DOI:
10.1039/D3SC05700C
(Edge Article)
Chem. Sci., 2024,
15, 3182-3191
Strain-enabled radical spirocyclization cascades: rapid access to spirocyclobutyl lactones and – lactams†
Received
25th October 2023
, Accepted 20th December 2023
First published on 21st December 2023
Abstract
Spirocyclobutane derivatives have gained significant attention in drug discovery programs due to their broad spectrum of biological activities and clinical applications. Ring-strain in organic molecules is a powerful tool to promote reactivity by releasing strain energy, allowing the construction of complex molecules selectively and efficiently. Herein, we report the first strain-enabled radical spirocyclization cascades for the synthesis of functionalized spirocyclobutyl lactones and – lactams, which are finding increasing applications in medicinal chemistry. The reaction of interelement compounds with bicyclobutane (BCB) allyl esters and – amides proceeds with high chemoselectivity under simple, catalyst-free conditions using blue light irradiation. The reaction has been successfully extended to synthesize bis-spirocycles. To introduce a more diverse set of functional groups, we have developed a dual photoredox/nickel catalytic system capable of mediating the carbosulfonylation of BCB allyl amides. The reaction shows broad applicability across various (hetero)aryl halides, aryl sulfinates, and BCB allyl amides, operates under mild conditions and demonstrates excellent functional group compatibility. The functional groups introduced during the cascade reactions served as versatile handles for further synthetic elaboration.
Introduction
Spirocyclic compounds are among the most important structural components of small-molecule drug candidates.1 Due to their rigid conformation, substituents on spirocyclic scaffolds are well-defined in their spatial arrangement and, thus, allow for the orientation of various functional groups along the designated vectors. As a result, interactions of a ligand with a three-dimensional (3D) binding site can be attained more effectively with a spirocyclic motif than with flat aromatic compounds.2 Owing to its unique puckered structure, the inclusion of a cyclobutane ring also allows for the orientation of substituents along pre-selected vectors, and it improves certain physicochemical properties and pharmacokinetics, such as metabolic stability, lipophilicity, and acidity/basicity (Scheme 1A).3 All these factors have fueled a rush in reports of biologically active spirocyclobutane-based compounds.1c,4 Among these, spirocyclobutyl lactones and – lactams have gained significant attention in medicinal chemistry discovery programs due to their broad biological activity spectrum (Scheme 1A).5 Despite this, there is scant literature on the synthesis of these privileged motifs.6 Another limitation is the difficulty in accessing functionalized spirocyclobutyl lactones and – lactams that would allow extra structural variations. Such motifs would represent very useful building blocks that could foster the application of these spirocycles in drug discovery.5
 |
| Scheme 1 (A) Importance of spirocyclobutanes. (B) Previous reports on BCBs. (C) Our reaction design and challenges. (D) This work: strain-enabled radical spirocyclization cascades. | |
Strain energy in organic molecules is a powerful driving force that promotes reactivity through the release of ring-strain, allowing a plethora of valuable transformations with applications in various research fields, including total synthesis, bioconjugation, bioisosterism, and polymer science.7 Bicyclobutane (BCB), the smallest fused strained hydrocarbon with a ring-strain of 64 kcal mol−1, gained significant attention in recent years not only due to its unique structural features but also its ability to unlock strategies through strain release to synthetically challenging compounds either via single-electron or two-electron pathways.8 For example, the stained carbon–carbon bond of BCB derivatives participates in various difunctionalization,6c,9 carbene insertion,10 or cycloaddition reactions,11 leading to highly valuable carbocycles and heterocycles, which are bioisosteres of (hetero)arenes (Scheme 1B).12 Despite the growing number of synthetic strategies using BCBs, reports on strain-enabled radical spirocyclization cascades haven't been studied to date. However, such radical strategies would give rapid access to functionalized spirocyclobutane derivatives, which are finding increasing applications in pharmaceuticals.5
Fundamentally new triggers for spirocyclization, especially for the construction of all-carbon quaternary spirocentres, are highly desirable since they have the potential to open considerable chemical space and can lead to new avenues in chemical synthesis. We envisioned the possibility of a novel strain-enabled radical spirocyclization cascade. This approach involves a series of well-orchestrated, radical-mediated bond-forming events (Scheme 1C). We hypothesized that radical intermediate II could be accessed by the addition of radical I, which could be generated either by photolysis or single electron oxidation of the corresponding radical precursor, onto the strained carbon–carbon bond of BCB A. Subsequent 5-exo-trig cyclization would lead to alkyl radical III that could be trapped with another radical intermediate IV to give spirocyclobutane B. Alternatively, the radical III could also be intercepted with a metal species under metallophotoredox catalysis for further functionalization to afford spirocyclobutane C. We recognized three main challenges associated with the successful realization of this radical cascade: (1) the radical I should first add chemoselectively to the strained carbon–carbon bond rather than adding to the π bond of the olefin, (2) the rate of cyclization of the radical intermediate II should be faster than the direct functionalization to avoid the direct addition product D, and (3) the further functionalization of alkyl radical III should be quicker to prevent the formation of hydrogen atom transfer (HAT) product E (Scheme 1C). Notwithstanding these challenges, here we describe the successful realization of this strategy and report the first strain-enabled radical spirocyclization cascades, providing rapid access to functionalized spirocyclobutyl lactones and – lactams for augmenting medicinal chemistry libraries (Scheme 1D). Notably, the methodology was employed to access bis-spirocycles, which constitute the core frameworks of bioactive compounds.13 To broaden the scope, we have developed a dual photoredox/nickel catalytic system capable of mediating the carbosulfonylation of BCB allyl amides. The mild conditions display excellent functional group tolerance and broad substrate scope. Furthermore, the functional groups introduced during the reaction served as versatile handles for further synthetic modifications.
Results and discussion
Initial exploration into the proposed spirocyclization cascade process focused on using BCB tethered allyl ester 1a and a bifunctional oxime ester 4, which was previously used by Glorius and co-workers for difunctionalization of alkenes under energy transfer photocatalysis (Scheme 2).14 When we irradiated a mixture of 1a and 4 using thioxanthone as a photocatalyst in EtOAc, the formation of desired spirocycle 5 was not observed and the starting material BCB was simply reisolated. Next, we used molecular iodine under light irradiation to promote the cascade.15 Although we didn't detect the desired spirocycle, the direct di-iodination product 6 was formed in 43% yield.16 Our attempts to cyclize 6 under photochemical conditions were unsuccessful. To force the cyclization, we sought to use the Thorpe–Ingold effect17 by employing the gem-dimethyl substituted BCB allyl ester 1b. However, treatment of 1b with iodine also delivered the di-iodination product 7 instead of the spirocycle product. Next, we turned our attention to employing diphenyl disulfide 2a, which is known to add to unsaturated compounds and strained carbon–carbon bonds and can also capture carbon radicals.18
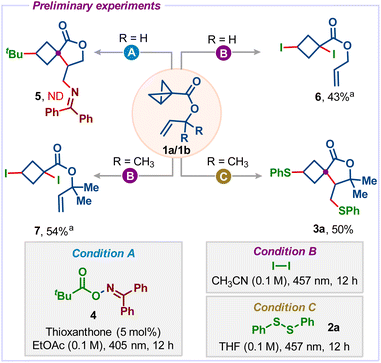 |
| Scheme 2 Preliminary experiments. a Direct iodine addition products were also obtained without light irradiation. We used light irradiation to cyclize it, but unfortunately, it didn't work. | |
To our delight, the treatment of 1b with diphenyl disulfide 2a under blue light irradiation delivered the desired spirocycle 3a in 50% yield along with the direct addition product 8 and HAT product 9. These initial results encouraged us to optimize this reaction further. Among the solvents tested (Fig. 1, columns 1–6): CH3CN was the optimal solvent, suppressing the formation of undesired products 8 and 9 and furnishing the desired product in 77% yield. When the reaction was performed using the Ir-photocatalyst, which was employed for transferring the energy to dialkyl disulfide,18a no reaction was observed (Fig. 1, column 7). A control experiment demonstrated the importance of light, as 3a was not observed in the dark, even at 60 °C (Fig. 1, column 8).
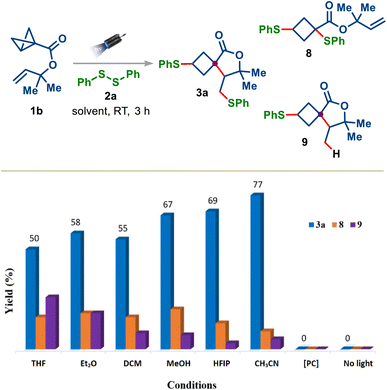 |
| Fig. 1 Optimization of the reaction conditions. Reactions were carried out under an inert atmosphere. Conditions: 1b (0.15 mmol), 2a (0.15 mmol), solvent (1.5 mL, 0.1 M), 457 nm photocube, 3 h. [PC] = [Ir(dF(CF3)ppy)2(dtbbpy)]PF6. Yields were determined by 1H-NMR using CH2Br2 as the internal standard. Depending on the solvent, dr varied between 1.7 : 1 to 1.2 : 1 (see the ESI†). | |
Having established the optimal conditions, the scope of BCB allyl esters was evaluated using 1,2-bis(4-methoxyphenyl)disulfane (2b) (Scheme 3). Simple gem-dimethyl substituted BCB allyl ester 1b provided the spirocycle 3b in 75% yield. Next, we applied our methodology for the synthesis of bis-spirocyclic scaffolds, which are core frameworks in several biologically active natural products and show attractive features, including high three-dimensional character, molecular complexity, and rich Fsp3.13 To our delight, the reaction worked efficiently with cyclobutyl, cyclopentyl, cyclohexyl, cycloheptyl, and piperidinyl appended BCB allyl esters, providing the corresponding bis-spirocycles 3c–3g in moderate to good yields. Subsequently, we explored the scope of the diaryl disulfides using BCB allyl ester 1e. Simple diphenyl disulfide and electron-donating substituents bearing diaryl disulfides underwent the desired transformation successfully to afford bis-spirocycles 3h–3k. Notably, 1,2-bis(4-bromophenyl)disulfane also participated well in this reaction, furnishing the desired spirocycle 3j, leaving the C–Br bond intact, which is useful for further synthetic diversification. The reaction was not limited to BCB allyl esters; a range of BCB allyl amides having different substituents on nitrogen could be used successfully, providing access to spirocyclobutyl lactams in good yields (products 3l–3p).
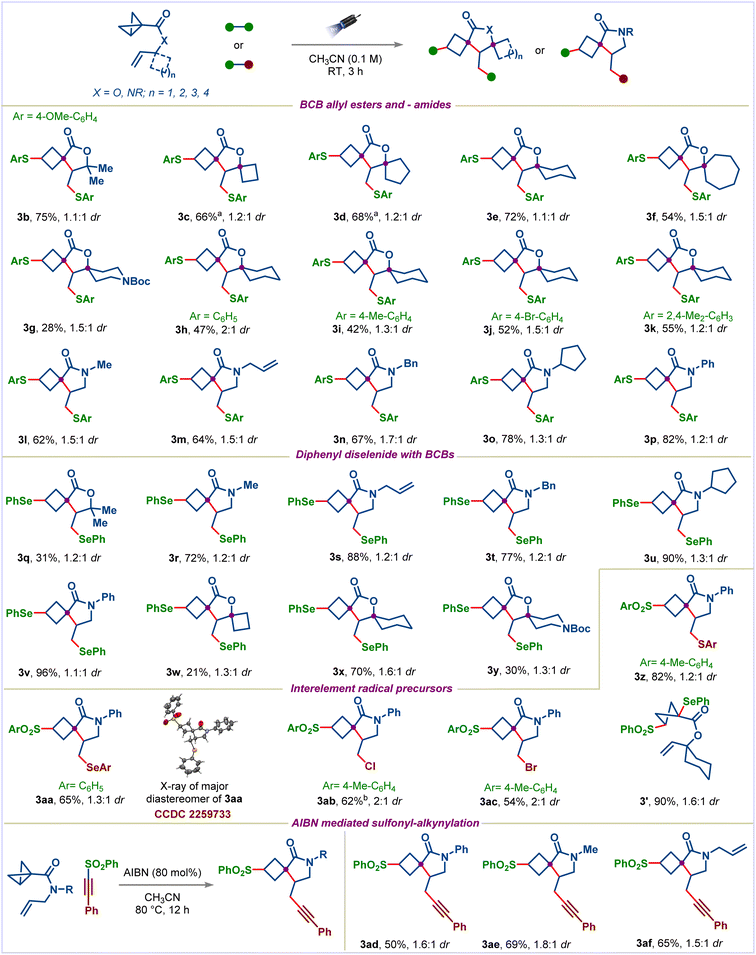 |
| Scheme 3 Scope of BCB allyl esters and – amides with interelement compounds. Reaction conditions: 0.3 mmol of 1, 0.3 mmol of interelement compound 2, 3.0 mL of dry CH3CN, 457 nm photocube, RT, 3 h. Yields are of isolated products and dr was determined by 1H-NMR from the crude reaction mixture. a 0.2 mmol scale. b Using 365 nm. | |
Organoselenium compounds are not only present in several bioactive compounds but are also highly valuable synthetic intermediates as they can be efficiently converted into a broad range of useful functional groups.19 Therefore, next, we turned our attention to accessing selenium-substituted spirocycles. Pleasingly, diphenyl diselenide reacted well under our standard conditions with both BCB allyl esters and – amides, giving the desired spirocycles in moderate to high yields (products 3q–3v). Notably, selenium appended bis-spirocycles 3w–3y could also be accessed.
Next, we sought to explore various interelement radical precursors to selectively introduce different functional groups, which can be further functionalized orthogonally. We were pleased to find that the reaction of BCB allyl amide 1l with S-(4-methylphenyl)4-methylbenzenethiosulfonate afforded the spirocycle 3z in 82% yield with excellent chemoselectivity. Selenosulfonylation was also possible using Se-phenyl benzenesulfonoselenoate with high selectivity. The structure of the major diastereomer of 3aa was unambiguously determined by X-ray analysis (CCDC 2259733). Interelement compounds that simultaneously create carbon-sulfur and carbon–halogen atom bonds could also be utilized in the reaction (products 3ab and 3ac).9r Surprisingly, the reaction of BCB allyl esters with Se-phenyl benzenesulfonoselenoate didn't afford the desired cyclized product; instead, it gave direct addition product 3′ in 90% yield. The reaction with gem-dimethyl substituted BCB allyl ester 1b was also unsuccessful.
Alkynes are versatile functional groups in organic synthesis. Introducing an alkyne group to spirocycles could enhance the transformability of these products. To our delight, treatment of 1l with ((phenylethynyl)sulfonyl)benzene in the presence of AIBN under heating conditions provided the corresponding alkyne bearing spirocycle 3ad in 50% yield.20 Various substituents on the nitrogen of BCB allyl amides were also well tolerated in this reaction (products 3ae and 3af).
Recently, dual photoredox/nickel catalysis has emerged as a powerful synthetic tool in organic chemistry for cross-coupling reactions of alkyl radicals with various electrophiles.21 In most of these cross-coupling reactions, alkyl radicals can either be generated directly by homolytic cleavage of the C(sp3)–X bond of a radical precursor or the generated radicals further undergo a cyclization, β-scission, migratory insertion, or 1,5-HAT process to give new radicals that take part in the following nickel catalyzed cross-coupling reaction. However, to the best of our knowledge, the dual photoredox/nickel strategy has never been applied to cross-coupling alkyl radicals resulting from the strain-enabled radical cascade. Realizing this would significantly extend our strategy to a three-component conjunctive cross-coupling reaction, thus allowing modular construction of diverse and complex spirocyclic scaffolds using readily available aryl sulfinates and (hetero)aryl or alkenyl halides.
Our studies began with the reaction of BCB allyl amide 1l, sodium benzenesulfinate (10a), and 4-bromobenzonitrile (11a) (Fig. 2). Using 2.5 mol% of the organic photocatalyst (4CzIPN), 10 mol% NiCl2·glyme and 15 mol% 4,4′-di-tert-butyl-2,2′-dipyridyl (L1) in CH3CN under blue light irradiation, the desired conjunctive cross-coupled product 12a was obtained in 10% yield (Fig. 2, column 1). Changing to Lewis basic solvents, such as DMSO and DMA, the yield of the product 12a was improved significantly (Fig. 2, columns 2 and 3). Other solvents were also examined but did not give any further improvement (see the ESI† for complete optimization). The yield could be improved to 71% by decreasing the reaction concentration (Fig. 2, column 4). Various ligands on the nickel catalyst had a substantial effect on the reaction yield: 1,10-phenanthroline (L2) and 2,2′:6′,2′′-terpyridine (L3) gave the desired product in much lower yields (Fig. 1, columns 5 and 6), whereas 2,2′-bipyridine (L4) gave similar yield to dtbpy (Fig. 2, column 7). Among the photocatalysts tested, 4CzIPN emerged as the best catalyst (Fig. 2, columns 8–10). Finally, control experiments highlighted the necessity for the nickel catalyst, ligand, photocatalyst, and light (Fig. 2, columns 11–14).
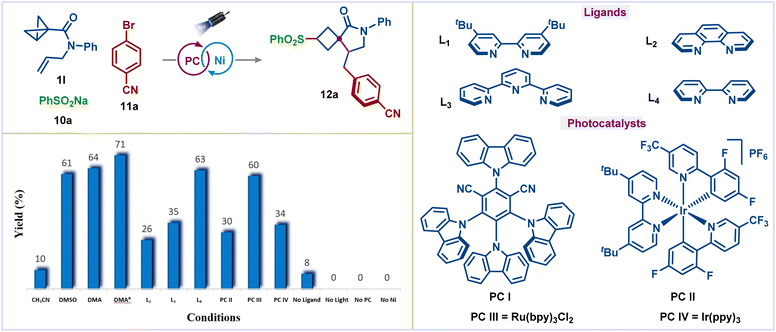 |
| Fig. 2 Optimization of dual photoredox/nickel catalysis. Reactions were carried out under an inert atmosphere. Reaction conditions: 1l (0.15 mmol, 1.0 equiv.), sodium benzenesulfinate (10a) (0.225 mmol, 1.5 equiv.), 4-bromobenzonitrile (11a) (0.30 mmol, 2.0 equiv.) and 4CzIPN (2.5 mol%), NiCl2·glyme (10 mol%), L (15 mol%), 1.5 mL of solvent, 457 nm photocube, RT, 12 h. a 3.0 mL of DMA. Yields were determined by 1H-NMR using CH2Br2 as the internal standard. In all cases, dr is ∼2 : 1, which was determined by 1H-NMR from the crude reaction mixture. | |
With optimum conditions in hand, we first investigated the scope of the reaction with respect to BCB allyl amides (Scheme 4). Various substituents on the nitrogen, including simple phenyl, allyl, benzyl, and cyclopentyl groups were well tolerated (products 12a–12d). Next, we explored a diverse range of aryl halides. Simple iodobenzene, electron-deficient, and electron-rich aryl halides could be successfully coupled to furnish the desired spirocycles 12e–12j in moderate to good yields. The structure of the major diastereomer of 12i was determined by X-ray analysis (CCDC 2292233). Coupling with 1-bromonapthalene was also possible, providing 12k in 65% yield. Noteworthily, the use of heteroaryl halides allowed the introduction of heterocycles, such as quinoline and indole (products 12l and 12m). Next, we showed that alkenyl halides were also viable coupling partners in this reaction, providing the desired alkenylated products 12n and 12o in 37% and 67% yields, respectively. Finally, we explored the scope of aryl sulfinates: electron-donating and -withdrawing groups were well tolerated on the aryl ring (products 12p and 12q). Unfortunately, BCB allyl esters didn't give the desired products under photoredox/nickel dual catalysis.
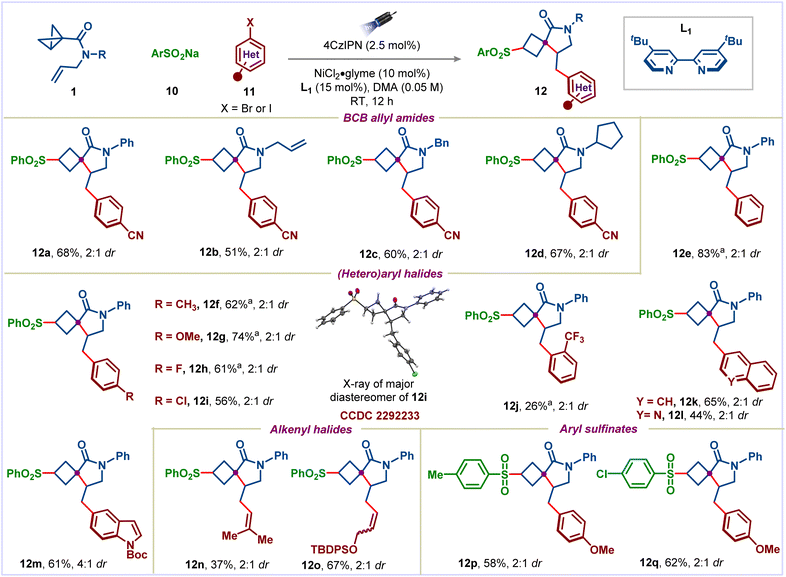 |
| Scheme 4 Scope of dual photoredox/nickel catalysis. Reaction conditions: BCB 1 (0.30 mmol, 1.0 equiv.), sodium arylsulfinate 10 (0.45 mmol, 1.5 equiv.), (hetero)aryl or alkenyl halide 11 (0.60 mmol, 2.0 equiv.) and 4CzIPN (2.5 mol%), NiCl2·glyme (10 mol%), L1 (15 mol%), 6.0 mL of dry DMA, 457 nm photocube, RT, 12 h. Yields are of isolated products and dr was determined by 1H-NMR from the crude reaction mixture. Unless otherwise mentioned, aryl-bromide was used. a Corresponding aryl-iodide was used. | |
To highlight the potential application of our method for the preparation of diverse spirocycles, several transformations of the obtained products were performed. We first synthesized the two spirocycles 3aa and 3ac on a 2 mmol scale with 70% and 50% yields, respectively (Scheme 5A). The phenylselenyl group was selectively reduced under radical conditions, giving spirocycle 13 in 79% yield (Scheme 5B). Oxidation followed by the Seleno-Cope reaction gave exocyclic double bond bearing spirocycle 14 in 41% yield. Treatment of 3aa with borane gave spiro-pyrrolidine derivative 15, a common motif present in several bioactive compounds.22 Finally, we could introduce functional groups, which are highly challenging to install directly during the cascade. For example, we could displace bromide in 3ac successfully with azide under heating conditions in DMF to access azide appended spirocycle 16 in 50% yield.
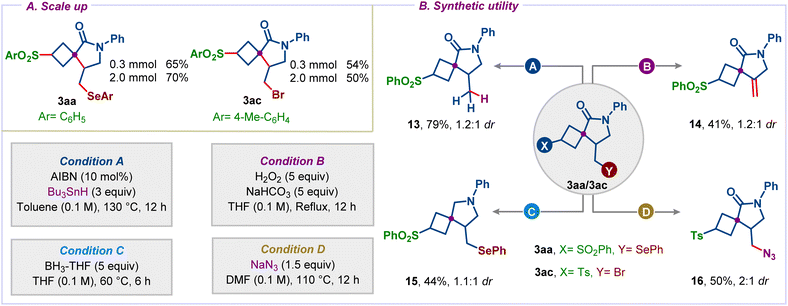 |
| Scheme 5 (A) Scale up. (B) Synthetic utility. | |
Next, we shed light on the mechanism of the reaction involving interelement compounds. To identify key reactive intermediates in this reaction, radical trapping experiments were performed (Scheme 6A). Adding 3 equiv. of TEMPO to the standard conditions led to the complete inhibition of product 3a, with the detection of TEMPO adducts 17, 18 and/or 19. The identified compounds suggest that the reaction proceeds via a radical pathway. Based on radical trapping and literature reports,18b,c we proposed a plausible mechanism for this reaction. The homolytic cleavage of PhSSPh (2a) under visible light irradiation23 leads to the thiyl radical I, which adds to the strained carbon–carbon bond of the BCB 1b to give tertiary radical intermediate II. Subsequent 5-exo-trig cyclization of II generates primary alkyl radical III. Finally, intermediate III reacts with PhSSPh, affords the desired product, and regenerates the thiyl radical I. Alternatively, intermediate III combines with the thiyl radical I to give the desired product 3a. The light on/off experiments suggest that light irradiation is needed throughout the reaction (see the ESI†). However, we cannot rule out the chain propagation mechanism at this stage. The detection of HAT product 9 indicates that the thiyl radical I first added to the strained carbon–carbon bond.
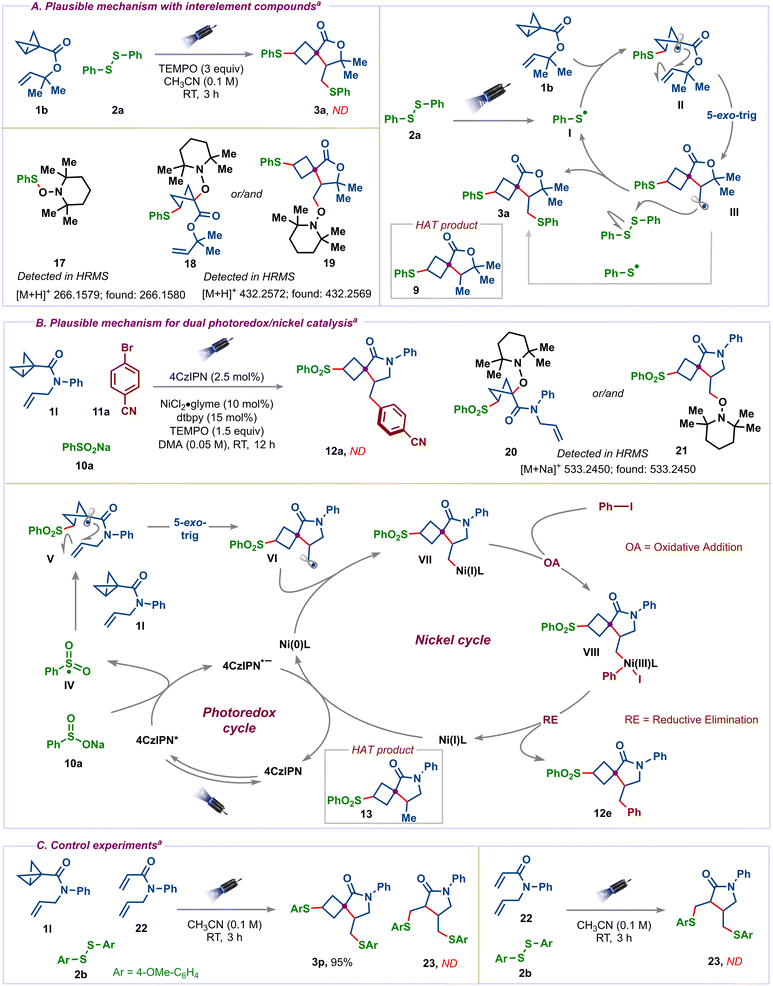 |
| Scheme 6 (A) Plausible mechanism with interelement compounds. (B) Plausible mechanism for dual photoredox/nickel catalysis. (C) Control experiments. a Reactions were carried out on a 0.15 mmol scale. Light source: 457 nm photocube. | |
For the dual photoredox/nickel catalyzed conjunctive cross-coupling reaction, the mechanism starts with the reductive quenching of an excited state of the photocatalyst (PC = 4CzIPN) by single-electron-transfer (SET) from sodium-benzenesulfinate 10 to generate the sulfonyl radical IV (E1/2(PhO2S˙/PhSO2Na) = −0.37 V vs. SCE)24 and (E1/2(PC*/PC˙−) = +1.43 V vs. SCE)25 (Scheme 6B). The addition of the sulfonyl radical to the strained carbon–carbon bond of the BCB 1l then produces a tertiary radical V. Subsequent 5-exo-trig cyclization of the radical onto the olefin gives alkyl radical intermediate VI, which is quickly captured by the Ni(0) complex to furnish Ni(I) species VII. Oxidative addition of iodobenzene to the Ni(I) complex gives Ni(III) complex VIII that subsequently undergoes reductive elimination to give the desired product 12e and Ni(I) species. Next, the reduction of Ni(I) by the reduced photocatalyst (E1/2(PC/PC˙−) = −1.24 V vs. SCE)25a regenerates the photocatalyst and Ni(0) species (Ni(I)/Ni(0) = −1.17 V vs. SCE)26 to close both the catalytic cycles. The detection of TEMPO adducts 20 and/or 21 and HAT product 13 confirms the radical intermediacy in dual catalysis. Finally, successful control experiments (Scheme 6C) using N-allyl-N-phenylacrylamide27 (22) under standard conditions with and without BCB 1l showed that the lack of reactivity with 22 is due to the absence of strained bicyclobutane, which highlights the importance of ring-strain in enabling the present spirocyclization cascades.
Conclusion
In summary, we have developed the first strain-enabled radical spirocyclization cascade for the synthesis of functionalized spirocyclobutyl lactones and – lactams. The applicability of this strategy was further highlighted through the synthesis of a diverse family of bis-spirocyclic scaffolds. The reaction displays broad scope toward BCB allyl esters and – amides and diaryl disulfides with a wide range of functional group tolerance. The reaction was also successfully extended to the synthesis of selenium-substituted spirocycles. Furthermore, various interelement compounds reacted well in this reaction, giving spirocycles with multi-functional groups. Finally, dual photoredox/nickel catalyzed conjunctive cross-coupling of BCB allyl amides with aryl sulfinates and (hetero)aryl halides has been developed for the synthesis of arylated spirocyclobutyl lactams. The multiple points for diversifying the obtained spirocycles provided the potential to construct medicinally relevant spirocycles rapidly. Further extending this methodology to other radical precursors and their applications is currently under investigation in our laboratory.
Data availability
General information, experimental procedures, characterization data for all new compounds, and NMR spectra are in the ESI.† Data for the crystal structure reported in this paper have been deposited at the Cambridge Crystallographic Data Centre (CCDC) under the deposition number CCDC 2259733 and 2292233.
Author contributions
K. D. and D. P. H. conceived and designed the project. K. D. carried out optimization studies, substrate scope, and mechanistic studies. A. P. and T. S. helped in the substrate scope studies. D. P. H. and K. D. wrote the manuscript with suggestions from A. P. and T. S. All authors have given approval to the final version of the manuscript.
Conflicts of interest
There are no conflicts to declare.
Acknowledgements
Financial support from the Science and Engineering Research Board (SERB), Government of India (File CRG/2022/007372), is greatly acknowledged. D. P. H. thanks the Indian Institute of Science (IISc) Bangalore for the infrastructure. T. S. thanks the Ministry of Education, Government of India for PMRF.
Notes and references
-
(a) E. M. Carreira and T. C. Fessard, Chem. Rev., 2014, 114, 8257–8322 CrossRef CAS PubMed;
(b) Y.-J. Zheng and C. M. Tice, Expert Opin. Drug Discovery, 2016, 11, 831–834 CrossRef PubMed;
(c) A. J. Boddy and J. A. Bull, Org. Chem. Front., 2021, 8, 1026–1084 RSC;
(d) K. Hiesinger, D. Dar'in, E. Proschak and M. Krasavin, J. Med. Chem., 2021, 64, 150–183 CrossRef CAS PubMed;
(e) N. Moshnenko, A. Kazantsev, E. Chupakhin, O. Bakulina and D. Dar'in, Synthetic Routes to Approved Drugs Containing a Spirocycle, Molecules, 2023, 28, 4209 CrossRef CAS PubMed.
- K. E. Prosser, R. W. Stokes and S. M. Cohen, ACS Med. Chem. Lett., 2020, 11, 1292–1298 CrossRef CAS PubMed.
-
(a) D. E. Rathkopf and H. I. Scher, Expert Rev. Anticancer Ther., 2018, 18, 823–836 CrossRef CAS PubMed;
(b) M. A. Rice, S. V. Malhotra and T. Stoyanova, Front. Oncol., 2019, 9, 801 CrossRef PubMed;
(c) M. R. van der Kolk, M. A. C. H. Janssen, F. P. J. T. Rutjes and D. Blanco-Ania, ChemMedChem, 2022, 17, e202200020 CrossRef CAS PubMed.
- Y. Zheng, C. M. Tice and S. B. Singh, Bioorg. Med. Chem. Lett., 2014, 24, 3673–3682 CrossRef CAS PubMed.
- Selected patents on spirocyclobutyl lactones:
(a)
D. J. Canney, B. E. Blass, R. Gao and M. Abou-Gharbia, Novel 5-Hydroxytryptamine Receptor 7 Activity Modulators and Their Method of Use, WO2016040554A1, 2016;
(b)
D. J. Canney, B. E. Blass, R. Gao and K. Blattner, Novel Sigma-2 Receptor Binders and Their Method of Use, WO2016183150A1, 2016;
(c)
S. Angle, J. Cao, J. Come, L. A. Dakin, Z. Gale-Day, E. B. Krueger, J. H. Olsen, T. J. Senter, A. J. Shimizu, S. D. Stone and T. Wang, 2-Methyl-4′,5′-Dihydrospiro[Piperidine-4,7′-Thieno[2,3-C]Pyran] Derivatives as Inhibitors of Apol1 and Methods of Using Same, WO2023154344A1, 2023, selected patents on spirocyclobutyl lactams;
(d)
J. Caravella, B. Han, C. Liu, S. Ioannidis, A. J. Buckmelter, D. J. Richard, M. W. Martin, S. Mischke and S. Mente, Inhibiting Ubiquitin Specific Peptidase 30, EP3692028A1, 2020;
(e)
F. Jakob, J. Alen, S. Krüger, D. Friebe, S. Hennen and P. Barbie, Substituted Pyrrolidine Amides III, US2022089573A1, 2022;
(f)
S. Liu, Z. Yu, W. Pang, J. Wang and P. Chen, Highly Active CSF1R Inhibitor Compound, US11591328B2, 2023.
-
(a) M. Bertrand, A. Meou and A. Tubul, Tetrahedron Lett., 1982, 23, 3691–3694 CrossRef CAS;
(b) A. Call, M. Cianfanelli, P. Besalú-Sala, G. Olivo, A. Palone, L. Vicens, X. Ribas, J. M. Luis, M. Bietti and M. Costas, J. Am. Chem. Soc., 2022, 144, 19542–19558 CrossRef CAS PubMed;
(c) Z. Zhang and V. Gevorgyan, J. Am. Chem. Soc., 2022, 144, 20875–20883 CrossRef CAS PubMed.
-
(a) J. Turkowska, J. Durka and D. Gryko, Chem. Commun., 2020, 56, 5718–5734 RSC;
(b) J. L. Tyler and V. K. Aggarwal, Chem.–Eur. J., 2023, 29, e202300008 CrossRef CAS PubMed;
(c) C. B. Kelly, J. A. Milligan, L. J. Tilley and T. M. Sodano, Chem. Sci., 2022, 13, 11721–11737 RSC;
(d) P. Bellotti and F. Glorius, J. Am. Chem. Soc., 2023, 145, 20716–20732 CrossRef CAS PubMed.
-
(a) M. A. A. Walczak, T. Krainz and P. Wipf, Acc. Chem. Res., 2015, 48, 1149–1158 CrossRef CAS PubMed;
(b) M. Golfmann and J. C. L. Walker, Commun. Chem., 2023, 6, 9 CrossRef CAS PubMed.
-
(a) Y. Gaoni, A. Tomazic and E. Potgieter, J. Org. Chem., 1985, 50, 2943–2947 CrossRef CAS;
(b) T. G. Archibald, L. C. Garver, K. Baum and M. C. Cohen, J. Org. Chem., 1989, 54, 2869–2873 CrossRef CAS;
(c) R. Gianatassio, J. M. Lopchuk, J. Wang, C.-M. Pan, L. R. Malins, L. Prieto, T. A. Brandt, M. R. Collins, G. M. Gallego, N. W. Sach, J. E. Spangler, H. Zhu, J. Zhu and P. S. Baran, Science, 2016, 351, 241–246 CrossRef CAS PubMed;
(d) J. A. Milligan, C. A. Busacca, C. H. Senanayake and P. Wipf, Org. Lett., 2016, 18, 4300–4303 CrossRef CAS;
(e) J. M. Lopchuk, K. Fjelbye, Y. Kawamata, L. R. Malins, C.-M. Pan, R. Gianatassio, J. Wang, L. Prieto, J. Bradow, T. A. Brandt, M. R. Collins, J. Elleraas, J. Ewanicki, W. Farrell, O. O. Fadeyi, G. M. Gallego, J. J. Mousseau, R. Oliver, N. W. Sach, J. K. Smith, J. E. Spangler, H. Zhu, J. Zhu and P. S. Baran, J. Am. Chem. Soc., 2017, 139, 3209–3226 CrossRef CAS PubMed;
(f) X. Wu, W. Hao, K.-Y. Ye, B. Jiang, G. Pombar, Z. Song and S. Lin, J. Am. Chem. Soc., 2018, 140, 14836–14843 CrossRef CAS PubMed;
(g) A. Fawcett, T. Biberger and V. K. Aggarwal, Nat. Chem., 2019, 11, 117–122 CrossRef CAS PubMed;
(h) M. Silvi and V. K. Aggarwal, J. Am. Chem. Soc., 2019, 141, 9511–9515 CrossRef CAS PubMed;
(i) S. H. Bennett, A. Fawcett, E. H. Denton, T. Biberger, V. Fasano, N. Winter and V. K. Aggarwal, J. Am. Chem. Soc., 2020, 142, 16766–16775 CrossRef CAS PubMed;
(j) G. Ernouf, E. Chirkin, L. Rhyman, P. Ramasami and J.-C. Cintrat, Angew. Chem., Int. Ed., 2020, 59, 2618–2622 CrossRef CAS PubMed;
(k) M. Ociepa, A. J. Wierzba, J. Turkowska and D. Gryko, J. Am. Chem. Soc., 2020, 142, 5355–5361 CrossRef CAS PubMed;
(l) C. J. Pratt, R. A. Aycock, M. D. King and N. T. Jui, Synlett, 2020, 31, 51–54 CrossRef CAS PubMed;
(m) B. D. Schwartz, M. Y. Zhang, R. H. Attard, M. G. Gardiner and L. R. Malins, Chem.–Eur. J., 2020, 26, 2808–2812 CrossRef CAS PubMed;
(n) X. Yu, M. Lübbesmeyer and A. Studer, Angew. Chem., Int. Ed., 2021, 60, 675–679 CrossRef CAS;
(o) G. Tan, M. Das, H. Keum, P. Bellotti, C. Daniliuc and F. Glorius, Nat. Chem., 2022, 14, 1174–1184 CrossRef CAS;
(p) J. Majhi, R. K. Dhungana, Á. Rentería-Gómez, M. Sharique, L. Li, W. Dong, O. Gutierrez and G. A. Molander, J. Am. Chem. Soc., 2022, 144, 15871–15878 CrossRef CAS PubMed;
(q) A. Guin, S. Bhattacharjee, M. S. Harariya and A. T. Biju, Chem. Sci., 2023, 14, 6585–6591 RSC;
(r) H. D. Pickford, V. Ripenko, R. E. McNamee, S. Holovchuk, A. L. Thompson, R. C. Smith, P. K. Mykhailiuk and E. A. Anderson, Angew. Chem., Int. Ed., 2023, 62, e202213508 CrossRef CAS PubMed;
(s) W. Huang, Y. Zheng, S. Keess and G. A. Molander, J. Am. Chem. Soc., 2023, 145, 5363–5369 CrossRef CAS PubMed;
(t) H.-C. Shen, M. V. Popescu, Z.-S. Wang, L. de Lescure, A. Noble, R. S. Paton and V. K. Aggarwal, J. Am. Chem. Soc., 2023, 145, 16508–16516 CrossRef CAS PubMed;
(u) H. Wang, J. E. Erchinger, M. Lenz, S. Dutta, C. G. Daniliuc and F. Glorius, J. Am. Chem. Soc., 2023, 145, 23771–23780 CrossRef CAS PubMed.
-
(a) R. M. Bychek, V. Hutskalova, Y. P. Bas, O. A. Zaporozhets, S. Zozulya, V. V. Levterov and P. K. Mykhailiuk, J. Org. Chem., 2019, 84, 15106–15117 CrossRef CAS PubMed;
(b) X. Ma, D. L. Sloman, Y. Han and D. J. Bennett, Org. Lett., 2019, 21, 7199–7203 CrossRef CAS PubMed;
(c) R. E. McNamee, M. M. Haugland, J. Nugent, R. Chan, K. E. Christensen and E. A. Anderson, Chem. Sci., 2021, 12, 7480–7485 RSC;
(d) R. Bychek and P. K. Mykhailiuk, Angew. Chem., Int. Ed., 2022, 61, e202205103 CrossRef CAS PubMed.
-
(a) M. Ueda, M. A. A. Walczak and P. Wipf, Tetrahedron Lett., 2008, 49, 5986–5989 CrossRef CAS PubMed;
(b) K. Dhake, K. J. Woelk, J. Becica, A. Un, S. E. Jenny and D. C. Leitch, Angew. Chem., Int. Ed., 2022, 61, e202204719 CrossRef CAS PubMed;
(c) Z. Ding, Z. Liu, Z. Wang, T. Yu, M. Xu, J. Wen, K. Yang, H. Zhang, L. Xu and P. Li, J. Am. Chem. Soc., 2022, 144, 8870–8882 CrossRef CAS PubMed;
(d) R. Guo, Y.-C. Chang, L. Herter, C. Salome, S. E. Braley, T. C. Fessard and M. K. Brown, J. Am. Chem. Soc., 2022, 144, 7988–7994 CrossRef CAS PubMed;
(e) R. Kleinmans, T. Pinkert, S. Dutta, T. O. Paulisch, H. Keum, C. G. Daniliuc and F. Glorius, Nature, 2022, 605, 477–482 CrossRef CAS PubMed;
(f) Y. Liang, R. Kleinmans, C. G. Daniliuc and F. Glorius, J. Am. Chem. Soc., 2022, 144, 20207–20213 CrossRef CAS PubMed;
(g) B. D. Schwartz, A. P. Smyth, P. E. Nashar, M. G. Gardiner and L. R. Malins, Org. Lett., 2022, 24, 1268–1273 CrossRef CAS PubMed;
(h) Y. Zheng, W. Huang, R. K. Dhungana, A. Granados, S. Keess, M. Makvandi and G. A. Molander, J. Am. Chem. Soc., 2022, 144, 23685–23690 CrossRef CAS PubMed;
(i) S. Agasti, F. Beltran, E. Pye, N. Kaltsoyannis, G. E. M. Crisenza and D. J. Procter, Nat. Chem., 2023, 15, 535–541 CrossRef CAS PubMed;
(j) R. Kleinmans, S. Dutta, K. Ozols, H. Shao, F. Schäfer, R. E. Thielemann, H. T. Chan, C. G. Daniliuc, K. N. Houk and F. Glorius, J. Am. Chem. Soc., 2023, 145, 12324–12332 CrossRef CAS PubMed;
(k) T. Yu, J. Yang, Z. Wang, Z. Ding, M. Xu, J. Wen, L. Xu and P. Li, J. Am. Chem. Soc., 2023, 145, 4304–4310 CrossRef CAS;
(l) Y. Liu, S. Lin, Y. Li, J.-H. Xue, Q. Li and H. Wang, ACS Catal., 2023, 13, 5096–5103 CrossRef CAS;
(m) N. Radhoff, C. G. Daniliuc and A. Studer, Angew. Chem., Int. Ed., 2023, 62, e202304771 CrossRef CAS PubMed;
(n) Y. Liang, F. Paulus, C. G. Daniliuc and F. Glorius, Angew. Chem., Int. Ed., 2023, 62, e202305043 CrossRef CAS PubMed;
(o) T. V. T. Nguyen, A. Bossonnet, M. D. Wodrich and J. Waser, J. Am. Chem. Soc., 2023, 145, 25411–25421 CrossRef CAS.
- N. A. Meanwell, J. Agric. Food Chem., 2023, 71, 18087–18122 CrossRef CAS PubMed.
- S. Stotani, C. Lorenz, M. Winkler, F. Medda, E. Picazo, R. Ortega Martinez, A. Karawajczyk, J. Sanchez-Quesada and F. Giordanetto, ACS Comb. Sci., 2016, 18, 330–336 CrossRef CAS PubMed.
- G. Tan, F. Paulus, A. Petti, M.-A. Wiethoff, A. Lauer, C. Daniliuc and F. Glorius, Chem. Sci., 2023, 14, 2447–2454 RSC.
- Y. Lu, C. Chen, H. Zhu, Z. Luo and Y. Zhang, Green Chem., 2022, 24, 8021–8028 RSC.
- V. A. Vasin, A. V. Semenov, S. G. Kostryukov and V. V. Razin, Russ. J. Org. Chem., 2008, 44, 1296–1304 CrossRef CAS.
- E. D. Hughes and C. K. Ingold, J. Chem. Soc., 1935, 244–255 RSC.
-
(a) M. Teders, C. Henkel, L. Anhäuser, F. Strieth-Kalthoff, A. Gómez-Suárez, R. Kleinmans, A. Kahnt, A. Rentmeister, D. Guldi and F. Glorius, Nat. Chem., 2018, 10, 981–988 CrossRef CAS PubMed;
(b) R. M. Bär, G. Heinrich, M. Nieger, O. Fuhr and S. Bräse, Beilstein J. Org. Chem., 2019, 15, 1172–1180 CrossRef PubMed;
(c) C. Wang, Y. Zhang, K. Sun, T. Yu, F. Liu and X. Wang, Molecules, 2023, 28, 1–35 Search PubMed.
- H. Chuai, S.-Q. Zhang, H. Bai, J. Li, Y. Wang, J. Sun, E. Wen, J. Zhang and M. Xin, Eur. J. Med. Chem., 2021, 223, 113621 CrossRef CAS PubMed.
-
(a) J. Gong and P. L. Fuchs, J. Am. Chem. Soc., 1996, 118, 4486–4487 CrossRef CAS;
(b) L. Liang, G. Guo, C. Li, S.-L. Wang, Y.-H. Wang, H.-M. Guo and H.-Y. Niu, Org. Lett., 2021, 23, 8575–8579 CrossRef CAS PubMed.
-
(a) C. Zhu, H. Yue, L. Chu and M. Rueping, Chem. Sci., 2020, 11, 4051–4064 RSC;
(b) S. O. Badir and G. A. Molander, Chem, 2020, 6, 1327–1339 CrossRef CAS PubMed;
(c) M. Marchi, G. Gentile, C. Rosso, M. Melchionna, P. Fornasiero, G. Filippini and M. Prato, ChemSusChem, 2022, 15, e202201094 CrossRef CAS PubMed;
(d) Z. Zuo, D. T. Ahneman, L. Chu, J. A. Terrett, A. G. Doyle and D. W. C. MacMillan, Science, 2014, 345, 437–440 CrossRef CAS PubMed;
(e) E. B. Corcoran, M. T. Pirnot, S. Lin, S. D. Dreher, D. A. DiRocco, I. W. Davies, S. L. Buchwald and D. W. C. MacMillan, Science, 2016, 353, 279–283 CrossRef CAS PubMed;
(f) X. Zhang, R. T. Smith, C. Le, S. J. McCarver, B. T. Shireman, N. I. Carruthers and D. W. C. MacMillan, Nature, 2020, 580, 220–226 CrossRef CAS;
(g) Z. Dong and D. W. C. MacMillan, Nature, 2021, 598, 451–456 CrossRef CAS PubMed;
(h) C. N. Prieto Kullmer, J. A. Kautzky, S. W. Krska, T. Nowak, S. D. Dreher and D. W. C. MacMillan, Science, 2022, 376, 532–539 CrossRef PubMed.
-
(a)
S. Hoelder, J. Blagg, S. Solanki, H. Woodward, S. Naud, V. Bavetsias, P. Sheldrake, P. Innocenti, J. Cheung and B. Atrash, Inhibitor Compounds, WO2014037750A1, 2014;
(b)
G. A. Brown, J. E. Cansfield, M. S. Congreve, M. A. O'brien, M. Pickworth, M. D. Rackham, B. G. Tehan and B. J. Teobold, Bicyclic Aza Compounds as Muscarinic M1 Receptor Agonists, WO2015118342A1, 2015;
(c)
F. Wagner and D. Hickman, Hdac6 Inhibitors and Uses Thereof, WO2023150203A1, 2023.
-
(a) H. Ruan, L.-G. Meng, L. Zhu and L. Wang, Adv. Synth. Catal., 2019, 361, 3217–3222 CrossRef CAS;
(b) S.-Y. Tian, J.-J. Ai, J.-H. Han, W. Rao, S.-S. Shen, D. Sheng and S.-Y. Wang, J. Org. Chem., 2023, 88, 828–837 CrossRef CAS PubMed.
- A. U. Meyer, S. Jäger, D. Prasad Hari and B. König, Adv. Synth. Catal., 2015, 357, 2050–2054 CrossRef CAS.
-
(a) E. Speckmeier, T. G. Fischer and K. Zeitler, J. Am. Chem. Soc., 2018, 140, 15353–15365 CrossRef CAS PubMed;
(b) X. Du, I. Cheng-Sánchez and C. Nevado, J. Am. Chem. Soc., 2023, 145, 12532–12540 CrossRef CAS PubMed.
- S. H. Lau, M. A. Borden, T. J. Steiman, L. S. Wang, M. Parasram and A. G. Doyle, J. Am. Chem. Soc., 2021, 143, 15873–15881 CrossRef CAS PubMed.
-
(a) I. De Riggi, S. Gastaldi, J. M. Surzur, M. P. Bertrand and A. Virgili, J. Org. Chem., 1992, 57, 6118–6125 CrossRef CAS;
(b) T. Naito, Y. Honda, O. Miyata and I. Ninomiya, J. Chem. Soc., Perkin Trans. 1, 1995, 19–26 RSC.
|
This journal is © The Royal Society of Chemistry 2024 |
Click here to see how this site uses Cookies. View our privacy policy here.