DOI:
10.1039/D4RA07295B
(Paper)
RSC Adv., 2024,
14, 39833-39843
Microwave-assisted synthesis of base-modified fluorescent 1,4-dihydropyridine nucleosides: photophysical characterization and insights†
Received
11th October 2024
, Accepted 12th December 2024
First published on 18th December 2024
Abstract
A synthesis of a small library of fluorescent 1,4-dihydropyridine nucleoside analogues has been successfully carried out under solvent-free conditions via a one-pot three-component Hantzsch condensation reaction. The process involved a Ba(NO3)2 catalyzed solvent-free reaction between 3′,5′-di-O-acetyl-5-formyl-2′-deoxyuridine, differently substituted β-keto ester and ammonium acetate under microwave irradiation. This facile methodology yielded the desired products with very high yields (86–96%) under solvent-free reaction conditions in a short reaction time, which was followed by a simple workup. Yields obtained under microwave and conventional heating were compared, with the microwave irradiation condition displaying superior results. The synthesized compounds were characterized by IR, 1H-NMR, 13C-NMR, 1H–1H COSY, 1H–13C HETCOR, 2D NOESY NMR and HRMS analysis. These nucleoside analogues exhibited significant fluorescence, with a prominent emission band around 460 nm (excitation at 235 nm). Photophysical studies revealed strong fluorescence intensity, excellent Stokes shifts (70–162 nm), and high quantum yields (0.022–0.579), outperforming other pyrimidine-based fluorescent nucleosides. Notably, 5-(diethyl 2′′,6′′-propyl-1′′,4′′-dihydropyridine-3′′,5′′-dicarboxylate)-4′′-yl-2′-deoxyuridine demonstrated a quantum yield as high as 0.579 in DMSO during solvatochromic studies, highlighting their potential for probing local nucleic acid structure and dynamics. Additionally, we demonstrated the scalability of the synthesis protocol by producing one of the compounds on a gram scale, confirming its practical viability for large-scale production. This study underscores the potential of these fluorescent nucleoside analogues for various biochemical applications.
1. Introduction
DNA, the “molecule of life”, stores genetic instructions vital for biological processes such as cell division and viral replication.1 DNA's structure is constituted by nucleotides, which contains nucleobase, carbohydrate and phosphate backbone, whereas nucleoside monomers are composed of a nucleobase and carbohydrate moiety. Over recent decades, chemists have synthesized numerous modified nucleosides to evaluate their biological activities, including antiviral,2–5 anti-HIV,6,7 anticancer,8,9 antimetabolites,10,11 antisense properties12 and many more.13,14 The worldwide clinical success of these nucleosides inspires scientists to advance the synthesis of modified nucleosides (Fig. 1).15–20 Among the modified nucleosides, the synthesis of modified fluorescent nucleoside analogues has undergone rapid growth due to the non-emissive nature of the canonical bases in nucleic acids.21
 |
| Fig. 1 Structures of some FDA approved sugar modified nucleosides. | |
Several base analogues, such as 2-aminopurine, pyrrolo-dC (pyrrolo-deoxycytidine), 1,3-diaza-2-oxophenoxazine (tCO), bodipy-labelled bases, and cyanine-labelled bases, which possess intrinsic fluorescence or can be chemically modified to exhibit fluorescent properties, have been used in the synthesis of fluorescent nucleosides.22–25 When fluorescent nucleobases are incorporated synthetically into DNA and RNA, they serve as potent tools, providing new insights into DNA and RNA and leading to advancements in chemistry, biology, and medicine.26 The fascination with base-modified fluorescent nucleosides lies in their exceptional sensitivity to the microenvironment. This characteristic feature positions them as potent tools for delving into the intricate structure and functions of nucleic acids, offering promising avenues for further research and applications.27–30
In the last few decades, structural modifications at the fifth position of the uracil base of uridine have gained attention for exploration of their photophysical properties (Fig. 2).21,31–43 Modifications at this position exhibited Watson–Crick type hydrogen bonding as similar as their native counterparts.44 Additionally, 5-substituted 2′-deoxyuridines are known for their antiviral activity against a range of viruses, including Herpes simplex virus (HSV-1 and HSV-2),45 Hepatitis B virus (HBV),46 Vaccinia virus (VV),47 Varicella-zoster virus (VZV),48 and Cowpox virus (CV)46 and these nucleoside monomers have also demonstrated notable antibacterial activities.46,49,50
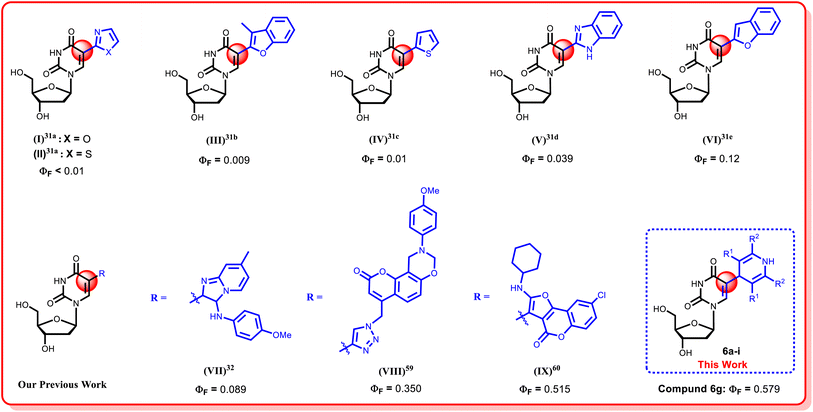 |
| Fig. 2 Some representative base modified fluorescent nucleosides. | |
Over the decades, our research group has developed numerous facile and fruitful methodologies to synthesize sugar-modified or nucleobase modified nucleosides.32,51–60 Recently, we have focused our attention on modifying the C-5 position of the uracil base of uridine by introducing different heterocyclic functionalities at that position to develop nucleosides with enhanced fluorescence properties (Fig. 2).32,59,60 As part of our ongoing efforts to enhance the fluorescence properties of the uridine moiety, a green methodology to incorporate 1,4-dihydropyridine (DHP) at the C-5 position of uridine has been reported herein. 1,4-Dihydropyridines (DHPs) stand out as crucial heterocyclic compounds with diverse pharmacological properties, commonly synthesized through the Hantzsch reaction,61 which serves as a privileged pharmacophore in organic chemistry.62 Additionally, the photophysical characteristics63–66 of 1,4-dihydropyridine derivatives have been extensively investigated and documented in the literature. However, to the best of our knowledge, the incorporation of DHP moieties into the nucleoside chemistry has not been explored till date. In this article, we are reporting an environmentally friendly, efficient and solvent-free reaction to incorporate the 1,4-dihydropyridine moiety at the C-5 position of uridine for the first time via microwave irradiation. This research work includes the comparative studies of the conventional heating methodology and microwave irradiation procedures, where the later one provided an excellent solution as a non-conventional energy source, offering uniform internal heating and significantly reducing the reaction time, followed by an easier workup.67 A comprehensive study of the photophysical properties of the synthesized nucleoside analogues is also included in this article.
2. Results and discussion
2.1. Chemistry
The primary goal of this research work was to incorporate 1,4-dihydropyridines into the uridine moiety. For this purpose, a one-pot, three-component, Hantzsch condensation reaction was attempted with 3′,5′-di-O-acetyl-5-formyl-2′-deoxyuridine (3) under both conventional as well as microwave heating conditions (Scheme 1).
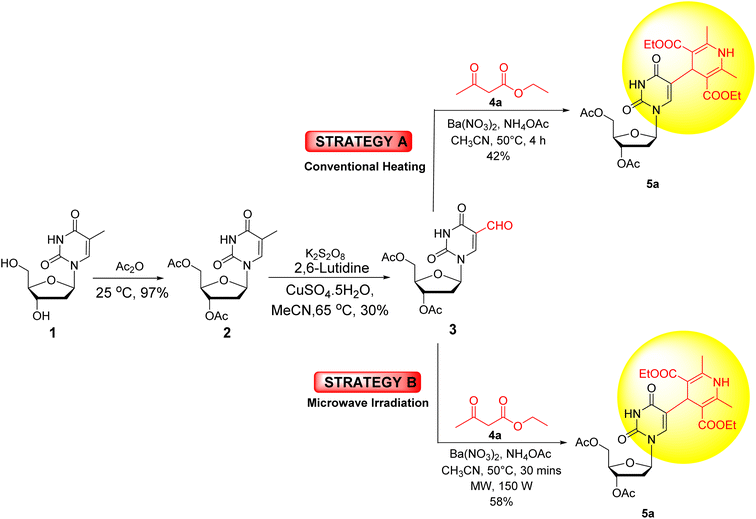 |
| Scheme 1 Method A: conventional Heating; Method B: microwave Irradiation. | |
At the outset, thymidine 1 was acetylated with acetic anhydride to produce 3′,5′-di-O-acetylthymidine (2). Subsequent oxidation with potassium persulfate, 2,6-lutidine, and cupric sulfate in acetonitrile yielded 3′,5′-di-O-acetyl-5-formyl-2′-deoxyuridine (3).68 Initially, both microwave and conventional methods were used for synthesizing the target compound 5a starting from nucleoside 3. In Method A (conventional heating), 3′,5′-di-O-acetyl-5-formyl-2′-deoxyuridine (3) was treated with ethyl acetoacetate (4a) and ammonium acetate in the presence of Ba(NO3)2 as a catalyst in CH3CN at 50 °C. It yielded the desired compound 5a in 42% yield. Whereas, in Method B (microwave heating), under identical reaction conditions, 3′,5′-di-O-acetyl-5-formyl-2′-deoxyuridine (3) produced the desired product 5a in 58% yield in 30 minutes (Scheme 1).
After establishing the superiority of microwave heating over conventional heating for the synthesis of our desired compound 5a, optimization of the reaction conditions was carried out (Table 1). Initially, performing the reaction in acetonitrile (CH3CN) at 50 °C yielded the desired 1,4-dihydropyridine nucleoside 5a in 58% yield in 30 minutes (entry 1, Table 1). Next, various solvents, such as 1,4-dioxane, MeOH, ethanol, THF, AcOH, EtOAc, H2O, DMF, isopropyl alcohol, and toluene (entries 2–11, Table 1), were screened. The higher yield of 72% was obtained in 1,4-dioxane, while other solvents resulted in inferior yields. Interestingly, significant improvement in the yield was achieved under solvent-free reaction conditions, with 86% yield at 50 °C in just 20 minutes (entry 12, Table 1). This outcome encouraged us to conduct the next reactions under solvent free reaction conditions. Hence, the following reactions were carried out at different temperatures, where the highest yield (93%) was obtained at 60 °C (entry 13, Table 1). Higher temperatures like 70 °C, 80 °C, 100 °C, and 120 °C resulted in relatively inferior yields of 88%, 68%, 32%, and 25%, respectively (entries 14–17, Table 1). When the reaction was performed in the absence of catalyst Ba(NO3)2 under solvent-free conditions at 60 °C for 30 minutes under microwave irradiation, the reaction remained incomplete, yielding the desired product 5a in 20% yield only (entry 18, Table 1). Among the conditions explored, microwave irradiation under solvent-free conditions at 60 °C was found to be the most favourable for synthesizing the 1,4-dihydropyridine nucleoside analogue 5a (entry 13, Table 1).
Table 1 Optimization of the reaction of 3′,5′-di-O-acetyl-5-formyl-2′-deoxyuridine 3 with ethyl acetoacetate 4a and ammonium acetate in the presence of Ba(NO3)2 as a catalyst to obtain 1,4-dihydropyridine nucleoside analogue 5aad
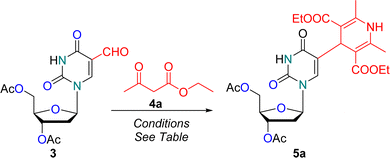
|
S. no. |
Solvent |
Temperature (°C) |
Time (min) |
Reaction yield (percent yield)b |
Reaction conditions: 3′,5′-di-O-acetyl-5-formyl-2′-deoxyuridine 3 (0.34 g, 1 mmol), ethyl acetoacetate (0.39 g, 3 mmol), NH4OAc (92.3 mg, 1.2 mmol) and barium nitrate (26.2 mg, 0.1 mmol). Isolated yield. Without catalyst: reaction remained incomplete within 30 min and only 20 percent of the final product was obtained. When we attempted to extend the optimized reaction conditions to synthesize unsymmetrical DHP using 3′,5′-di-O-acetyl-5-formyl-2′-deoxyuridine 3 (0.34 g, 1 mmol), ethyl acetoacetate (0.13 g, 1 mmol), allyl acetoacetate (0.14 g, 1 mmol), NH4OAc (92.3 mg, 1.2 mmol) and barium nitrate (26.2 mg, 0.1 mmol), the reaction produced a un-separable diastereomeric mixture, as indicated by un-separable spots on TLC. Unfortunately, we were unable to separate these diastereomeric mixture product (see ESI, Fig. S43 and S44) using column chromatography. |
1 |
Acetonitrile |
50 |
30 |
58 |
2 |
1,4-Dioxane |
50 |
30 |
72 |
3 |
MeOH |
50 |
30 |
62 |
4 |
EtOH |
50 |
30 |
54 |
5 |
THF |
50 |
30 |
46 |
6 |
AcOH |
50 |
30 |
38 |
7 |
EtOAc |
50 |
30 |
40 |
8 |
H2O |
50 |
30 |
44 |
9 |
DMF |
50 |
30 |
35 |
10 |
i-PrOH |
50 |
30 |
38 |
11 |
Toluene |
50 |
30 |
65 |
12 |
Solvent-free |
50 |
20 |
86 |
13 |
Solvent-free |
60 |
20 |
93 |
14 |
Solvent-free |
70 |
20 |
88 |
15 |
Solvent-free |
80 |
20 |
68 |
16 |
Solvent-free |
100 |
20 |
32 |
17 |
Solvent-free |
120 |
20 |
25 |
18 |
Solvent-free |
60 |
30 |
20c |
The optimized reaction condition was employed for the condensation of 3′,5′-di-O-acetyl-5-formyl-2′-deoxyuridine 3 with eight differently substituted β-keto ester 4a–h or acetyl acetone 4i in the presence of NH4OAc, yielding base-modified 1,4-dihydropyridine nucleoside analogues 5a–i in 86–96% yields (Scheme 2). Deacetylation of the diacetylated nucleosides 5a–i was performed using NaOMe in MeOH at 25 °C, resulting in the desired 1,4-dihydropyridine nucleoside analogues 6a–i in quantitative yields (Scheme 2).
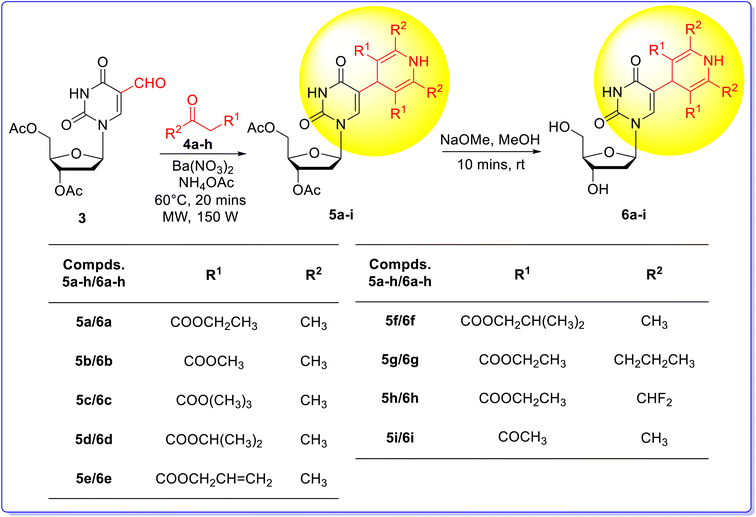 |
| Scheme 2 Synthesis of fluorescent 1,4-dihydropyridine nucleoside analogues. | |
The structures of all the synthesized compounds, including 3′,5′-di-O-acetyl-thymidine 2, 3′,5′-di-O-acetyl-5-formyl-2′-deoxyuridine 3, base modified 1,4-dihydropyridine nucleoside analogues 5a–i and 6a–i, were unambiguously established by spectral data analysis (IR, 1H, 13C-NMR, 1H–1H COSY NMR, 1H–13C HETCOR NMR, NOESY NMR, and HRMS). The structures of the known compounds 2 and 3 were confirmed by comparing their melting point and spectral data with literature reports.32,59,60
2.2. Gram scale synthesis of 3′,5′-di-O-acetyl-5-(diethyl 2′′,6′′-dimethyl 1′′,4′′-dihydropyridine-3′′,5′′-dicarboxylate)-2′-deoxyuridine (5a)
To showcase the applicability and broaden the applicative range of our methodology, we carried out the gram scale synthesis of 3′,5′-di-O-acetyl-5-(diethyl 2′′,6′′-dimethyl-1′′,4′′-dihydropyridine-3′′,5′′-dicarboxylate)-2′-deoxyuridine 5a, which highlights the practicality and effectiveness of the methodology for large-scale reactions. A reaction mixture comprising of 3′,5′-di-O-acetyl-5-formyl-2′-deoxyuridine 3 (1.02 g, 3 mmol), ethyl acetoacetate 4a (1.17 g, 9 mmol), NH4OAc (0.277 g, 3.6 mmol) and Ba(NO3)2 (78.5 mg, 0.3 mmol) was subjected to microwave irradiation under solvent-free conditions. The reaction proceeded smoothly resulting in 86% yield (1.45 g), highlighting the efficacy of this protocol for large-scale reactions.
3. Photophysical characterization
Over the last few decades, several modified nucleosides with fluorescent properties have been synthesized and studied for their application for studying the structure and dynamics of nucleic acid. The photophysical characterization of the thus synthesized 5-(1′′,4′′-dihydropyridine)-2′-deoxyuridine nucleoside analogues 6a–i, consisting of a highly extended conjugation system, was carried out in acetonitrile at 2.5 × 10−5 M concentration.
3.1. Absorption spectra
The absorption spectra of the synthesized 5-(1′′,4′′-dihydropyridine)-2′-deoxyuridine nucleoside analogues 6a–i was recorded in acetonitrile at 2.5 × 10−5 M concentration at 25 °C and all the compounds exhibited three absorption bands in the region 200–250 nm, 250–300 nm and 300–400 nm (Fig. 3). The presence of multiple peaks in the absorption spectra can be attributed to the extended conjugated system at the C-5 position of the nucleoside, corresponding to n → π* and π → π* transitions in the UV-vis region. Further, no significant effect on the absorption spectra was observed on changing the substituents at the C-2′′, C-3′′, C-5′′ or C-6′′ positions of the 1′′,4′′-dihydropyridine ring for compounds 6a–6h. However, a significant hypsochromic shift for compound 6i was observed.
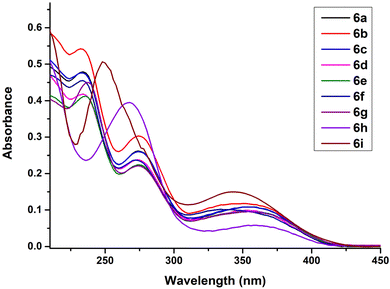 |
| Fig. 3 UV/vis absorption spectra of compounds 6a–i in acetonitrile at 2.5 × 10−5 M concentration. | |
3.2. Emission spectra
The emission spectra of the 5-(1′′,4′′-dihydropyridine)-2′-deoxyuridine nucleoside analogues 6a–i was also recorded in acetonitrile at the same concentration of 2.5 × 10−5 M with λex = 235 nm as the excitation wavelength (Fig. 4). All the compounds exhibited a strong red-shifted band in the region 446–477 nm with two weak bands at around 310 and 350 nm. The highest fluorescence intensity was observed for compounds 6a and 6h. No significant substituent effect was observed on the emission spectra for compounds 6a–6h. However, a significant bathochromic shift was observed for compound 6i with a red-shifted band at 504 nm. Also, a weak blue-shifted band appeared at 310 nm for compound 6i.
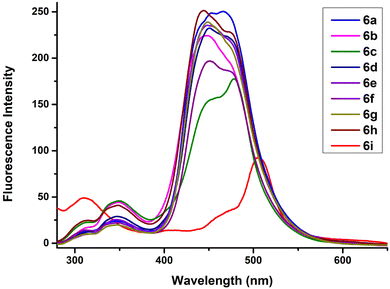 |
| Fig. 4 Emission spectra of compounds 6a–i in acetonitrile at 2.5 × 10−5 M concentration (λex = 235 nm). | |
3.3. Quantum yield calculations and Stokes shift analysis
The quantum yields ΦF for compounds 6a–i were calculated using the following equation:
ΦF = Φst × Su/Sst × Ast/Au × nDu2/nDst2 |
with quinine sulphate (Φst = 0.54 in 0.1 M H2SO4) as the reference standard. Wherein, ΦF = Emission quantum yield for the synthesized compounds 6a–i, Φst = emission quantum yield of the standard, Sst = integrated emission band area of the standard Su = integrated emission band area of the samples Ast = absorbance of the standard at the excitation wavelength Au = absorbance of the samples at the excitation wavelength ηDst = solvent refractive index of the standard ηDu = solvent refractive index of the sample.
The subscripts ‘u’ and ‘st’ are used to denote the unknown samples and the standard, respectively. The quantum yields thus calculated are tabulated in Table 2.
Table 2 Photophysical properties of 5-(1′′,4′′-dihydropyridine)-2′-deoxyuridine nucleoside analogues 6a–i in acetonitrile
Compound no. |
Absorbance λabs (nm) |
Emission λem (nm) |
Stokes shift (nm) |
Quantum yield (ΦF) |
Molar extinction coefficient ε (M−1 cm−1) |
Brightness ε × ΦF (M−1 cm−1) |
6a |
356 |
464 |
108 |
0.077 |
1.9 × 104 |
1.5 × 103 |
6b |
352 |
446 |
94 |
0.080 |
2.1 × 104 |
1.7 × 103 |
6c |
356 |
477 |
121 |
0.119 |
1.9 × 104 |
2.2 × 103 |
6d |
354 |
472 |
118 |
0.091 |
1.7 × 104 |
1.5 × 103 |
6e |
356 |
449 |
93 |
0.089 |
1.6 × 104 |
1.5 × 103 |
6f |
334 |
474 |
140 |
0.078 |
1.8 × 104 |
1.4 × 103 |
6g |
360 |
474 |
114 |
0.123 |
9.4 × 103 |
1.1 × 103 |
6h |
354 |
449 |
95 |
0.074 |
1.8 × 104 |
1.3 × 103 |
6i |
342 |
504 |
162 |
0.022 |
2.0 × 104 |
4.4 × 102 |
The quantum yield values (ΦF) were found to be in the range 0.022–0.123 with the highest being 0.123 for compound 6g. High values of Stokes shift were observed ranging from 93–162 nm. The brightness of all modified nucleosides 6a–i was calculated and enlisted in Table 2.
3.4. Solvatochromism
Now, in order to study the effect of solvent polarity on the photophysical properties of the synthesized nucleoside analogues 6a–i, the solvatochromic characterization of one of the compounds 6g was performed in six different organic solvents; acetonitrile, CHCl3, MeOH, DMF, DMSO and ethyl acetate.
Minor bathochromic/hypsochromic shifts in the absorption spectra were observed on changing the solvent from acetonitrile to CHCl3, MeOH, DMF, DMSO or ethyl acetate (Fig. 5). A dual emission was observed in MeOH (310 nm and 456 nm), DMF (313 nm and 450 nm), DMSO (315 nm and 454 nm) and ethyl acetate (338 nm and 444 nm) while a single emission band was observed in acetonitrile (474 nm) and CHCl3 (442 nm) (Fig. 6). The highest fluorescence intensity as well as highest quantum yield (0.579) was observed in DMSO. The highest Stokes shift value (114 nm) was observed in case of acetonitrile while the lowest value (70 nm) was recorded in CHCl3. The emission wavelength, quantum yield, Stokes shift values as well as the molar absorption coefficient of compound 6g in different solvents is tabulated in Table 3.
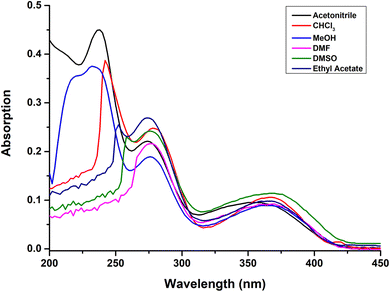 |
| Fig. 5 UV/vis absorption spectra of compound 6g in different organic solvents; acetonitrile, CHCl3, MeOH, DMF, DMSO, and ethyl acetate at 2.5 × 10−5 M concentration. | |
 |
| Fig. 6 Emission spectra of compound 6g in different organic solvents; acetonitrile, CHCl3, MeOH, DMF, DMSO, and ethyl acetate at 2.5 × 10−5 M concentration. | |
Table 3 Photophysical properties of 5-(1′′,4′′-dihydropyridine)-2′-deoxyuridine 6g in acetonitrile, CHCl3, MeOH, DMF, DMSO, and ethyl acetate
Properties |
Solvent |
Acetonitrile |
CHCl3 |
MeOH |
DMF |
DMSO |
Ethyl acetate |
Absorbance λabs (nm) |
360 |
372 |
370 |
368 |
375 |
369 |
Emission λem (nm) |
474 |
442 |
456 |
450 |
454 |
444 |
Stokes shift (nm) |
114 |
70 |
86 |
82 |
79 |
75 |
Quantum yield (ΦF) |
0.123 |
0.331 |
0.441 |
0.468 |
0.579 |
0.197 |
Molar extinction coefficient ε (M−1 cm−1) |
9.4 × 103 |
1.5 × 104 |
1.4 × 104 |
8.8 × 103 |
9.9 × 103 |
1.2 × 104 |
Brightness ε × ΦF (M−1 cm−1) |
1.1 × 103 |
4.9 × 103 |
6.2 × 103 |
4.1 × 103 |
5.7 × 103 |
2.3 × 103 |
3.5. Comparative discussion
The synthesized fluorescent 1,4-dihydropyridine nucleoside analogues 6a–i demonstrated superior photophysical properties compared to previously reported C-5 modified pyrimidine nucleosides. Specifically, these compounds exhibited higher quantum yields (0.022–0.579) and Stokes shift values (70–162 nm), as detailed in Table 4. This indicated that these modified nucleosides not only surpassed the quantum yield values obtained in previous reports but also showed competitive Stokes shift values, emphasizing their potential in fluorescence applications.
Table 4 Comparison of various photophysical parameters of compound 6g with reported C-5 modified pyrimidine nucleosidesa
Ref. |
Structure |
Absorbance λabs (nm) |
Emission λem (nm) |
Stokes shift (nm) |
Quantum yield (ΦF) |
Extinction coefficient ε (M−1 cm−1) |
Solvent |
Nr: not reported. |
Ref. 31d |
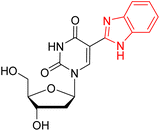 |
320 |
405 |
85 |
0.039 |
Nr |
100 mM HCl |
Ref. 31e & 34 |
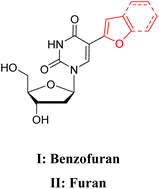 |
(I) 322 |
(I) 423 |
(I) 101 |
(I) 0.12 |
(I) nr |
(I) MeOH |
(II) 316 |
(II) 431 |
(II) 115 |
(II) 0.03 |
(II) nr |
(II) H2O |
Ref. 31a |
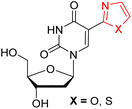 |
(X = O) 296 |
(X = O) 400 |
(X = O) 104 |
(X = O) < 0.01 |
(X = O) nr |
(X = O) H2O |
(X = S) 316 |
(X = S) 404 |
(X = S) 88 |
(X = S) < 0.01 |
(X = S) nr |
(X = S) H2O |
Ref. 31b |
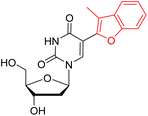 |
306 |
457 |
151 |
0.009 |
Nr |
MeOH |
Ref. 32 |
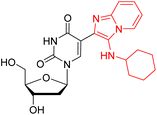 |
242 |
346 |
107 |
0.072 |
1.46 × 104 |
MeOH |
This work (6g) |
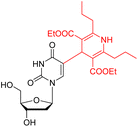 |
370 |
456 |
86 |
0.441 |
1.4 × 104 |
Acetonitrile |
375 |
454 |
79 |
0.579 |
9.9 × 103 |
DMSO |
4. Conclusion
In this study, we have successfully developed an environmentally-friendly, economical and highly efficient methodology for synthesizing a small library of novel fluorescent 1,4-dihydropyridine nucleoside analogues, 5a–i and 6a–i, via a one-pot three-component Hantzsch condensation reaction under solvent-free conditions. This process involved reaction between 3′,5′-di-O-acetyl-5-formyl-2′-deoxyuridine 3, β-keto ester 4a–h or acetyl acetone 4i, and ammonium acetate, catalyzed by Ba(NO3)2 under microwave irradiation. This method offered high efficiency, producing the desired products in high yields up to 96%. Comparative studies demonstrated the superior performance of microwave irradiation over conventional heating. The protocol demonstrated practicability and broad applicability through successful gram-scale synthesis. The synthesized compounds displayed strong fluorescence at around 442–477 nm and exhibited significantly higher absorption and emission bands compared to thymidine, attributed to extended conjugation. Photophysical investigations revealed a noteworthy fluorescence intensity, excellent Stokes shift values (70–162 nm), and superior quantum yields (0.022–0.579), particularly notable in DMSO (0.579). These highly fluorescent nucleoside derivatives are suitable for their incorporation into oligonucleotides for carrying out hybridization studies with complementary DNA strands, facilitating the investigation of DNA local structure.
Data availability
The authors confirm that the data supporting the findings of this study are available within the article and its ESI materials.†
Conflicts of interest
The authors declare no competing financial interest.
Acknowledgements
We appreciate the funding provided by the Institute of Imminence (IOE), University of Delhi which has contributed to further research and development. We appreciate the assistance provided by USIC and Department of Chemistry, University of Delhi for recording NMR, HRMS and IR data. Aditi Arora (SRF, File No. 09/0045(11270)/2021/EMR-I) and Sumit Kumar (SRF, File No. 09/045(1798)/2020-EMR-1) are grateful to CSIR, New Delhi for their fellowship.
References
- L. P. Jordheim, D. Durantel, F. Zoulim and C. Dumontet, Nat. Rev. Drug Discovery, 2013, 12, 447 CrossRef CAS PubMed.
- K. L. Seley-Radtke and M. K. Yates, Antivir. Res., 2018, 154, 66 CrossRef CAS PubMed.
- J. Zhang, F. He, J. Chen, Y. Wang, Y. Yang, D. Hu and B. Song, J. Agric. Food Chem., 2021, 69, 5575 CrossRef CAS PubMed.
- C. K. Chu, L. Ma, S. Olegen, C. Pierra, J. Du, G. Gumina, E. Gullen, Y. C. Cheng and R. F. Schinazi, J. Med. Chem., 2000, 43, 3906 CrossRef CAS PubMed.
- D. C. Schultz, R. M. Johnson, K. Ayyanathan, J. Miller, K. Whig, B. Kamalia, M. Dittmar, S. Weston, H. L. Hammond, C. Dillen, J. Ardanuy, L. Taylor, J. S. Lee, M. Li, E. Lee, C. Shoffler, C. Petucci, S. Constant, M. Ferrer, C. A. Thaiss, M. B. Frieman and S. Cherry, Nature, 2022, 604, 134 CrossRef CAS PubMed.
- P. Sharma, V. Nurpeisov, H. B. Santiago, T. Beltran and R. Schinazi, Curr. Top. Med. Chem., 2004, 4, 895 CrossRef CAS PubMed.
- Y. Yoshida, M. Honma, Y. Kimura and H. Abe, ChemMedChem, 2020, 16, 743 CrossRef PubMed.
- P. L. Bonate, L. Arthaud, W. R. Cantrell, K. Stephenson, J. A. Secrist and S. Weitman, Nat. Rev. Drug Discovery, 2006, 5, 855 CrossRef CAS PubMed.
- M. Guinan, C. Benckendorff, M. Smith and G. J. Miller, Molecules, 2020, 25, 2050 CrossRef CAS PubMed.
- G. Elgemeie, Curr. Pharm. Des., 2003, 9, 2627 CrossRef CAS PubMed.
- W. Parker, J. Secrist and W. Waud, Curr. Opin. Invest. Drugs, 2004, 5, 592 CAS.
- V. K. Sharma, R. K. Sharma and S. K. Singh, MedChemComm, 2014, 5, 1454 RSC.
- Y. Mehellou and E. D. Clercq, J. Med. Chem., 2010, 53, 521 CrossRef CAS PubMed.
- E. D. Clercq, J. Med. Chem., 2010, 53, 1438 CrossRef PubMed.
- W. H. Prusoff, Biochim. Biophys. Acta, 1959, 32, 295 CrossRef CAS PubMed.
-
(a) G. B. Elion, P. A. Furman, J. A. Fyfe, P. Miranda, L. Beauchamp and H. J. Schaeffer, Proc. Natl. Acad. Sci. U.S.A., 1977, 74, 5716 CrossRef CAS PubMed;
(b) K. Katarzyna, P. Aneta, K. Anna and N. Maria, Curr. Med. Chem., 2020, 27, 4118 CrossRef PubMed;
(c) Y. P. Wei, L. Y. Yao, Y. Y. Wu, X. Liu, L. H. Peng, Y. L. Tian, J. H. Ding, K. H. Li and Q. G. He, Molecules, 2021, 26, 6566 CrossRef CAS PubMed.
-
(a) T. Waga, H. Ohrui and H. Meguro, Nucleosides Nucleotides, 1996, 15, 287 CrossRef CAS;
(b) R. Yarchoan, P. Brouwers, A. R. Spitzer, J. Grafman, B. Safai, C. F. Perno, S. M. Larson, G. B. M. Fischl, A. Wichman, R. V. Thomas, A. Brunetti, P. J. Schmidt, C. E. Myers and S. Broder, Lancet, 1987, 329, 132 CrossRef PubMed;
(c) R. Yarchoan, R. V. Thomas, J. P. Allain, N. Mcatee, R. Dubinsky, H. Mitsuya, T. J. Lawley, B. Safai, C. E. Myers, C. F. Perno, R. W. Klecker, R. J. Wills, M. A. Fischl, M. C. Mcneely, J. M. Pluda, M. Leuther, J. M. Collins and S. Broder, Lancet, 1988, 331, 76 CrossRef PubMed;
(d) R. Yarchoan, H. Mitsuya, R. V. Thomas, J. M. Pluda, N. R. Hartman, C. F. Perno, K. S. Marczyk, J. P. Allain, D. G. Johns and S. Broder, Science, 1989, 245, 412 CrossRef CAS PubMed;
(e) R. Yarchoan, H. Mitsuya, C. E. Myers and S. N. Broder, N. Engl. J. Med., 1989, 321, 726 CrossRef CAS PubMed;
(f) M. Miller, J. Schneider, B. K. Sathyanarayana, M. V. Toth, G. R. Marshall, L. Clawson, L. Selk, S. B. H. Kent and A. Wlodawer, Science, 1989, 246, 1149 CrossRef CAS PubMed.
-
(a) K. Z. Rana and M. N. Dudley, Clin. Pharmacokinet., 1997, 33, 276 CrossRef CAS PubMed;
(b) M. Hurst and S. Noble, Drugs, 1999, 58, 919 CrossRef CAS PubMed;
(c) J. A. S. Al-Hussaini, O. A. Hatem and Z. A. H. Alebady, Syst. Rev. Pharm., 2020, 11, 96 CAS;
(d) B. Kumar, A. Sharma, S. K. Tiwari, D. Agrawal, A. K. Sharma and M. Khandelwal, Eur. J. Biomed. Pharm., 2021, 8, 148 CAS.
-
(a) B. Jarvis and D. Faulds, Drugs, 1999, 58, 101 CrossRef CAS PubMed;
(b) R. Quercia, C. F. Perno, J. Koteff, K. Moore, C. McCoig, M. S. Clair and D. Kuritzkes, J. Acquir. Immune Defic. Syndr., 2018, 78, 125 CrossRef CAS PubMed;
(c) R. Patel, L. Evitt, I. Mariolis, S. D. Giambenedetto, A. Monforte, J. Casado, A. C. Úbeda, L. Hocqueloux, C. Allavena, T. Barber, D. Jha, R. Kumar, R. D. Kamath, T. Vincent, J. V. Wyk and J. Koteff, Infect. Dis. Ther., 2021, 10, 2051 CrossRef PubMed;
(d) R. Roediger, E. K. Smyth and D. Dieterich, Antivir. Ther., 2022, 27, 1 Search PubMed.
-
(a) N. Katagiri, M. Takebayashi, H. Kokufuda, C. Kaneko, K. Kanehira and M. Torihara, J. Org. Chem., 1997, 62, 1580 CrossRef CAS;
(b) V. E. Kataev and B. F. Garifullin, Chem. Heterocycl. Compd., 2021, 57, 326 CrossRef CAS PubMed.
- B. Y. Michel, D. Dziuba, R. Benhida, A. P. Demchenko and A. Burger, Front. Chem., 2020, 8, 112 CrossRef CAS PubMed.
- F. Seela and V. R. Sirivolu, Org. Biomol. Chem., 2008, 6, 1674 RSC.
- I. Schönrath, V. B. Tsvetkov, T. S. Zatsepin, A. V. Aralov and J. Müller, J. Biol. Inorg. Chem., 2019, 24, 693–702 CrossRef PubMed.
- A. C. Jones and R. K. Neely, Rev. Geophys., 2015, 48, 244–279 CAS.
- T. Gustavsson, N. Sarkar, Á. Bányász, D. Markovitsi and R. Improta, Photochem. Photobiol., 2007, 83, 595–599 CrossRef CAS PubMed.
- M. Wilhelmsson & Y. Tor, Fluorescent Analogues of Biomolecular Building Blocks: Design and Applications, John Wiley & Sons, 2016 Search PubMed.
- Y. Saito and R. H. E. Hudson, J. Photochem. Photobiol. C Photochem., 2018, 36, 48–73 CrossRef CAS.
- L. Zilbershtein-Shklanovsky, M. Weitman, D. T. Major and B. Fischer, J. Org. Chem., 2013, 78, 11999–12008 CrossRef CAS PubMed.
- K. Seio, T. Kanamori and Y. Masaki, Tetrahedron Lett., 2018, 59, 1977–1985 CrossRef CAS.
- J. Zayas, M. Annoual, J. K. Das, Q. Felty, W. G. Gonzalez, J. Mikssovska, N. Sharifai, A. Chiba and S. F. Wnuk, Bioconjugate Chem., 2015, 26, 1519–1532 CrossRef CAS PubMed.
-
(a) N. J. Greco and Y. Tor, Tetrahedron, 2007, 63, 3515–3527 CrossRef CAS PubMed;
(b) T. Kanamori, H. Ohzeki, Y. Masaki, A. Ohkubo, M. Takahashi, K. Tsuda, T. Ito, M. Shirouzu, K. Kuwasako, Y. Muto, M. Sekine and K. Seio, ChemBioChem, 2015, 16, 167–176 CrossRef CAS PubMed;
(c) M. S. Noé, R. W. Sinkeldam and Y. Tor, J. Org. Chem., 2013, 78, 8123–8128 CrossRef PubMed;
(d) P. Guo, X. Xu, X. Qiu, Y. Zhou, S. Yan, C. Wang, C. Lu, W. Ma, X. Weng, X. Zhang and X. Zhou, Org. Biomol. Chem., 2013, 11, 1610–1613 RSC;
(e) A. A. Tanpure and S. G. Srivatsan, ChemBioChem, 2012, 13, 2392–2399 CrossRef CAS PubMed.
- S. Kumar, S. Kumar, J. Maity, B. Kumar, S. B. Mehta and A. K. Prasad, New J. Chem., 2021, 45, 16635–16647 RSC.
- N. Amann and H. A. Wagenknecht, Synlett, 2002, 5, 0687–0691 CrossRef.
- N. J. Greco and Y. Tor, J. Am. Chem. Soc., 2005, 127, 10784–10785 CrossRef CAS PubMed.
- C. Isabelle, B. Norberg, A. Olivier, C. Evrard, G. Evrard, P. Wigerninck, P. Herdewijn and F. Durant, J. Chem. Crystallogr., 1996, 26, 777–789 CrossRef.
- A. V. Ardhapure, V. Gayakhe, S. Bhilare, A. R. Kapdi, S. S. Bag, Y. S. Sanghvi and K. C. Gunturu, New J. Chem., 2020, 44, 14744–14754 RSC.
- A. J. Gutierrez, T. J. Terhorst, M. D. Matteucci and B. C. Froehler, J. Am. Chem. Soc., 1994, 116, 5540–5544 CrossRef CAS.
- Z. Wen, P. R. Tuttle, A. H. Howlader, A. Vasilyeva, L. Gonzalez, A. Tangar, R. Lei, E. E. Laverde, Y. Liu, J. Miksovska and S. F. Wnuk, J. Org. Chem., 2019, 84, 3624–3631 CrossRef CAS PubMed.
- H. Song, X. Li, Y. Long, G. Schatte and H. B. Kraatz, Dalton Trans., 2006, 4696–4701 RSC.
- X. Fan, X. Zhang, L. Zhou, K. A. Keith, E. R. Kern and P. F. Torrence, Bioorg. Med. Chem. Lett., 2006, 16, 3224–3228 CrossRef CAS PubMed.
- T. Ehrenschwender and H. A. Wagenknecht, Synthesis, 2008, 22, 3657–3662 Search PubMed.
- X. Fan, Y. Wang, Y. He, X. Zhang and J. Wang, Tetrahedron Lett., 2010, 51, 3493–3496 CrossRef CAS.
- X. Zhang, X. Li, X. Fan, X. Wang, D. Li, G. Qu and J. Wang, Mol. Divers., 2009, 13, 57–61 CrossRef CAS PubMed.
- A. P. Silverman and E. T. Kool, Chem. Rev., 2006, 106, 3775 CrossRef CAS PubMed.
- A. V. Ivanov, A. R. Simonyan, E. F. Belanov and L. A. Aleksandrova, Russ. J. Bioorg. Chem., 2005, 31, 556–562 CrossRef CAS PubMed.
- M. L. Bryant, E. G. Bridges, L. Placidi, A. Faraj, A. G. Loi, C. Pierra, D. Dukhan, G. Gosselin, J. L. Imbach, B. Hernandez, A. Juodawlkis, B. Tennant, B. Korba, P. Cote, E. Cretton-Scott, R. F. Schinazi and J. P. Sommadossi, Nucleos Nucleot. Nucleic Acids, 2001, 20, 597 CrossRef CAS PubMed.
- X. Fan, X. Zhang, C. Bories, P. M. Loiseau and P. F. Torrence, Bioorg. Chem., 2007, 35, 121–136 CrossRef CAS PubMed.
- P. Wigerinck, R. Snoeck, P. Claes, E. De Clercq and P. Herdewijn, J. Med. Chem., 1991, 34, 1767–1772 CrossRef CAS PubMed.
- J. Krim, C. Grunewald, M. Taourirte and J. W. Engels, Bioorg. Med. Chem., 2012, 20, 480–486 CrossRef CAS PubMed.
- S. Kumar, S. Kumar, J. Maity, A. Arora and A. K. Prasad, ChemistrySelect, 2022, 7(45), e202203432 CrossRef CAS.
- A. K. Prasad, M. D. Sorensen, V. S. Parmar and J. Wengel, Tetrahedron Lett., 1995, 36, 6163–6166 CrossRef CAS.
- C. Beuck, I. Singh, A. Bhattacharya, W. Hecker, V. S. Parmar, O. Seitz and E. Weinhold, Angew. Chem., Int. Ed., 2003, 42, 3958–3960 CrossRef CAS PubMed.
- Poonam, A. K. Prasad, C. Mukherjee, G. Shakya, G. K. Meghwanshi, J. Wengel, R. K. Saxena and V. S. Parmar, Pure Appl. Chem., 2005, 77, 237–243 CrossRef CAS.
- A. K. Prasad, N. Kalra, Y. Yadav, R. Kumar, S. K. Sharma, S. Patkar, L. Lange, J. Wengel and V. S. Parmar, Chem. Commun., 2007, 2616–2617 RSC.
- J. Maity, G. Shakya, S. Singh, V. T. Ravikumar, V. S. Parmar and A. K. Prasad, J. Org. Chem., 2008, 73, 5629–5632 CrossRef CAS PubMed.
- S. Srivastava, V. K. Maikhuri, R. Kumar, K. Bohra, H. Singla, J. Maity and A. K. Prasad, Carbohydr. Res., 2018, 470, 19–25 CrossRef CAS PubMed.
- S. Kumar, A. Arora, R. Kumar, N. N. Senapati and B. K. Singh, Carbohydr. Res., 2023, 108857 CrossRef CAS PubMed.
- H. Singla, S. Kumar, V. K. Maikhuri, Kavita and A. K. Prasad, ChemistrySelect, 2023, 8, e202203412 CrossRef CAS.
- A. Arora, S. Kumar, S. Kumar, A. Dua and B. K. Singh, Org. Biomol. Chem., 2024, 22, 4922–4939 RSC.
- S. Kumar, A. Arora, S. Kumar, J. Maity, A. Dua and B. K. Singh, J. Mol. Struct., 2024, 1321, 139915 CrossRef.
- A. Hantzsch and J. Liebigs, Ann. Chem., 1882, 215, 1–82 CrossRef.
-
(a) N. Edraki, A. R. Mehdipour, M. Khoshneviszadeh and R. Miri, Drug Discovery Today, 2009, 14, 1058–1066 CrossRef CAS PubMed;
(b) H. S. Sohal, Mater. Today: Proc., 2022, 48, 1163–1170 CAS;
(c) M. G. Sharma, J. Pandya, D. M. Patel, R. M. Vala, V. Ramkumar, R. Subramanian, V. K. Gupta, R. L. Gardas, A. Dhanasekaran and H. M. Patel, Polycyclic Aromat. Compd., 2021, 41, 1495–1505 CrossRef CAS;
(d) S. G. Ouellet, A. M. Walji and D. W. Macmillan, Acc. Chem. Res., 2007, 40, 1327–1339 CrossRef CAS PubMed;
(e) V. Dean, C. M. Wandikayi, R. Manian, I. Nadeem, W. W. Michael, E. H. Susan and E. K. Edward, J. Med. Chem., 1995, 38, 2851–2859 CrossRef PubMed;
(f) F. G. Mikhail, V. P. Dinesh and M. G. Eric, J. Org. Chem., 1996, 61, 924–928 CrossRef;
(g) A. Tambe, G. Sadaphal, R. Dhawale and G. Shirole, Res. Chem. Intermed., 2022, 48, 1273–1286 CrossRef CAS;
(h) D. S. Malhi, M. Kaur and H. S. Sohal, ChemistrySelect, 2019, 4, 11321–11336 CrossRef CAS;
(i) A. S. Khedkar and B. P. Auti, Mini Rev. Med. Chem., 2014, 14, 282–290 CrossRef PubMed;
(j) A. M. Vijesha, A. M. Isloor, S. K. Peethambar, K. N. Shivananda, T. Arulmoli and N. A. Isloor, Eur. J. Med. Chem., 2011, 46, 5549 CrossRef PubMed;
(k) K. Sirisha, D. Bikshapathi, G. Achaiah and V. M. Reddy, Eur. J. Med. Chem., 2011, 46, 1564 CrossRef CAS PubMed;
(l) A. González, J. Casado, M. G. Gündüz, B. Santos, A. Velázquez-Campoy, C. Sarasa-Buisan, M. F. Fillat, M. Montes, E. Piazuelo and A. Lanas, Front. Microbiol., 2022, 25(13), 874709 CrossRef PubMed;
(m) T. Narsinghani, L. K. Soni and S. Chourey, J. Drug Deliv. Therapeut., 2017, 7, 142–145 CAS;
(n) A. Malani, A. Makwana, J. Monapara, I. Ahmad, H. Patel and N. Desai, J. Biochem. Mol. Toxicol., 2021, 35, e22903 CrossRef CAS PubMed;
(o) D. S. Malhi, H. S. Sohal, K. Singh, Z. M. Almarhoon, A. B. Bacha and M. I. Al-Zaben, ACS Omega, 2022, 7, 16055–16062 CrossRef CAS PubMed;
(p) A. K. Chillar, P. Arya, C. Mukherjee, P. Kumar, Y. Yadav, A. K. Sharma, V. Yadav, J. Gupta, R. Dabur, H. N. Jha, A. C. Watterson, V. S. Parmar, A. K. Prasad and G. L. Sharma, Bioorg. Med. Chem., 2006, 14, 973–981 CrossRef PubMed.
- Y. He, H. Wang, C. Ge and H. Yan, J. Mol. Struct., 2023, 1281, 135167 CrossRef CAS.
- Q. Fan, P. Li, H. Yan and J. Photocgem, J. Photochem. Photobiol., A, 2018, 358, 51–60 CrossRef CAS.
- J. Ramchander, G. Raju, N. Rameshwar, T. S. Reddy and A. R. Reddy, Spectrochim. Acta, Part A, 2012, 85, 210–216 CrossRef CAS PubMed.
- L. Camargo da Luz, M. G. Gündz, R. Beal, G. M. Zanotto, E. R. Kuhn, P. A. Netz, C. Şafak, P. F. B. Gonçalves, F. da Silveira Santos, F. S. Rodembusch and J. Photocgem, J. Photochem. Photobiol., A, 2018, 358, 51–60 CrossRef.
-
(a) A. S. Grewal, K. Kumar, S. Redhu and S. Bhardwaj, Int. Res. J. Pharmaceut. Appl. Sci., 2013, 3, 278–285 Search PubMed;
(b) A. R. Yadav, S. K. Mohie and C. S. Magdum, Asian J. Res. Chem., 2020, 13, 275–278 CrossRef.
- A. Amer, S. Senior and X. Fan, Nucleosides, Nucleotides Nucleic Acids, 2012, 31, 42–54 CrossRef CAS PubMed.
|
This journal is © The Royal Society of Chemistry 2024 |
Click here to see how this site uses Cookies. View our privacy policy here.