apoptosis in response to photodynamic therapy: what, where, why, and how, Photochem. Photobiol. Sci., 2002, 1(1), 1–21 CrossRef CAS PubMed.
- B. Krammer, Vascular effects of photodynamic therapy, Anticancer Res., 2001, 21(6B), 4271–4277 CAS.
- D. E. Dolmans, A. Kadambi, J. S. Hill, C. A. Waters, B. C. Robinson, J. P. Walker, D. Fukumura and R. K. Jain, Vascular accumulation of a novel photosensitizer, MV6401, causes selective thrombosis in tumor vessels after photodynamic therapy, Cancer Res., 2002, 62(7), 2151–2156 CAS.
- G. Canti, A. De Simone and M. Korbelik, Photodynamic therapy and the immune system in experimental oncology, Photochem. Photobiol. Sci., 2002, 1(1), 79–80 CrossRef CAS.
- S. G. Bown, A. Z. Rogowska, D. E. Whitelaw, W. R. Lees, L. B. Lovat, P. Ripley, L. Jones, P. Wyld, A. Gillams and A. W. Hatfield, Photodynamic therapy for cancer of the pancreas, Gut, 2002, 50(4), 549–557 CrossRef CAS PubMed.
- T. J. Dougherty, C. J. Gomer, B. W. Henderson, G. Jori, D. Kessel, M. Korbelik, J. Moan and Q. Peng, Photodynamic therapy, J. Natl. Cancer Inst., 1998, 90(12), 889–905 CrossRef CAS.
- T. M. Busch, E. P. Wileyto, M. J. Emanuele, F. Del Piero, L. Marconato, E. Glatstein and C. J. Koch, Photodynamic therapy creates fluence rate-dependent gradients in the intratumoral spatial distribution of oxygen, Cancer Res., 2002, 62(24), 7273–7279 CAS.
- Q. Peng and J. M. Nesland, Effects of photodynamic therapy on tumor stroma, Ultrastruct. Pathol., 2004, 28(5–6), 333–340 CrossRef.
- B. W. Henderson, S. M. Waldow, T. S. Mang, W. R. Potter, P. B. Malone and T. J. Dougherty, Tumor destruction and kinetics of tumor cell death in two experimental mouse tumors following photodynamic therapy, Cancer Res., 1985, 45(2), 572–576 CAS.
- D. Kessel, M. G. Vicente and J. J. Reiners Jr, Initiation of apoptosis and autophagy by photodynamic therapy, Lasers Surg. Med., 2006, 38(5), 482–488 CrossRef PubMed.
- J. O. Yoo and K. S. Ha, New insights into the mechanisms for photodynamic therapy-induced cancer cell death, Int. Rev. Cell Mol. Biol., 2012, 295, 139–174 CAS.
- M. L. Agarwal, M. E. Clay, E. J. Harvey, H. H. Evans, A. R. Antunez and N. L. Oleinick, Photodynamic therapy induces rapid cell death by apoptosis in L5178Y mouse lymphoma cells, Cancer Res., 1991, 51(21), 5993–5996 CAS.
- D. R. Mokoena, B. P. George and H. Abrahamse, Photodynamic therapy induced cell death mechanisms in breast cancer, Int. J. Mol. Sci., 2021, 22(19), 10506 CrossRef CAS PubMed.
- H. E. Yoon, M. Y. Ahn, Y. C. Kim and J. H. Yoon, Involvement of endoplasmic reticulum stress and cell death by synthesized Pa-PDT in oral squamous cell carcinoma cells, J. Dent. Sci., 2022, 17(4), 1722–1730 CrossRef PubMed.
- A. F. Dos Santos, A. Inague, G. S. Arini, L. F. Terra, R. A. Wailemann, A. C. Pimentel, M. Y. Yoshinaga, R. R. Silva, D. Severino, D. R. de Almeida and V. M. Gomes, Distinct photo-oxidation-induced cell death pathways lead to selective killing of human breast cancer cells, Cell Death Dis., 2020, 11(12), 1070 CrossRef CAS.
- Y. Y. Wang, Y. K. Chen, C. S. Hu, L. Y. Xiao, W. L. Huang, T. C. Chi, K. H. Cheng, Y. M. Wang and S. S. Yuan, MAL-PDT inhibits oral precancerous cells and lesions via autophagic cell death, Oral Dis., 2019, 25(3), 758–771 CrossRef PubMed.
- Z. Sun, M. Zhao, W. Wang, L. Hong, Z. Wu, G. Luo, S. Lu, Y. Tang, J. Li, J. Wang and Y. Zhang, 5-ALA mediated photodynamic therapy with combined treatment improves anti-tumor efficacy of immunotherapy through boosting immunogenic cell death, Cancer Lett., 2023, 554, 216032 CrossRef CAS.
- J. Zhang, L. Liu, X. Li, X. Shen, G. Yang, Y. Deng, Z. Hu, J. Zhang and Y. Lu, 5-ALA-PDT induced ferroptosis in keloid fibroblasts via ROS, accompanied by downregulation of xCT, GPX4, Photodiagn. Photodyn. Ther., 2023, 42, 103612 CrossRef CAS.
- A. A. Abdelrahim, S. Hong and J. M. Song, Integrative in situ photodynamic therapy-induced cell death measurement of 3D-bioprinted MCF-7 tumor spheroids, Anal. Chem., 2022, 94(40), 13936–13943 CrossRef CAS PubMed.
- Y. Huang, Z. Xiao, Z. Guan, Y. Shen, Y. Jiang, X. Xu, Z. Huang and C. Zhao, A light-triggered self-reinforced nanoagent for targeted
chemo-photodynamic therapy of breast cancer bone metastases via ER stress and mitochondria mediated apoptotic pathways, J. Controlled Release, 2020, 319, 119–134 CrossRef CAS PubMed.
- D. Kessel, Photodynamic therapy: apoptosis, paraptosis and beyond, Apoptosis, 2020, 25(9), 611–615 CrossRef PubMed.
- G. C. Lamarque, D. A. Méndez, A. A. Matos, T. J. Dionísio, M. A. Machado, A. C. Magalhães, R. C. Oliveira and T. Cruvinel, Cytotoxic effect and apoptosis pathways activated by methylene blue-mediated photodynamic therapy in fibroblasts, Photodiagn. Photodyn. Ther., 2020, 29, 101654 CrossRef.
- C. Song, W. Xu, H. Wu, X. Wang, Q. Gong, C. Liu, J. Liu and L. Zhou, Photodynamic therapy induces autophagy-mediated cell death in human colorectal cancer cells via activation of the ROS/JNK signaling pathway, Cell Death Dis., 2020, 11(10), 938 CrossRef CAS PubMed.
- F. Valli, M. C. Vior, L. P. Roguin and J. Marino, Crosstalk between oxidative stress-induced apoptotic and autophagic signaling pathways in Zn (II) phthalocyanine photodynamic therapy of melanoma, Free Radicals Biol. Med., 2020, 152, 743–754 CrossRef CAS.
- L. Ke, F. Wei, L. Xie, J. Karges, Y. Chen, L. Ji and H. Chao, A Biodegradable Iridium (III) Coordination Polymer for Enhanced Two-Photon Photodynamic Therapy Using an Apoptosis–Ferroptosis Hybrid Pathway, Angew. Chem., Int. Ed., 2022, 61(28), e202205429 CrossRef CAS PubMed.
- X. Wang, Q. Gong, C. Song, J. Fang, Y. Yang, X. Liang, X. Huang and J. Liu, Berberine-photodynamic therapy sensitizes melanoma cells to cisplatin-induced apoptosis through ROS-mediated P38 MAPK pathways, Toxicol. Appl. Pharmacol., 2021, 418, 115484 CrossRef CAS.
- B. Ortel, C. R. Shea and P. Calzavara-Pinton, Molecular mechanisms of photodynamic therapy, Front. Biosci., 2009, 14(14), 4157–4172 CrossRef CAS PubMed.
- K. Plaetzer, T. Kiesslich, C. B. Oberdanner and B. Krammer, Apoptosis following photodynamic tumor therapy: induction, mechanisms and detection, Curr. Pharm. Des., 2005, 11(9), 1151–1165 CrossRef CAS PubMed.
- T. Kaneko, H. Chiba, T. Yasuda and K. Kusama, Detection of photodynamic therapy-induced early apoptosis in human salivary gland tumor cells in vitro and in a mouse tumor model, Oral Oncol., 2004, 40(8), 787–792 CrossRef CAS PubMed.
- R. Chaloupka, T. Obšil, J. Plášek and F. Sureau, The effect of hypericin and hypocrellin-A on lipid membranes and membrane potential of 3T3 fibroblasts, Biochim. Biophys. Acta, Biomembr., 1999, 1418(1), 39–47 CrossRef CAS PubMed.
- D. Kessel and Y. Luo, Photodynamic therapy: a mitochondrial inducer of apoptosis, Cell Death Differ., 1999, 6(1), 28–35 CrossRef CAS PubMed.
- A. J. Kowaltowski, J. Turin, G. L. Indig and A. E. Vercesi, Mitochondrial effects of triarylmethane dyes, J. Bioenerg. Biomembr., 1999, 31, 581–590 CrossRef CAS.
- E. Ucar, O. Seven, D. Lee, G. Kim, J. Yoon and E. U. Akkaya, Selectivity in photodynamic action: higher activity of mitochondria targeting photosensitizers in cancer cells, ChemPhotoChem, 2019, 3(3), 129–132 CrossRef CAS.
- R. Wang, X. Li and J. Yoon, Organelle-targeted photosensitizers for precision photodynamic therapy, ACS Appl. Mater. Interfaces, 2021, 13(17), 19543–19571 CrossRef CAS PubMed.
- I. F. Mariz, S. N. Pinto, A. M. Santiago, J. M. Martinho, J. Recio, J. J. Vaquero, A. M. Cuadro and E. Maçôas, Two-photon activated precision molecular photosensitizer targeting mitochondria, Commun. Chem., 2021, 4(1), 142 CrossRef CAS PubMed.
- M. Yang, J. Deng, D. Guo, Q. Sun, Z. Wang, K. Wang and F. Wu, Mitochondria-targeting Pt/Mn porphyrins as
efficient photosensitizers for magnetic resonance imaging and photodynamic therapy, Dyes Pigm., 2019, 166, 189–195 CrossRef CAS.
- Y. Ni, H. Zhang, C. Chai, B. Peng, A. Zhao, J. Zhang, L. Li, C. Zhang, B. Ma, H. Bai and K. L. Lim, Mitochondria-Targeted Two-Photon Fluorescent Photosensitizers for Cancer Cell Apoptosis via Spatial Selectability, Adv. Healthcare Mater., 2019, 8(14), 1900212 CrossRef.
- B. Wang, H. Zhou, L. Chen, Y. Ding, X. Zhang, H. Chen, H. Liu, P. Li, Y. Chen, C. Yin and Q. Fan, A Mitochondria-Targeted Photosensitizer for Combined Pyroptosis and Apoptosis with NIR-II Imaging/Photoacoustic Imaging-Guided Phototherapy, Angew. Chem., Int. Ed., 2024, 23, e202408874 Search PubMed.
- X. Zhao, Y. Huang, G. Yuan, K. Zuo, Y. Huang, J. Chen, J. Li and J. Xue, A novel tumor and mitochondria dual-targeted photosensitizer showing ultra-efficient photodynamic anticancer activities, Chem. Commun., 2019, 55(6), 866–869 RSC.
- D. J. Granville, C. M. Carthy, H. Jiang, J. G. Levy, B. M. McManus, J. Y. Matroule, J. Piette and D. W. Hunt, Nuclear factor-κB activation by the photochemotherapeutic agent verteporfin, Blood, 2000, 95(1), 256–262 CrossRef CAS.
- L. Y. Xue, S. M. Chiu and N. L. Oleinick, Photodynamic therapy-induced death of MCF-7 human breast cancer cells: a role for caspase-3 in the late steps of apoptosis but not for the critical lethal event, Exp. Cell Res., 2001, 263(1), 145–155 CrossRef CAS PubMed.
- D. J. Granville, J. G. Levy and D. W. Hunt, Photodynamic therapy induces caspase-3 activation in HL-60 cells, Cell Death Differ., 1997, 4(7), 623–628 CrossRef CAS.
- A. C. Moor, Signaling pathways in cell death and survival after photodynamic therapy, J. Photochem. Photobiol., B, 2000, 57(1), 1–3 CrossRef CAS PubMed.
- M. Korbelik and G. Krosl, Cellular levels of photosensitisers in tumours: the role of proximity to the blood supply, Br. J. Cancer, 1994, 70(4), 604–610 CrossRef CAS.
- B. J. Tromberg, A. Orenstein, S. Kimel, S. J. Barker, J. Hyatt, J. S. Nelson and M. W. Berns, In vivo tumor oxygen tension measurements for the evaluation of the efficiency of photodynamic therapy, Photochem. Photobiol., 1990, 52(2), 375–385 CrossRef CAS PubMed.
- B. W. Pogue, R. D. Braun, J. L. Lanzen, C. Erickson and M. W. Dewhirst, Analysis of the Heterogeneity of pO2 Dynamics During Photodynamic Therapy with Verteporfin, Photochem. Photobiol., 2001, 74(5), 700–706 CrossRef CAS PubMed.
- I. S. Turan, D. Yildiz, A. Turksoy, G. Gunaydin and E. U. Akkaya, A bifunctional photosensitizer for enhanced fractional photodynamic therapy: singlet oxygen generation in the presence and absence of light, Angew. Chem., Int. Ed., 2016, 55(8), 2875–2878 CrossRef CAS.
- R. K. Jain and T. Stylianopoulos, Delivering nanomedicine to solid tumors, Nat. Rev. Clin. Oncol., 2010, 7(11), 653–664 CrossRef CAS.
- S. J. Martin, C. M. Henry and S. P. Cullen, A perspective on mammalian caspases as positive and negative regulators of inflammation, Mol. Cell, 2012, 46(4), 387–397 CrossRef CAS.
- J. L. Li and M. Gu, Surface plasmonic gold nanorods for enhanced two-photon microscopic imaging and apoptosis induction of cancer cells, Biomaterials, 2010, 31(36), 9492–9498 CrossRef CAS.
- M. Pérez-Hernández, P. Del Pino, S. G. Mitchell, M. Moros, G. Stepien, B. Pelaz, W. J. Parak, E. M. Galvez, J. Pardo and J. M. de la Fuente, Dissecting the molecular mechanism of apoptosis during photothermal therapy using gold nanoprisms, ACS Nano, 2015, 9(1), 52–61 CrossRef.
- T. Cirman, K. Oresić, G. D. Mazovec, V. Turk, J. C. Reed, R. M. Myers, G. S. Salvesen and B. Turk, Selective disruption of lysosomes in HeLa cells triggers apoptosis mediated by cleavage of Bid by multiple papain-like lysosomal cathepsins, J. Biol. Chem., 2004, 279(5), 3578–3587 CrossRef CAS PubMed.
- J. M. Stern, V. V. Kibanov Solomonov, E. Sazykina, J. A. Schwartz, S. C. Gad and G. P. Goodrich, Initial evaluation of the safety of nanoshell-directed photothermal therapy in the treatment of prostate disease, Int. J. Toxicol., 2016, 35(1), 38–46 CrossRef CAS PubMed.
- G. Alfranca and D. Cui, The true complexity of photothermal therapy: A brief perspective, Nano Biomed. Eng., 2017, 9(2), 129–134 CAS.
- C. Ayala-Orozco, C. Urban, M. W. Knight, A. S. Urban, O. Neumann, S. W. Bishnoi, S. Mukherjee, A. M. Goodman, H. Charron, T. Mitchell and M. Shea, Au nanomatryoshkas as efficient near-infrared photothermal transducers for cancer treatment: benchmarking against nanoshells, ACS Nano, 2014, 8(6), 6372–6381 CrossRef CAS PubMed.
- L. O. Svaasand, C. J. Gomer and E. Morinelli, On the physical rationale of laser induced hyperthermia, Lasers Med. Sci., 1990, 5, 121–128 CrossRef.
- V. P. Nguyen, S. W. Kim, H. Kim, H. Kim, K. H. Seok, M. J. Jung, Y. C. Ahn and H. W. Kang, Biocompatible astaxanthin as a novel marine-oriented agent for dual chemo-photothermal therapy, PLoS one, 2017, 12(4), e0174687 CrossRef.
- Z. Qin and J. C. Bischof, Thermophysical and biological responses of gold nanoparticle laser heating, Chem. Soc. Rev., 2012, 41(3), 1191–1217 RSC.
- B. E. Smith, P. B. Roder, X. Zhou and P. J. Pauzauskie, Nanoscale materials for hyperthermal theranostics, Nanoscale, 2015, 7(16), 7115–7126 RSC.
- M. Pérez-Hernández, Mechanisms of cell death induced by optical hyperthermia, In Nanomaterials for Magnetic and Optical Hyperthermia Applications, Elsevier, 2019, pp. 201–228 Search PubMed.
- S. Lal, S. E. Clare and N. J. Halas, Nanoshell-enabled photothermal cancer therapy: impending clinical impact, Acc. Chem. Res., 2008, 41(12), 1842–1851 CrossRef CAS.
- M. Aioub, S. R. Panikkanvalappil and M. A. El-Sayed, Platinum-coated gold nanorods: efficient reactive oxygen scavengers that prevent oxidative damage toward healthy, untreated cells during plasmonic photothermal therapy, ACS Nano, 2017, 11(1), 579–586 CrossRef CAS.
- L. Minai, D. Yeheskely-Hayon and D. Yelin, High levels of reactive oxygen species in gold nanoparticle-targeted cancer cells following femtosecond pulse irradiation, Sci. Rep., 2013, 3(1), 2146 CrossRef PubMed.
- M. R. Ali, Y. Wu, T. Han, X. Zang, H. Xiao, Y. Tang, R. Wu, F. M. Fernández and M. A. El-Sayed, Simultaneous time-dependent surface-enhanced Raman spectroscopy, metabolomics, and proteomics reveal cancer cell death mechanisms associated with gold nanorod photothermal therapy, J. Am. Chem. Soc., 2016, 138(47), 15434–15442 CrossRef CAS.
- J. J. Li, D. Hartono, C. N. Ong, B. H. Bay and L. Y. Yung, Autophagy and oxidative stress associated with gold nanoparticles, Biomaterials, 2010, 31(23), 5996–6003 CrossRef CAS.
- X. Ma, Y. Wu, S. Jin, Y. Tian, X. Zhang, Y. Zhao, L. Yu and X. J. Liang, Gold nanoparticles induce autophagosome accumulation through size-dependent nanoparticle uptake and lysosome impairment, ACS Nano, 2011, 5(11), 8629–8639 CrossRef CAS PubMed.
- Z. Zhou, Y. Yan, K. Hu, Y. Zou, Y. Li, R. Ma, Q. Zhang and Y. Cheng, Autophagy inhibition enabled efficient photothermal therapy at a mild temperature, Biomaterials, 2017, 141, 116–124 CrossRef CAS PubMed.
- V. L. Crotzer and J. S. Blum, Autophagy and adaptive immunity, Immunology, 2010, 131(1), 9–17 CrossRef CAS PubMed.
- D. M. Katschinski, On heat and cells and proteins, Physiology, 2004, 19(1), 11–15 CrossRef CAS PubMed.
- J. W. Fisher, S. Sarkar, C. F. Buchanan, C. S. Szot, J. Whitney, H. C. Hatcher, S. V. Torti, C. G. Rylander and M. N. Rylander, Photothermal response of human and murine cancer cells to multiwalled carbon nanotubes after laser irradiation, Cancer Res., 2010, 70(23), 9855–9864 CrossRef CAS PubMed.
- S. K. Calderwood, M. A. Khaleque, D. B. Sawyer and D. R. Ciocca, Heat shock proteins in cancer: chaperones of tumorigenesis, Trends Biochem. Sci., 2006, 31(3), 164–172 CrossRef CAS PubMed.
- X. F. Huang, W. Ren, L. Rollins, P. Pittman, M. Shah, L. Shen, Q. Gu, R. Strube, F. Hu, S. Y. Chen and A. broadly applicable, personalized heat shock protein-mediated oncolytic tumor vaccine, Cancer Res., 2003, 63(21), 7321–7329 CAS.
- K. F. Chu and D. E. Dupuy, Thermal ablation of tumours: biological mechanisms and advances in therapy, Nat. Rev. Cancer, 2014, 14(3), 199–208 CrossRef CAS PubMed.
- Q. Chen, L. Xu, C. Liang, C. Wang, R. Peng and Z. Liu, Photothermal therapy with immune-adjuvant nanoparticles together with checkpoint blockade for effective cancer immunotherapy, Nat. Commun., 2016, 7(1), 13193 CrossRef CAS PubMed.
- S. Basu, R. J. Binder, R. Suto, K. M. Anderson and P. K. Srivastava, Necrotic but not apoptotic cell death releases heat shock proteins, which deliver a partial maturation signal to dendritic cells and activate the NF-κB pathway, Int. Immunol., 2000, 12(11), 1539–1546 CrossRef CAS PubMed.
- M. Wei, N. Chen, J. Li, M. Yin, L. Liang, Y. He, H. Song, C. Fan and Q. Huang, Polyvalent immunostimulatory nanoagents with self-assembled CpG oligonucleotide-conjugated gold nanoparticles, Angew. Chem., 2012, 5(124), 1228–1232 CrossRef.
- L. A. Dykman and N. G. Khlebtsov, Immunological properties of gold nanoparticles, Chem. Sci., 2017, 8(3), 1719–1735 RSC.
- M. A. Curran, W. Montalvo, H. Yagita and J. P. Allison, PD-1 and CTLA-4 combination blockade expands infiltrating T cells and reduces regulatory T and myeloid cells within B16 melanoma tumors, Proc. Natl. Acad. Sci. U. S. A., 2010, 107(9), 4275–4280 CrossRef CAS.
- J. Fornalski, Photodynamic therapy mechanism of action and adhibition in dermatology, Nowa Medycyna, 2006, 71–74 Search PubMed.
- R. R. Allison and C. H. Sibata, Oncologic photodynamic therapy photosensitizers: a clinical review, Photodiagn. Photodyn. Ther., 2010, 7(2), 61–75 CrossRef CAS PubMed.
- A. F. Taub, Photodynamic therapy in dermatology: history and horizons, J. Drugs Dermatol., 2004, 3(1 Suppl), S8–S25 Search PubMed.
- M. H. Gold, History of photodynamic therapy. In Photodynamic Therapy in Dermatology, Springer New York, New York, NY, 2011, pp. 1–4 Search PubMed.
- H. Abrahamse and M. R. Hamblin, New photosensitizers for photodynamic therapy, Biochem. J., 2016, 473(4), 347–364 CrossRef CAS PubMed.
- D. K. Chatterjee, L. S. Fong and Y. Zhang, Nanoparticles in photodynamic therapy: an emerging paradigm, Adv. Drug Delivery Rev., 2008, 60(15), 1627–1637 CrossRef CAS.
- S. Kwiatkowski, B. Knap, D. Przystupski, J. Saczko, E. Kędzierska, K. Knap-Czop, J. Kotlińska, O. Michel, K. Kotowski and J. Kulbacka, Photodynamic therapy–mechanisms, photosensitizers and combinations, Biomed. Pharmacother., 2018, 106, 1098–1107 CrossRef.
- I. Yoon, J. Z. Li and Y. K. Shim, Advance in photosensitizers and light delivery for photodynamic therapy, Clin. Endosc., 2013, 46(1), 7–23 CrossRef PubMed.
- C. A. Morton, The emerging role of 5-ALA-PDT in dermatology: is PDT superior to standard treatments?, J. Dermatol. Treat., 2002, 13(sup1), s25–s29 CrossRef CAS PubMed.
- A. Nowak-Stepniowska, P. Pergoł and A. Padzik-Graczyk, Photodynamic method of cancer diagnosis and therapy-mechanisms and applications, Postepy Biochem., 2013, 59(1), 53–63 Search PubMed.
- L. B. Josefsen and R. W. Boyle, Photodynamic therapy: novel third-generation photosensitizers one step closer?, Br. J. Pharmacol., 2008, 154(1), 1–3 CrossRef CAS PubMed.
- P. P. Lee, P. C. Lo, E. Y. Chan, W. P. Fong, W. H. Ko and D. K. Ng, Synthesis and in vitro photodynamic activity of novel galactose-containing phthalocyanines, Tetrahedron Lett., 2005, 46(9), 1551–1554 CrossRef CAS.
- H. Kataoka, H. Nishie, N. Hayashi, M. Tanaka, A. Nomoto, S. Yano and T. Joh, New photodynamic therapy with next-generation photosensitizers, Ann. Transl. Med., 2017, 5(8) CAS.
- M. R. Hamblin, L. Y. Chiang, S. Lakshmanan, Y. Y. Huang, M. Garcia-Diaz, M. Karimi, A. N. de Souza Rastelli and R. Chandran, Nanotechnology for photodynamic therapy: A perspective from the laboratory of Dr Michael R. Hamblin in the Wellman Center for Photomedicine at Massachusetts General Hospital and Harvard Medical School, Nanotechnol. Rev., 2015, 4(4), 359–372 CAS.
- J. Krajczewski, K. Rucińska, H. E. Townley and A. Kudelski, Role of various nanoparticles in photodynamic therapy and detection methods of singlet oxygen, Photodiagn. Photodyn. Ther., 2019, 26, 162–178 CrossRef CAS PubMed.
- T. Kato, C. S. Jin, H. Ujiie, D. Lee, K. Fujino, H. Wada, H. P. Hu, R. A. Weersink, J. Chen, M. Kaji and K. Kaga, Nanoparticle targeted folate receptor 1-enhanced photodynamic therapy for lung cancer, Lung Cancer, 2017, 113, 59–68 CrossRef.
- W. H. Tsai, K. H. Yu, Y. C. Huang and C. I. Lee, EGFR-targeted photodynamic therapy by curcumin-encapsulated chitosan/TPP nanoparticles, Int. J. Nanomed., 2018, 9, 903–916 CrossRef.
- S. Wang, R. Gao, F. Zhou and M. Selke, Nanomaterials and singlet oxygen photosensitizers: potential applications in photodynamic therapy, J. Mater. Chem., 2004, 14(4), 487–493 RSC.
- S. S. Lucky, K. C. Soo and Y. Zhang, Nanoparticles in photodynamic therapy, Chem. Rev., 2015, 115(4), 1990–2042 CrossRef CAS PubMed.
- C. J. Ackerson, P. D. Jadzinsky and R. D. Kornberg, Thiolate ligands for synthesis of water-soluble gold clusters, J. Am. Chem. Soc., 2005, 127(18), 6550–6551 CrossRef CAS PubMed.
- P. Ghosh, G. Han, M. De, C. K. Kim and V. M. Rotello, Gold nanoparticles in delivery applications, Adv. Drug Delivery Rev., 2008, 60(11), 1307–1315 CrossRef CAS PubMed.
- R. Vankayala, Y. K. Huang, P. Kalluru, C. S. Chiang and K. C. Hwang, First demonstration of gold nanorods-mediated photodynamic therapeutic destruction of tumors via near infra-red light activation, Small, 2014, 10(8), 1612–1622 CAS.
- G. Pasparakis, Light-induced generation of singlet oxygen by naked gold nanoparticles and its implications to cancer cell phototherapy, Small, 2013, 9(24), 4130–4134 CAS.
- C. Jiang, T. Zhao, P. Yuan, N. Gao, Y. Pan, Z. Guan, N. Zhou and Q. H. Xu, Two-photon induced photoluminescence and singlet oxygen generation from aggregated gold nanoparticles, ACS Appl. Mater. Interfaces, 2013, 5(11), 4972–4977 CrossRef CAS PubMed.
- R. Vankayala, C. L. Kuo, A. Sagadevan, P. H. Chen, C. S. Chiang and K. C. Hwang, Morphology dependent photosensitization and formation of singlet oxygen (1 Δg) by gold and silver nanoparticles and its application in cancer treatment, J. Mater. Chem. B, 2013, 1(35), 4379–4387 RSC.
- A. Sharma, A. K. Goyal and G. Rath, Recent advances in metal nanoparticles in cancer therapy, J. Drug Targeting, 2018, 26(8), 617–632 CrossRef CAS PubMed.
- P. G. Mahajan, N. C. Dige, B. D. Vanjare, S. H. Eo, S. Y. Seo, S. J. Kim, S. K. Hong, C. S. Choi and K. H. Lee, A potential mediator for photodynamic therapy based on silver nanoparticles functionalized with porphyrin, J. Photochem. Photobiol., A, 2019, 377, 26–35 CrossRef CAS.
- P. Khoza, I. Ndhundhuma, A. Karsten and T. Nyokong, Photodynamic therapy activity of phthalocyanine-silver nanoparticles on melanoma cancer cells, J. Nanosci. Nanotechnol., 2020, 20(5), 3097–3104 CrossRef CAS PubMed.
- H. Montaseri, N. W. Nkune and H. Abrahamse, Active targeted photodynamic therapeutic effect of silver-based nanohybrids on melanoma cancer cells, J. Photochem. Photobiol., 2022, 11, 100136 CrossRef.
- F. Yan and R. Kopelman, The Embedding of Meta-tetra (Hydroxyphenyl)-Chlorin into Silica Nanoparticle Platforms for Photodynamic Therapy and Their Singlet Oxygen Production and pH-dependent Optical Properties, Photochem. Photobiol., 2003, 78(6), 587–591 CrossRef CAS.
- J. Lin, S. Wang, P. Huang, Z. Wang, S. Chen, G. Niu, W. Li, J. He, D. Cui, G. Lu and X. Chen, Photosensitizer-loaded gold vesicles with strong plasmonic coupling effect for imaging-guided photothermal/photodynamic therapy, ACS Nano, 2013, 7(6), 5320–5329 Search PubMed.
- T. Y. Ohulchanskyy, I. Roy, L. N. Goswami, Y. Chen, E. J. Bergey, R. K. Pandey, A. R. Oseroff and P. N. Prasad, Organically modified silica nanoparticles with covalently incorporated photosensitizer for photodynamic therapy of cancer, Nano Lett., 2007, 7(9), 2835–2842 CrossRef CAS PubMed.
- Y. Piao, A. Burns, J. Kim, U. Wiesner and T. Hyeon, Designed fabrication of silica-based nanostructured particle systems for nanomedicine applications, Adv. Funct. Mater., 2008, 18(23), 3745–3758 CrossRef CAS.
- P. Couleaud, V. Morosini, C. Frochot, S. Richeter, L. Raehm and J. O. Durand, Silica-based nanoparticles for photodynamic therapy applications, Nanoscale, 2010, 2(7), 1083–1095 Search PubMed.
- D. S. Nady, A. Hassan, M. U. Amin, U. Bakowsky and S. A. Fahmy, Recent Innovations of Mesoporous Silica Nanoparticles Combined with Photodynamic Therapy for Improving Cancer Treatment, Pharmaceutics, 2023, 16(1), 14 Search PubMed.
- S. Bayir, A. Barras, R. Boukherroub, S. Szunerits, L. Raehm, S. Richeter and J. O. Durand, Mesoporous silica nanoparticles in recent photodynamic therapy applications, Photochem. Photobiol. Sci., 2018, 17, 1651–1674 Search PubMed.
- M. Gary-Bobo, O. Hocine, D. Brevet, M. Maynadier, L. Raehm, S. Richeter, V. Charasson, B. Loock, A. Morère, P. Maillard and M. Garcia, Cancer therapy improvement with mesoporous silica nanoparticles combining targeting, drug delivery and PDT, Int. J. Pharm., 2012, 423(2), 509–515 Search PubMed.
- S. Li, Y. Zhang, X. W. He, W. Y. Li and Y. K. Zhang, Multifunctional mesoporous silica nanoplatform based on silicon nanoparticles for targeted two-photon-excited fluorescence imaging-guided chemo/photodynamic synergetic therapy in vitro, Talanta, 2020, 209, 120552 Search PubMed.
- X. Lin, M. Wu, M. Li, Z. Cai, H. Sun, X. Tan, J. Li, Y. Zeng, X. Liu and J. Liu, Photo-responsive hollow silica nanoparticles for light-triggered genetic and photodynamic synergistic therapy, Acta Biomater., 2018, 76, 178–192 CAS.
- D. Aggad, C. M. Jimenez, S. Dib, J. G. Croissant, L. Lichon, D. Laurencin, S. Richeter, M. Maynadier, S. K. Alsaiari, M. Boufatit and L. Raehm, Gemcitabine delivery and photodynamic therapy in cancer cells via porphyrin-ethylene-based periodic mesoporous organosilica nanoparticles, ChemNanoMat, 2018, 4(1), 46–51 Search PubMed.
- Ö. Er, S. G. Colak, K. Ocakoglu, M. Ince, R. Bresolí-Obach, M. Mora, M. L. Sagristá, F. Yurt and S. Nonell, Selective photokilling of human pancreatic cancer cells using cetuximab-targeted mesoporous silica nanoparticles for delivery of zinc phthalocyanine, Molecules, 2018, 23(11), 2749 Search PubMed.
- Q. Sun, Q. You, X. Pang, X. Tan, J. Wang, L. Liu, F. Guo, F. Tan and N. Li, A photoresponsive and rod-shape nanocarrier: Single wavelength of light triggered photothermal and photodynamic therapy based on AuNRs-capped & Ce6-doped mesoporous silica nanorods, Biomaterials, 2017, 122, 188–200 Search PubMed.
- Y. Zhou, C. Chang, Z. Liu, Q. Zhao, Q. Xu, C. Li, Y. Chen, Y. Zhang and B. Lu, Hyaluronic acid-functionalized hollow mesoporous silica nanoparticles as pH-sensitive nanocarriers for cancer chemo-photodynamic therapy, Langmuir, 2021, 37(8), 2619–2628 Search PubMed.
- D. Zhou, J. D. Piper, C. Abell, D. Klenerman, D. J. Kang and L. Ying, Fluorescence resonance energy transfer between a quantum dot donor and a dye acceptor attached to DNA, Chem. Commun., 2005,(38), 4807–4809 CAS.
- A. C. Samia, S. Dayal and C. Burda, Quantum dot-based energy transfer: perspectives and potential for applications in photodynamic therapy, Photochem. Photobiol., 2006, 82(3), 617–625 CrossRef CAS PubMed.
- A. R. Clapp, T. Pons, I. L. Medintz, J. B. Delehanty, J. S. Melinger, T. Tiefenbrunn, P. E. Dawson, B. R. Fisher, B. O'Rourke and H. Mattoussi, Two-photon excitation of quantum-dot-based fluorescence resonance energy transfer and its applications, Adv. Mater., 2007, 19(15), 1921–1926 Search PubMed.
- Z. D. Qi, D. W. Li, P. Jiang, F. L. Jiang, Y. S. Li, Y. Liu, W. K. Wong and K. W. Cheah, Biocompatible CdSe quantum dot-based photosensitizer under two-photon excitation for photodynamic therapy, J. Mater. Chem., 2011, 21(8), 2455–2458 Search PubMed.
- Y. Shen, Y. Sun, R. Yan, E. Chen, H. Wang, D. Ye, J. J. Xu and H. Y. Chen, Rational engineering of semiconductor QDs enabling remarkable 1O2 production for tumor-targeted photodynamic therapy, Biomaterials, 2017, 148, 31–40 Search PubMed.
- G. Murali, B. Kwon, H. Kang, J. K. Modigunta, S. Park, S. Lee, H. Lee, Y. H. Park, J. Kim, S. Y. Park and Y. J. Kim, Hematoporphyrin photosensitizer-linked carbon quantum dots for photodynamic therapy of cancer cells, ACS Appl. Nano Mater., 2022, 5(3), 4376–4385 Search PubMed.
- Q. Zeng, D. Shao, X. He, Z. Ren, W. Ji, C. Shan, S. Qu, J. Li, L. Chen and Q. Li, Carbon dots as a trackable drug delivery carrier for localized cancer therapy in vivo, J. Mater. Chem. B, 2016, 4(30), 5119–5126 RSC.
- A. Alaghmandfard, O. Sedighi, N. T. Rezaei, A. A. Abedini, A. M. Khachatourian, M. S. Toprak and A. Seifalian, Recent advances in the modification of carbon-based quantum dots for biomedical applications, Mater. Sci. Eng. C, 2021, 120, 111756 CrossRef CAS.
- J. Saleem, L. Wang and C. Chen, Carbon-based nanomaterials for cancer therapy via targeting tumor microenvironment, Adv. Healthcare Mater., 2018, 7(20), 1800525 CrossRef.
- T. Murakami, H. Nakatsuji, M. Inada, Y. Matoba, T. Umeyama, M. Tsujimoto, S. Isoda, M. Hashida and H. Imahori, Photodynamic and photothermal effects of semiconducting and metallic-enriched single-walled carbon nanotubes, J. Am. Chem. Soc., 2012, 134(43), 17862–17865 CrossRef CAS.
- L. Wang, J. Shi, R. Liu, Y. Liu, J. Zhang, X. Yu, J. Gao, C. Zhang and Z. Zhang, Photodynamic effect of functionalized single-walled carbon nanotubes: a potential sensitizer for photodynamic therapy, Nanoscale, 2014, 6(9), 4642–4651 RSC.
- M. Zhang, W. Wang, Y. Cui, X. Chu, B. Sun, N. Zhou and J. Shen, Magnetofluorescent Fe3O4/carbon quantum dots coated single-walled carbon nanotubes as dual-modal targeted imaging and chemo/photodynamic/photothermal triple-modal therapeutic agents, Chem. Eng. J., 2018, 338, 526–538 CrossRef CAS.
- Z. Li, F. L. Zhang, L. L. Pan, X. L. Zhu and Z. Z. Zhang, Preparation and characterization of injectable Mitoxantrone poly (lactic acid)/fullerene implants for in vivo chemo-photodynamic therapy, J. Photochem. Photobiol., B, 2015, 149, 51–57 CrossRef CAS PubMed.
- C. M. Lin and T. Y. Lu, C60 fullerene derivatized nanoparticles and their application to therapeutics, Recent Pat. Nanotechnol., 2012, 6(2), 105–113 CrossRef CAS.
- Q. Li, L. Hong, H. Li and C. Liu, Graphene oxide-fullerene C60 (GO-C60) hybrid for photodynamic and photothermal therapy triggered by near-infrared light, Biosens. Bioelectron., 2017, 89, 477–482 CrossRef CAS PubMed.
- Y. W. Chen, Y. L. Su, S. H. Hu and S. Y. Chen, Functionalized graphene nanocomposites for enhancing photothermal therapy in tumor treatment, Adv. Drug Delivery Rev., 2016, 105, 190–204 CrossRef CAS.
- J. Ge, M. Lan, B. Zhou, W. Liu, L. Guo, H. Wang, Q. Jia, G. Niu, X. Huang, H. Zhou and X. Meng, A graphene quantum dot photodynamic therapy agent with high singlet oxygen generation, Nat. Commun., 2014, 5(1), 4596 CrossRef CAS PubMed.
- W. S. Kuo, Y. T. Shao, K. S. Huang, T. M. Chou and C. H. Yang, Antimicrobial amino-functionalized nitrogen-doped graphene quantum dots for eliminating multidrug-resistant species in dual-modality photodynamic therapy and bioimaging under two-photon excitation, ACS Appl. Mater. Interfaces, 2018, 10(17), 14438–14446 CrossRef CAS.
- Y. Ding, L. Zhou, X. Chen, Q. Wu, Z. Song, S. Wei, J. Zhou and J. Shen, Mutual sensitization mechanism and self-degradation property of drug delivery system for in vitro photodynamic therapy, Int. J. Pharm., 2016, 498(1–2), 335–346 CrossRef CAS PubMed.
- D. Y. Zhang, Y. Zheng, C. P. Tan, J. H. Sun, W. Zhang, L. N. Ji and Z. W. Mao, Graphene oxide decorated with Ru (II)–polyethylene glycol complex for lysosome-targeted imaging and photodynamic/photothermal therapy, ACS Appl. Mater. Interfaces, 2017, 9(8), 6761–6771 CrossRef CAS PubMed.
- Y. Su, N. Wang, B. Liu, Y. Du, R. Li, Y. Meng, Y. Feng, Z. Shan and S. Meng, A phototheranostic nanoparticle for cancer therapy fabricated by BODIPY and graphene to realize photo-chemo synergistic therapy and fluorescence/photothermal imaging, Dyes Pigm., 2020, 177, 108262 CrossRef CAS.
- H. L. Cheng, H. L. Guo, A. J. Xie, Y. H. Shen and M. Z. Zhu, 4-in-1 Fe3O4/g-C3N4@ PPy-DOX nanocomposites: magnetic targeting guided trimode combinatorial chemotherapy/PDT/PTT for cancer, J. Inorg. Biochem., 2021, 215, 111329 CrossRef CAS.
- X. Qin, H. Zhang, Z. Wang and Y. Jin, Magnetic chitosan/graphene oxide composite loaded with novel photosensitizer for enhanced photodynamic therapy, RSC Adv., 2018, 8(19), 10376–10388 RSC.
- C. Murugan, V. Sharma, R. K. Murugan, G. Malaimegu and A. Sundaramurthy, Two-dimensional cancer theranostic nanomaterials: Synthesis, surface functionalization and applications in photothermal therapy, J. Controlled Release, 2019, 299, 1–20 CrossRef CAS PubMed.
- T. Fan, Z. Xie, W. Huang, Z. Li and H. Zhang, Two-dimensional non-layered selenium nanoflakes: facile fabrications and applications for self-powered photo-detector, Nanotechnology, 2019, 30(11), 114002 CrossRef CAS.
- J. N. Liu, W. Bu and J. Shi, Chemical design and synthesis of functionalized probes for imaging and treating tumor hypoxia, Chem. Rev., 2017, 117(9), 6160–6224 CrossRef CAS PubMed.
- Y. Chen, D. Ye, M. Wu, H. Chen, L. Zhang, J. Shi and L. Wang, Break-up of two-dimensional MnO2 nanosheets promotes ultrasensitive pH-triggered theranostics of cancer, Adv. Mater., 2014, 26(41), 7019–7026 CrossRef CAS.
- Y. Chen, H. Chen, S. Zhang, F. Chen, S. Sun, Q. He, M. Ma, X. Wang, H. Wu, L. Zhang and L. Zhang, Structure-property relationships in manganese oxide-mesoporous silica nanoparticles used for T1-weighted MRI and simultaneous anti-cancer drug delivery, Biomaterials, 2012, 33(7), 2388–2398 CrossRef CAS PubMed.
- H. Fan, G. Yan, Z. Zhao, X. Hu, W. Zhang, H. Liu, X. Fu, T. Fu, X. B. Zhang and W. Tan, A smart photosensitizer–manganese dioxide nanosystem for enhanced photodynamic therapy by reducing glutathione levels in cancer cells, Angew. Chem., Int. Ed., 2016, 55(18), 5477–5482 CrossRef CAS PubMed.
- D. Zeng, L. Wang, L. Tian, S. Zhao, X. Zhang and H. Li, Synergistic photothermal/photodynamic suppression of prostatic carcinoma by targeted biodegradable MnO2 nanosheets, Drug Delivery, 2019, 26(1), 661–672 CrossRef CAS.
- H. Chen, T. Liu, Z. Su, L. Shang and G. Wei, 2D transition metal dichalcogenide nanosheets for photo/thermo-based tumor imaging and therapy, Nanoscale Horiz., 2018, 3(2), 74–89 RSC.
- T. Liu, C. Wang, W. Cui, H. Gong, C. Liang, X. Shi, Z. Li, B. Sun and Z. Liu, Combined photothermal and photodynamic therapy delivered by PEGylated MoS 2 nanosheets, Nanoscale, 2014, 6(19), 11219–11225 RSC.
- S. Kapri and S. Bhattacharyya, Molybdenum sulfide–reduced graphene oxide p–n heterojunction nanosheets with anchored oxygen generating manganese dioxide nanoparticles for enhanced photodynamic therapy, Chem. Sci., 2018, 9(48), 8982–8989 RSC.
- L. S. Lin, Z. X. Cong, J. Li, K. M. Ke, S. S. Guo, H. H. Yang and G. N. Chen, Graphitic-phase C 3 N 4 nanosheets as efficient photosensitizers and pH-responsive drug nanocarriers for cancer imaging and therapy, J. Mater. Chem. B, 2014, 2(8), 1031–1037 RSC.
- Z. Chu, J. Liu, Z. Guo and H. Zhang, 2 μm passively Q-switched laser based on black phosphorus, Opt. Mater. Express, 2016, 6(7), 2374–2379 CrossRef CAS.
- D. Yang, G. Yang, P. Yang, R. Lv, S. Gai, C. Li, F. He and J. Lin, Assembly of Au plasmonic photothermal agent and iron oxide nanoparticles on ultrathin black phosphorus for targeted photothermal and photodynamic cancer therapy, Adv. Funct. Mater., 2017, 27(18), 1700371 CrossRef.
- J. Liu, P. Du, T. Liu, B. J. Wong, W. Wang, H. Ju and J. Lei, A black phosphorus/manganese dioxide nanoplatform: oxygen self-supply monitoring, photodynamic therapy enhancement and feedback, Biomaterials, 2019, 192, 179–188 CrossRef CAS PubMed.
- M. He, Y. Chen, C. Tao, Q. Tian, L. An, J. Lin, Q. Tian, H. Yang and S. Yang, Mn–porphyrin-based metal–organic framework with high longitudinal relaxivity for magnetic resonance imaging guidance and oxygen self-supplementing photodynamic therapy, ACS Appl. Mater. Interfaces, 2019, 11(45), 41946–41956 CrossRef CAS.
- K. Lu, T. Aung, N. Guo, R. Weichselbaum and W. Lin, Nanoscale metal–organic frameworks for therapeutic, imaging, and sensing applications, Adv. Mater., 2018, 30(37), 1707634 CrossRef.
- C. S. Jin, L. Cui, F. Wang, J. Chen and G. Zheng, Targeting-triggered porphysome nanostructure disruption for activatable photodynamic therapy, Adv. Healthcare Mater., 2014, 3(8), 1240–1249 CrossRef CAS PubMed.
- X. Li, C. Y. Kim, S. Lee, D. Lee, H. M. Chung, G. Kim, S. H. Heo, C. Kim, K. S. Hong and J. Yoon, Nanostructured phthalocyanine assemblies with protein-driven switchable photoactivities for biophotonic imaging and therapy, J. Am. Chem. Soc., 2017, 139(31), 10880–10886 CrossRef CAS.
- Y. Fu, X. Li, Z. Ren, C. Mao and G. Han, Multifunctional electrospun nanofibers for enhancing localized cancer treatment, Small, 2018, 14(33), 1801183 CrossRef.
- A. N. Severyukhina, N. V. Petrova, K. Smuda, G. S. Terentyuk, B. N. Klebtsov, R. Georgieva, H. Bäumler and D. A. Gorin, Photosensitizer-loaded electrospun chitosan-based scaffolds for photodynamic therapy and tissue engineering, Colloids Surf., B, 2016, 144, 57–64 CrossRef CAS PubMed.
- H. M. Wu, N. Chen, Z. M. Wu, Z. L. Chen and Y. J. Yan, Preparation of photosensitizer-loaded PLLA nanofibers and its anti-tumor effect for photodynamic therapy in vitro, J. Biomater. Appl., 2013, 27(6), 773–779 CrossRef PubMed.
- S. M. Costa, L. M. Lourenço, R. C. Calhelha, I. Calejo, C. C. Barrias, R. Fangueiro and D. P. Ferreira, Localized cancer photodynamic therapy approach based on core–shell electrospun nanofibers, Mater. Adv., 2024, 5(16), 6489–6500 RSC.
- L. M. Maestro, J. E. Ramirez-Hernandez, N. Bogdan, J. A. Capobianco, F. Vetrone, J. G. Solé and D. Jaque, Deep tissue bio-imaging using two-photon excited CdTe fluorescent quantum dots working within the biological window, Nanoscale, 2012, 4(1), 298–302 RSC.
- Q. Ju, X. Chen, F. Ai, D. Peng, X. Lin, W. Kong, P. Shi, G. Zhu and F. Wang, An upconversion nanoprobe operating in the first biological window, J. Mater. Chem. B, 2015, 3(17), 3548–3555 RSC.
- Y. A. Povrozin, L. I. Markova, A. L. Tatarets, V. I. Sidorov, E. A. Terpetschnig and L. D. Patsenker, Near-infrared, dual-ratiometric fluorescent label for measurement of pH, Anal. Biochem., 2009, 390(2), 136–140 CrossRef CAS PubMed.
- L. R. Hirsch, R. J. Stafford, J.
A. Bankson, S. R. Sershen, B. Rivera, R. E. Price, J. D. Hazle, N. J. Halas and J. L. West, Nanoshell-mediated near-infrared thermal therapy of tumors under magnetic resonance guidance, Proc. Natl. Acad. Sci. U. S. A., 2003, 100(23), 13549–13554 CrossRef CAS.
- M. I. Setyawati, C. Y. Tay, B. H. Bay and D. T. Leong, Gold nanoparticles induced endothelial leakiness depends on particle size and endothelial cell origin, ACS Nano, 2017, 11(5), 5020–5030 CrossRef CAS.
- P. K. Jain, K. S. Lee, I. H. El-Sayed and M. A. El-Sayed, Calculated absorption and scattering properties of gold nanoparticles of different size, shape, and composition: applications in biological imaging and biomedicine, J. Phys. Chem. B, 2006, 110(14), 7238–7248 CrossRef CAS.
- H. H. Richardson, Z. N. Hickman, A. O. Govorov, A. C. Thomas, W. Zhang and M. E. Kordesch, Thermooptical properties of gold nanoparticles embedded in ice: characterization of heat generation and melting, Nano Lett., 2006, 6(4), 783–788 CrossRef CAS.
- M. Manikandan, N. Hasan and H. F. Wu, Platinum nanoparticles for the photothermal treatment of Neuro 2A cancer cells, Biomaterials, 2013, 34(23), 5833–5842 CrossRef CAS PubMed.
- R. Jiang, S. Cheng, L. Shao, Q. Ruan and J. Wang, Mass-based photothermal comparison among gold nanocrystals, PbS nanocrystals, organic dyes, and carbon black, J. Phys. Chem. C, 2013, 117(17), 8909–8915 CrossRef CAS.
- S. Link and M. A. El-Sayed, Spectral properties and relaxation dynamics of surface plasmon electronic oscillations in gold and silver nanodots and nanorods, J. Phys. Chem. B, 1999, 103(40), 8410–8426 CrossRef CAS.
- C. M. Pitsillides, E. K. Joe, X. Wei, R. R. Anderson and C. P. Lin, Selective cell targeting with light-absorbing microparticles and nanoparticles, Biophys. J., 2003, 84(6), 4023–4032 CrossRef CAS.
- G. Mie, Beiträge zur Optik trüber Medien, speziell kolloidaler Metallösungen, Ann. Phys., 1908, 330(3), 377–445 CrossRef.
- M. F. Tsai, S. H. Chang, F. Y. Cheng, V. Shanmugam, Y. S. Cheng, C. H. Su and C. S. Yeh, Au nanorod design as light-absorber in the first and second biological near-infrared windows for in vivo photothermal therapy, ACS Nano, 2013, 7(6), 5330–5342 CrossRef CAS PubMed.
- R. Vankayala, C. C. Lin, P. Kalluru, C. S. Chiang and K. C. Hwang, Gold nanoshells-mediated bimodal photodynamic and photothermal cancer treatment using ultra-low doses of near infra-red light, Biomaterials, 2014, 35(21), 5527–5538 CrossRef CAS.
- S. Link, M. B. Mohamed and M. A. El-Sayed, Simulation of the optical absorption spectra of gold nanorods as a function of their aspect ratio and the effect of the medium dielectric constant, J. Phys. Chem. B, 1999, 103(16), 3073–3077 CrossRef CAS.
- P. Qiu, M. Yang, X. Qu, Y. Huai, Y. Zhu and C. Mao, Tuning photothermal properties of gold nanodendrites for in vivo cancer therapy within a wide near infrared range by simply controlling their degree of branching, Biomaterials, 2016, 104, 138–144 CrossRef CAS PubMed.
- K. Niikura, T. Matsunaga, T. Suzuki, S. Kobayashi, H. Yamaguchi, Y. Orba, A. Kawaguchi, H. Hasegawa, K. Kajino, T. Ninomiya and K. Ijiro, Gold nanoparticles as a vaccine platform: influence of size and shape on immunological responses in vitro and in vivo, ACS Nano, 2013, 7(5), 3926–3938 CrossRef CAS PubMed.
- M. S. Yavuz, Y. Cheng, J. Chen, C. M. Cobley, Q. Zhang, M. Rycenga, J. Xie, C. Kim, K. H. Song, A. G. Schwartz and L. V. Wang, Gold nanocages covered by smart polymers for controlled release with near-infrared light, Nat. Mater., 2009, 8(12), 935–939 CrossRef CAS.
- H. Liu, D. Chen, L. Li, T. Liu, L. Tan, X. Wu and F. Tang, Multifunctional gold nanoshells on silica nanorattles: a platform for the combination of photothermal therapy and chemotherapy with low systemic toxicity, Angew. Chem., Int. Ed., 2011, 4(50), 891–895 CrossRef PubMed.
- F. Rahimi-Moghaddam, N. Azarpira and N. Sattarahmady, Evaluation of a nanocomposite of PEG-curcumin-gold nanoparticles as a near-infrared photothermal agent: An in vitro and animal model investigation, Lasers Med. Sci., 2018, 33, 1769–1779 CrossRef CAS.
- S. Park, H. Kim, S. C. Lim, K. Lim, E. S. Lee, K. T. Oh, H. G. Choi and Y. S. Youn, Gold nanocluster-loaded hybrid albumin nanoparticles with fluorescence-based optical visualization and photothermal conversion for tumor detection/ablation, J. Controlled Release, 2019, 304, 7–18 CrossRef CAS.
- M. Wang, Y. Liu, X. Zhang, L. Luo, L. Li, S. Xing, Y. He, W. Cao, R. Zhu and D. Gao, Gold nanoshell coated thermo-pH dual responsive liposomes for resveratrol delivery and chemo-photothermal synergistic cancer therapy, J. Mater. Chem. B, 2017, 5(11), 2161–2171 RSC.
- F. Xia, J. Niu, Y. Hong, C. Li, W. Cao, L. Wang, W. Hou, Y. Liu and D. Cui, Matrix metallopeptidase 2 targeted delivery of gold nanostars decorated with IR-780 iodide for dual-modal imaging and enhanced photothermal/photodynamic therapy, Acta Biomater., 2019, 89, 289–299 CrossRef CAS.
- Y. Li, W. Lu, Q. Huang, C. Li and W. Chen, Copper sulfide nanoparticles for photothermal ablation of tumor cells, Nanomedicine, 2010, 5(8), 1161–1171 CrossRef CAS.
- J. T. Robinson, K. Welsher, S. M. Tabakman, S. P. Sherlock, H. Wang, R. Luong and H. Dai, High performance in vivo near-IR (> 1 μm) imaging and photothermal cancer therapy with carbon nanotubes, Nano Res., 2010, 3, 779–793 CrossRef CAS PubMed.
- Q. Tian, F. Jiang, R. Zou, Q. Liu, Z. Chen, M. Zhu, S. Yang, J. Wang, J. Wang and J. Hu, Hydrophilic Cu9S5 nanocrystals: a photothermal agent with a 25.7% heat conversion efficiency for photothermal ablation of cancer cells in vivo, ACS Nano, 2011, 5(12), 9761–9771 CrossRef CAS.
- B. Li, Q. Wang, R. Zou, X. Liu, K. Xu, W. Li and J. Hu, Cu 7.2 S 4 nanocrystals: a novel photothermal agent with a 56.7% photothermal conversion efficiency for photothermal therapy of cancer cells, Nanoscale, 2014, 6(6), 3274–3282 RSC.
- L. M. Peng, Z. Zhang, S. Wang and Y. Li, Carbon Nanotube (CNT)-Based High-Performance Electronic and Optoelectronic Devices. One-Dimensional Nanostructures: Principles and Applications, 2012, pp. 321–338 Search PubMed.
- X. Liu, N. Huang, H. Wang, H. Li, Q. Jin and J. Ji, The effect of ligand composition on the in vivo fate of multidentate poly (ethylene glycol) modified gold nanoparticles, Biomaterials, 2013, 34(33), 8370–8381 CrossRef CAS PubMed.
- A. R. Burke, R. N. Singh, D. L. Carroll, J. C. Wood, R. B. D'Agostino Jr, P. M. Ajayan, F. M. Torti and S. V. Torti, The resistance of breast cancer stem cells to conventional hyperthermia and their sensitivity to nanoparticle-mediated photothermal therapy, Biomaterials, 2012, 33(10), 2961–2970 CrossRef CAS.
- W. Zhang, Z. Guo, D. Huang, Z. Liu, X. Guo and H. Zhong, Synergistic effect of chemo-photothermal therapy using PEGylated graphene oxide, Biomaterials, 2011, 32(33), 8555–8561 CrossRef CAS PubMed.
- Z. M. Markovic, L. M. Harhaji-Trajkovic, B. M. Todorovic-Markovic, D. P. Kepić, K. M. Arsikin, S. P. Jovanović, A. C. Pantovic, M. D. Dramićanin and V. S. Trajkovic, In vitro comparison of the photothermal anticancer activity of graphene nanoparticles and carbon nanotubes, Biomaterials, 2011, 32(4), 1121–1129 CrossRef CAS PubMed.
- J. H. Lim, D. E. Kim, E. J. Kim, C. D. Ahrberg and B. G. Chung, Functional graphene oxide-based nanosheets for photothermal therapy, Macromol. Res., 2018, 26(6), 557–565 CrossRef CAS.
- M. Xie, F. Zhang, H. Peng, Y. Zhang, Y. Li, Y. Xu and J. Xie, Layer-by-layer modification of magnetic graphene oxide by chitosan and sodium alginate with enhanced dispersibility for targeted drug delivery and photothermal therapy, Colloids Surf., B, 2019, 176, 462–470 CrossRef CAS.
- A. Gulzar, J. Xu, D. Yang, L. Xu, F. He, S. Gai and P. Yang, Nano-graphene oxide-UCNP-Ce6 covalently constructed nanocomposites for NIR-mediated bioimaging and PTT/PDT combinatorial therapy, Dalton Trans., 2018, 47(11), 3931–3939 RSC.
- R. F. de Paula, I. A. Rosa, I. F. Gafanhão, J. L. Fachi, A. M. Melero, A. O. Roque, V. O. Boldrini, L. A. Ferreira, S. P. Irazusta, H. J. Ceragioli and E. C. de Oliveira, Reduced graphene oxide, but not carbon nanotubes, slows murine melanoma after thermal ablation using LED light in B16F10 lineage cells. Nanomedicine, Nanomedicine, 2020, 28, 102231 CrossRef CAS PubMed.
- I. Marangon, C. Ménard-Moyon, A. K. Silva, A. Bianco, N. Luciani and F. Gazeau, Synergic mechanisms of photothermal and photodynamic therapies mediated by photosensitizer/carbon nanotube complexes, Carbon, 2016, 97, 110–123 CrossRef CAS.
- J. Yang, J. Choi, D. Bang, E. Kim, E. K. Lim, H. Park, J. S. Suh, K. Lee, K. H. Yoo, E. K. Kim and Y. M. Huh, Convertible organic nanoparticles for near-infrared photothermal ablation of cancer cells, Angew. Chem., Int. Ed., 2011, 50(2), 441–444 CrossRef CAS PubMed.
- Z. Zhou, B. Kong, C. Yu, X. Shi, M. Wang, W. Liu, Y. Sun, Y. Zhang, H. Yang and S. Yang, Tungsten oxide nanorods: an efficient nanoplatform for tumor CT imaging and photothermal therapy, Sci. Rep., 2014, 4(1), 3653 CrossRef.
- S. M. Sharker, S. M. Kim, J. E. Lee, K. H. Choi, G. Shin, S. Lee, K. D. Lee, J. H. Jeong, H. Lee and S. Y. Park, Functionalized biocompatible WO3 nanoparticles for triggered and targeted in vitro and in vivo photothermal therapy, J. Controlled Release, 2015, 217, 211–220 CrossRef CAS PubMed.
- E. Vittorino, M. T. Sciortino, G. Siracusano and S. Sortino, Light-Activated Release of Nitric Oxide with Fluorescence Reporting in Living Cells, ChemMedChem, 2011, 6(9), 1551–1554 CrossRef CAS PubMed.
- M. Cheng, H. Wang, Z. Zhang, N. Li, X. Fang and S. Xu, Gold nanorod-embedded electrospun fibrous membrane as a photothermal therapy platform, ACS Appl. Mater. Interfaces, 2014, 6(3), 1569–1575 CrossRef CAS.
- X. Wang, L. Wang, S. Zong, R. Qiu and S. Liu, Use of multifunctional composite nanofibers for photothermalchemotherapy to treat cervical cancer in mice, Biomater. Sci., 2019, 7(9), 3846–3854 RSC.
- M. H. Azerbaijan, E. Bahmani, M. H. Jouybari, A. Hassaniazardaryani, P. Goleij, M. Akrami and M. Irani, Electrospun gold nanorods/graphene oxide loaded-core-shell nanofibers for local delivery of paclitaxel against lung cancer during photo-chemotherapy method, Eur. J. Pharm. Sci., 2021, 164, 105914 CrossRef CAS.
- S. Veeranarayanan and T. Maekawa, External stimulus responsive inorganic nanomaterials for cancer theranostics, Adv. Drug Delivery Rev., 2019, 138, 18–40 CrossRef.
- A. Sneider, D. VanDyke, S. Paliwal and P. Rai, Remotely triggered nano-theranostics for cancer applications, Nanotheranostics, 2017, 1(1), 1 CrossRef PubMed.
- Q. Chen, H. Ke, Z. Dai and Z. Liu, Nanoscale theranostics for physical stimulus-responsive cancer therapies, Biomaterials, 2015, 73, 214–230 CrossRef CAS.
- Y. Chen, H. Li, Y. Deng, H. Sun, X. Ke and T. Ci, Near-infrared light triggered drug delivery system for higher efficacy of combined chemo-photothermal treatment, Acta Biomater., 2017, 51, 374–392 CrossRef CAS PubMed.
- P. Manivasagan, S. W. Jun, G. Hoang, S. Mondal, H. Kim, V. H. Doan, J. Kim, C. S. Kim and J. Oh, Anti-EGFR antibody conjugated thiol chitosan-layered gold nanoshells for dual-modal imaging-guided cancer combination therapy, J. Controlled Release, 2019, 311, 26–42 CrossRef.
- W. Zhang, F. Wang, Y. Wang, J. Wang, Y. Yu, S. Guo, R. Chen and D. Zhou, pH and near-infrared light dual-stimuli responsive drug delivery using DNA-conjugated gold nanorods for effective treatment of multidrug resistant cancer cells, J. Controlled Release, 2016, 232, 9–19 CrossRef CAS.
- S. Huang, S. Duan, J. Wang, S. Bao, X. Qiu, C. Li, Y. Liu, L. Yan, Z. Zhang and Y. Hu, Folic-acid-mediated functionalized gold nanocages for targeted delivery of anti-miR-181b in combination of gene therapy and photothermal therapy against hepatocellular carcinoma, Adv. Funct. Mater., 2016, 26(15), 2532–2544 CrossRef CAS.
- D. Wang, Y. Ren, Y. Shao, D. Yu and L. Meng, Facile preparation of doxorubicin-loaded and folic acid-conjugated carbon nanotubes@ poly (N-vinyl pyrrole) for targeted synergistic chemo–Photothermal Cancer treatment, Bioconjugate Chem., 2017, 28(11), 2815–2822 CrossRef CAS PubMed.
- R. Lima-Sousa, D. de Melo-Diogo, C. G. Alves, E. C. Costa, P. Ferreira, R. O. Louro and I. J. Correia, Hyaluronic acid functionalized green reduced graphene oxide for targeted cancer photothermal therapy, Carbohydr. Polym., 2018, 200, 93–99 CrossRef CAS.
- S. Roy, A. Sarkar and A. Jaiswal, Poly (allylamine hydrochloride)-functionalized reduced graphene oxide for synergistic chemo-photothermal therapy, Nanomedicine, 2019, 14(3), 255–274 CrossRef CAS.
- K. Wang, Q. Chen, W. Xue, S. Li and Z. Liu, Combined chemo-photothermal antitumor therapy using molybdenum disulfide modified with hyperbranched polyglycidyl, ACS Biomater. Sci. Eng., 2017, 3(10), 2325–2335 CrossRef CAS PubMed.
- T. Bao, W. Yin, X. Zheng, X. Zhang, J. Yu, X. Dong, Y. Yong, F. Gao, L. Yan, Z. Gu and Y. Zhao, One-pot synthesis of PEGylated plasmonic MoO3–x hollow nanospheres for photoacoustic imaging guided chemo-photothermal combinational therapy of cancer, Biomaterials, 2016, 76, 11–24 CrossRef CAS PubMed.
- R. M. Yang, C. P. Fu, J. Z. Fang, X. D. Xu, X. H. Wei, W. J. Tang, X. Q. Jiang and L. M. Zhang, Hyaluronan-modified superparamagnetic iron oxide nanoparticles for bimodal breast cancer imaging and photothermal therapy, Int. J. Nanomed., 2017, 23, 197–206 Search PubMed.
- L. Hou, X. Shan, L. Hao, Q. Feng and Z. Zhang, Copper sulfide nanoparticle-based localized drug delivery system as an effective cancer synergistic treatment and theranostic platform, Acta Biomater., 2017, 54, 307–320 CrossRef CAS PubMed.
- Y. Ma, X. Liang, S. Tong, G. Bao, Q. Ren and Z. Dai, Gold nanoshell nanomicelles for potential magnetic resonance imaging, light-triggered drug release, and photothermal therapy, Adv. Funct. Mater., 2013, 23(7), 815–822 CrossRef CAS.
- M. Bañobre-López, A. Teijeiro and J. Rivas, Magnetic nanoparticle-based hyperthermia for cancer treatment, Rep. Pract. Oncol. Radiother., 2013, 18(6), 397–400 CrossRef PubMed.
- Z. Hedayatnasab, F. Abnisa and W. M. Daud, Review on magnetic nanoparticles for magnetic nanofluid hyperthermia application, Mater. Des., 2017, 123, 174–196 CrossRef CAS.
- M. Angelakeris, Magnetic nanoparticles: A multifunctional vehicle for modern theranostics, Biochim. Biophys. Acta - Gen. Subj., 2017, 1861(6), 1642–1651 CrossRef CAS.
- A. Matsumine, K. Takegami, K. Asanuma, T. Matsubara, T. Nakamura, A. Uchida and A. Sudo, A novel hyperthermia treatment for bone metastases using magnetic materials, Int. J. Clin. Oncol., 2011, 16, 101–108 CrossRef.
- Y. Ni, S. Mulier, Y. Miao, L. Michel and G. Marchal, A review of the general aspects of radiofrequency ablation, Abdom. Imaging, 2005, 30, 381–400 CrossRef CAS.
- A. A. Elsherbini, M. Saber, M. Aggag, A. El-Shahawy and H. A. Shokier, Laser and radiofrequency-induced hyperthermia treatment via gold-coated magnetic nanocomposites, Int. J. Nanomed., 2011, 28, 2155–2165 CrossRef PubMed.
- Q. Zhang and L. Li, Photodynamic combinational therapy in cancer treatment, J. BUON., 2018, 23(3), 561–567 Search PubMed.
- M. Faber, C. Coudray, H. Hida, M. Mousseau and A. Favier, Lipid peroxidation products, and vitamin and trace element status in patients with cancer before and after chemotherapy, including adriamycin: a preliminary study, Biol. Trace Elem. Res., 1995, 47, 117–123 CrossRef CAS.
- G. Canti, A. Nicolin, R. Cubeddu, P. Taroni, G. Bandieramonte and G. Valentini, Antitumor efficacy of the combination of photodynamic therapy and chemotherapy in murine tumors, Cancer Lett., 1998, 125(1–2), 39–44 CrossRef CAS PubMed.
- X. Wang, G. Meng, S. Zhang and X. Liu, A reactive 1O2-responsive combined treatment system of photodynamic and chemotherapy for cancer, Sci. Rep., 2016, 6(1), 1–9 CrossRef PubMed.
- A. Khdair, D. Chen, Y. Patil, L. Ma, Q. P. Dou, M. P. Shekhar and J. Panyam, Nanoparticle-mediated combination chemotherapy and photodynamic therapy overcomes tumor drug resistance, J. Controlled Release, 2010, 141(2), 137–144 CrossRef CAS PubMed.
- S. Bano, S. Nazir, S. Munir, M. F. AlAjmi, M. Afzal and K. Mazhar, “Smart” nickel oxide based core–shell nanoparticles for combined chemo and photodynamic cancer therapy, Int. J. Nanomed., 2016, 12, 3159–3166 Search PubMed.
- A. Nakano, D. Watanabe, Y. Akita, T. Kawamura, Y. Tamada and Y. Matsumoto, Treatment efficiency of combining photodynamic therapy and ionizing radiation for Bowen's disease, J. Eur. Acad. Dermatol. Venereol., 2011, 25(4), 475–478 CrossRef CAS PubMed.
- J. Takahashi, M. Misawa and H. Iwahashi, Transcriptome analysis of porphyrin-accumulated and X-ray-irradiated cell cultures under limited proliferation and non-lethal conditions, Microarrays, 2015, 4(1), 25–40 CrossRef CAS PubMed.
- M. Mitsunaga, T. Nakajima, K. Sano, P. L. Choyke and H. Kobayashi, Near-infrared theranostic photoimmunotherapy (PIT): repeated exposure of light enhances the effect of immunoconjugate, Bioconjugate Chem., 2012, 23(3), 604–609 CrossRef.
- C. He, X. Duan, N. Guo, C. Chan, C. Poon, R. R. Weichselbaum and W. Lin, Core-shell nanoscale coordination polymers combine chemotherapy and photodynamic therapy to potentiate checkpoint blockade cancer immunotherapy, Nat. Commun., 2016, 7(1), 12499 CrossRef CAS.
- X. Zheng, D. Xing, F. Zhou, B. Wu and W. R. Chen, Indocyanine green-containing nanostructure as near infrared dual-functional targeting probes for optical imaging and photothermal therapy, Mol. Pharmaceutics, 2011, 8(2), 447–456 CrossRef CAS.
- X. Zheng, F. Zhou, B. Wu, W. R. Chen and D. Xing, Enhanced tumor treatment using biofunctional indocyanine green-containing nanostructure by intratumoral or intravenous injection, Mol. Pharmaceutics, 2012, 9(3), 514–522 CrossRef CAS.
- M. Korbelik and I. Cecic, Enhancement of tumour response to photodynamic therapy by adjuvant mycobacterium cell-wall treatment, J. Photochem. Photobiol., B, 1998, 44(2), 151–158 CrossRef CAS.
- S. Wu and H. J. Butt, Near-infrared photochemistry at interfaces based on upconverting nanoparticles, Phys. Chem. Chem. Phys., 2017, 19(35), 23585–23596 RSC.
- Y. Li, D. He, J. Tu, R. Wang, C. Zu, Y. Chen, W. Yang, D. Shi, T. J. Webster and Y. Shen, The comparative effect of wrapping solid gold nanoparticles and hollow gold nanoparticles with doxorubicin-loaded thermosensitive liposomes for cancer thermo-chemotherapy, Nanoscale, 2018, 10(18), 8628–8641 RSC.
- Z. Meng, F. Wei, R. Wang, M. Xia, Z. Chen, H. Wang and M. Zhu, NIR-laser-switched in vivo smart nanocapsules for synergic photothermal and chemotherapy of tumors, Adv. Mater., 2016, 28(2), 245–253 CrossRef CAS PubMed.
- Y. Liu, Y. Xi, J. Zhao, J. Zhao, J. Li, G. Huang, J. Li, F. Fang, L. Gu and S. Wang, Preparation of therapeutic-laden konjac hydrogel for tumor combination therapy, Chem. Eng. J., 2019, 375, 122048 CrossRef CAS.
- Y. Zheng, W. Wang, J. Zhao, C. Wu, C. Ye, M. Huang and S. Wang, Preparation of injectable temperature-sensitive chitosan-based hydrogel for combined hyperthermia and chemotherapy of colon cancer, Carbohydr. Polym., 2019, 222, 115039 CrossRef CAS PubMed.
- V. P. Chauhan, T. Stylianopoulos, Y. Boucher and R. K. Jain, Delivery of molecular and nanoscale medicine to tumors: transport barriers and strategies, Annu. Rev. Chem. Biomol. Eng., 2011, 2(1), 281–298 CrossRef CAS.
- R. K. Jain, J. D. Martin and T. Stylianopoulos, The role of mechanical forces in tumor growth and therapy, Annu. Rev. Biomed. Eng., 2014, 16(1), 321–346 CrossRef CAS PubMed.
- Y. Zheng, F. Zou, J. Wang, G. Yin, V. Le, Z. Fei and J. Liu, Photodynamic therapy-mediated cancer vaccination enhances stem-like phenotype and immune escape, which can be blocked by thrombospondin-1 signaling through CD47 receptor protein, J. Biol. Chem., 2015, 290(14), 8975–8986 CrossRef CAS.
- Y. Liu, Y. Liu, W. Bu, Q. Xiao, Y. Sun, K. Zhao, W. Fan, J. Liu and J. Shi, Radiation-/hypoxia-induced solid tumor metastasis and regrowth inhibited by hypoxia-specific upconversion nanoradiosensitizer, Biomaterials, 2015, 49, 1–8 CrossRef CAS PubMed.
- L. Cheng, S. Shen, S. Shi, Y. Yi, X. Wang, G. Song, K. Yang, G. Liu, T. E. Barnhart, W. Cai and Z. Liu, FeSe2-decorated Bi2Se3 nanosheets fabricated via cation exchange for chelator-free 64Cu-labeling and multimodal image-guided photothermal-radiation therapy, Adv. Funct. Mater., 2016, 26(13), 2185–2197 CrossRef CAS PubMed.
- X. Yi, K. Yang, C. Liang, X. Zhong, P. Ning, G. Song, D. Wang, C. Ge, C. Chen, Z. Chai and Z. Liu, Imaging-guided
combined photothermal and radiotherapy to treat subcutaneous and metastatic tumors using iodine-131-doped copper sulfide nanoparticles, Adv. Funct. Mater., 2015, 25(29), 4689–4699 CrossRef CAS.
- W. Y. Sheng and L. Huang, Cancer immunotherapy and nanomedicine, Pharm. Res., 2011, 28, 200–214 CrossRef CAS.
- X. Dong, J. Liang, A. Yang, Z. Qian, D. Kong and F. Lv, Fluorescence imaging guided CpG nanoparticles-loaded IR820-hydrogel for synergistic photothermal immunotherapy, Biomaterials, 2019, 209, 111–125 CrossRef CAS PubMed.
- Y. Tao, E. Ju, J. Ren and X. Qu, Immunostimulatory oligonucleotides-loaded cationic graphene oxide with photothermally enhanced immunogenicity for photothermal/immune cancer therapy, Biomaterials, 2014, 35(37), 9963–9971 CrossRef CAS.
- S. Wang, P. Huang, L. Nie, R. Xing, D. Liu, Z. Wang, J. Lin, S. Chen, G. Niu, G. Lu and X. Chen, Single continuous wave laser induced photodynamic/plasmonic photothermal therapy using photosensitizer-functionalized gold nanostars, Adv. Mater., 2013, 25(22), 3055 CrossRef CAS PubMed.
- H. P. Tham, H. Chen, Y. H. Tan, Q. Qu, S. Sreejith, L. Zhao, S. S. Venkatraman and Y. Zhao, Photosensitizer anchored gold nanorods for targeted combinational photothermal and photodynamic therapy, Chem. Commun., 2016, 52(57), 8854–8857 RSC.
- P. Kalluru, R. Vankayala, C. S. Chiang and K. C. Hwang, Nano-graphene oxide-mediated In vivo fluorescence imaging and bimodal photodynamic and photothermal destruction of tumors, Biomaterials, 2016, 95, 1 CrossRef CAS.
- Y. Wang, H. Wang, D. Liu, S. Song, X. Wang and H. Zhang, Graphene oxide covalently grafted upconversion nanoparticles for combined NIR mediated imaging and photothermal/photodynamic cancer therapy, Biomaterials, 2013, 34(31), 7715–7724 CrossRef CAS.
- M. Overchuk, R. A. Weersink, B. C. Wilson and G. Zheng, Photodynamic and photothermal therapies: synergy opportunities for nanomedicine, ACS Nano, 2023, 17(9), 7979–8003 CrossRef CAS.
- J. Kadkhoda, A. Tarighatnia, J. Barar, A. Aghanejad and S. Davaran, Recent advances and trends in nanoparticles based photothermal and photodynamic therapy, Photodiagn. Photodyn. Ther., 2022, 37, 102697 CrossRef CAS.
- I. Rizvi, S. Nath, G. Obaid, M. K. Ruhi, K. Moore, S. Bano, D. Kessel and T. Hasan, A combination of visudyne and a lipid-anchored liposomal formulation of benzoporphyrin derivative enhances photodynamic therapy efficacy in a 3D model for ovarian cancer, Photochem. Photobiol., 2019, 95(1), 419–429 CrossRef CAS PubMed.
|
This journal is © The Royal Society of Chemistry 2024 |
Click here to see how this site uses Cookies. View our privacy policy here.