DOI:
10.1039/D3SC05738K
(Perspective)
Chem. Sci., 2024,
15, 2300-2322
Biocompatible strategies for peptide macrocyclisation
Received
27th October 2023
, Accepted 4th January 2024
First published on 5th January 2024
Abstract
Peptides are increasingly important drug candidates, offering numerous advantages over conventional small molecules. However, they face significant challenges related to stability, cellular uptake and overall bioavailability. While individual modifications may not address all these challenges, macrocyclisation stands out as a single modification capable of enhancing affinity, selectivity, proteolytic stability and membrane permeability. The recent successes of in situ peptide modifications during screening in combination with genetically encoded peptide libraries have increased the demand for peptide macrocyclisation reactions that can occur under biocompatible conditions. In this perspective, we aim to distinguish biocompatible conditions from those well-known examples that are fully bioorthogonal. We introduce key strategies for biocompatible peptide macrocyclisation and contextualise them within contemporary screening methods, providing an overview of available transformations.
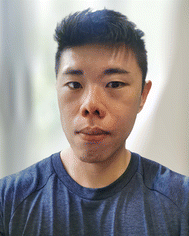 Junming He | Junming He received his BSc (Hons) in Medicinal Chemistry from the University of Auckland in 2018. He completed his PhD in Chemistry at the Research School of Chemistry, Australian National University (ANU) in Canberra in 2023. His research encompasses the use of organic and medicinal chemistry to investigate bioactive molecules for drug development. |
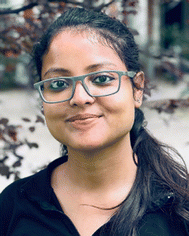 Pritha Ghosh | Pritha Ghosh is a Postdoctoral Research Fellow at the Research School of Chemistry, Australian National University (ANU) in Canberra. She received her PhD in 2022 from the Indian Institute of Science, Bangalore where she worked on amide backbone modifications to improve pharmacological properties of cyclic peptides. Her current research at the ANU focuses on the design and chemical synthesis of new classes of constrained peptides and proteins targeting infectious diseases. |
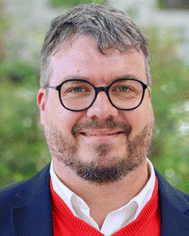 Christoph Nitsche | Christoph Nitsche is Associate Professor and ARC Future Fellow at the Research School of Chemistry, Australian National University (ANU) in Canberra. He obtained his PhD in 2014 from Heidelberg University and was a Feodor Lynen (2015–2018) and ARC DECRA Fellow (2019–2022) at the ANU before assuming a faculty position in 2020. His research revolves around peptide and protein modification, alongside drug discovery efforts targeting infectious diseases. |
Introduction
Macrocyclic peptides as therapeutics
The discovery of insulin therapy in the 1920s marked a major milestone in peptide therapeutics. Banting & Best were able to isolate insulin from the pancreatic isles of animals and used it for the treatment of type 1 diabetes.1 Other examples of early peptide therapeutics include, for example, adrenocorticotropic hormone (ACTH) for the ACTH stimulation test,2 salmon calcitonin for the treatment of Paget's disease and osteoporosis,3 oxytocin for inducing childbirth,4 vasopressin for the treatment of vasodilatory shock,5 and octreotide for the treatment of acromegaly and neuroendocrine tumours.6 Over time, an increasing number of peptide therapeutics successfully transitioned into the clinic. Between 2017 and 2023, approximately one in ten drugs approved by the FDA were peptides and peptidomimetics, with macrocyclic peptides accounting for nearly half of this category (Table S1† and Fig. 1).7–12
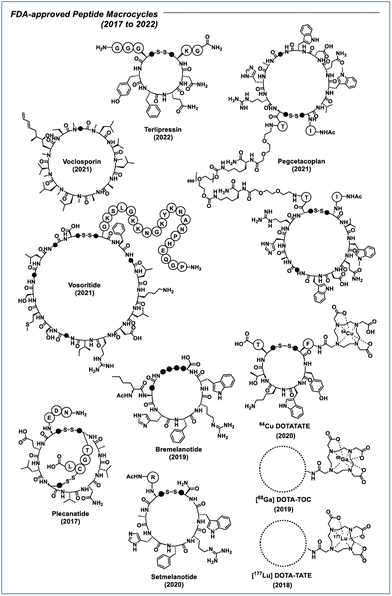 |
| Fig. 1 Peptide macrocycles approved by the US Food and Drug Administration (FDA) between 2017 and 2022. Each • represents a CH2 (methylene) group. | |
One reason why macrocyclic peptides have gathered such increasing attention within the pharmaceutical industries is due to their superior properties, frequently surpassing those of their linear counterparts.13–19 Peptide macrocycles may have enhanced bioactivity and lower toxicity along with improved affinity for their targets. Unlike random-coil (linear) peptides, macrocycles are constrained by their ring structure, reducing the number of conformations which in turn reduces the entropic penalty upon binding, thereby increasing selectivity and affinity at the target binding site. Additionally, macrocyclic peptides may exhibit greater resistance to proteolysis and enhanced cell-penetrating activity. In summary, simple macrocyclisation may lead to improved affinity, selectivity, stability and membrane permeability, which are four crucial parameters for successful drug candidates. Furthermore, macrocyclic peptides can be generated for virtually any drug target, thanks to the rapidly expanding toolbox for de novo discovery of macrocyclic ligands.
Types of macrocycles and examples from nature
Peptide macrocycles commonly exhibit distinct cyclisation patterns, which can be categorised into head-to-tail, side chain-to-side chain and termini-to-side chain (Fig. 2). Head-to-tail cyclic peptides are characterised by forming a closed loop that connects the C- and N-termini of the peptide. The points of cyclisation can usually not be determined in such a macrocycle. Prominent examples include cyclosporin A, gramicidin S and yunnanin A. While a homodetic bond refers specifically to such a loop formed through a head-to-tail macrolactam cyclisation,20 all other macrocycles formed between two functional groups are considered heterodetic.21 Side chain-to-side chain cyclisation involves connecting two side chain residues, as observed in somatostatin, oxytocin, salmon calcitonin and the crustacean cardioactive peptide. Moreover, termini-to-side chain cyclisation connects either the C- or N-terminus to one of the side chain residues, as observed, for example, in the antibiotic polymyxin B.22,23 There are special cases where a peptide macrocycle contains multiple loops or is connected at multiple points within the peptide sequence, which are referred to as bicycles and tricycles (Fig. 2). Naturally occurring peptide macrocycles have been isolated from various organisms, including marine resources (invertebrates),24,25 bacteria,26–28 plants,29 fungi30 and mammals.31,32 These peptides serve as hormones, growth factors or defensive mechanisms against competing microbes (i.e., antibiotics and toxins). We recently reviewed contemporary methods to synthesise bicyclic peptides.33
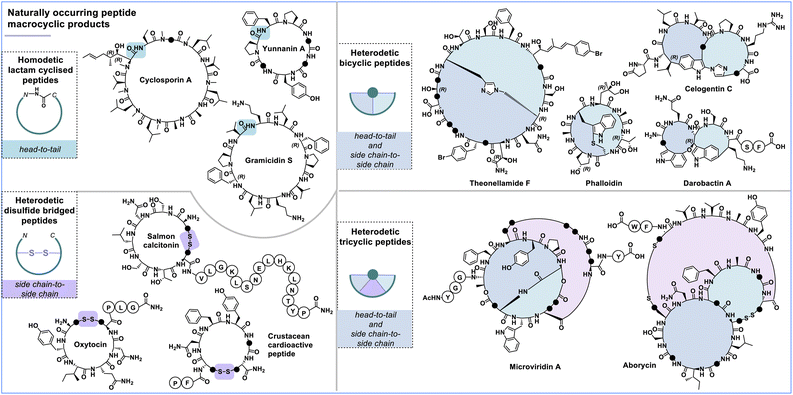 |
| Fig. 2 Examples of peptide macrocycles found in nature categorised by their cycle type and chemical linkage. Each • represents a CH2 (methylene) group. | |
The importance of biocompatible macrocyclisation strategies
The innovation of solid-phase peptide synthesis (SPPS) by Merrifield in the 1960s radically revolutionised the chemical synthesis of peptides via a facile, efficient and automated platform, enabling large scale production of bioactive peptides.34,35 Chemical synthesis has long been the cornerstone for establishing structure–activity relationships well before genetically encoded peptide libraries gained prominence. It has been a fundamental tool in understanding the relationship between the chemical structure and the biological activity of peptides. The modification of peptides in the presence of their target protein has enabled the in situ generation and selection of peptide ligands.36–38 Furthermore, peptide display techniques, such as phage or mRNA display,39,40 have the capability to screen billions of peptides against drug targets. However, these screenings require chemical conditions that support the structural and functional integrity of biopolymers (e.g., proteins, nucleic acids) involved.
Commonly used strategies for peptide macrocyclisation are highlighted in Fig. 3, covering a wide range of chemical linkages and reactions discussed in this perspective. Certain approaches require rather harsh and nonselective conditions, while others can proceed under very benign and selective conditions, even within a complex cellular environment. The latter chemical transformations are usually considered bioorthogonal, with the [3 + 2]-cycloaddition reaction between azide and alkyne motifs being the most prominent example.41 The importance of this click reaction and bioorthogonal chemistry more generally was recently recognised by The Nobel Prize in chemistry awarded to Bertozzi, Meldal and Sharpless.42
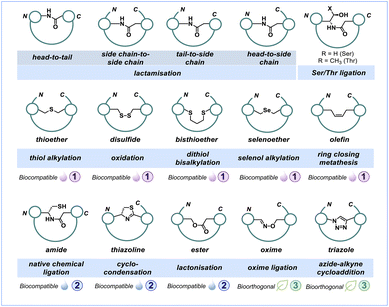 |
| Fig. 3 Various types of cyclic peptides and selected synthetic strategies for cyclisation. | |
While bioorthogonal chemistry plays a crucial role in numerous applications,43,44 not every reaction used to modify or cyclise peptides needs to be fully bioorthogonal. Inspired by Zhou & co-workers from the Chemical Abstracts Service (CAS),43 who recently reported criteria for bioorthogonal reactions, we defined the following characteristics for biocompatible and bioorthogonal reaction used throughout this perspective:
In fact, fulfilling only the first criterion listed above – a reaction that occurs in water at physiological pH and temperature – deems a reaction already biocompatible, as defined in this perspective article. Furthermore, it is highly desirable for a biocompatible reaction to meet the second criterion as closely as possible. Peptide display techniques often require robust reactions with minimal side products. Selectivity for certain amino acids in peptides and proteins, or even complete orthogonality to common endogenous nucleophiles and electrophiles, can be of great benefit. When screenings are performed in cells, considering toxicity and redox stability becomes an additional important factor. While reaction rate at low concentrations is a crucial factor for transformations used in bioconjugation, it is of lesser importance for macrocyclisation. Intramolecular reactions typically proceed faster than intermolecular reactions, and they are independent of concentration. In fact, lower concentrations can be desirable in macrocyclisation to suppress the formation of oligomers.
In this perspective article, our main objective is to highlight the most important strategies employed in peptide macrocyclisation with a particular focus on biocompatible methods. While some of these methods are routinely used for modifying peptides and proteins in biological environments, others hold potential for future applications. We will adhere to the definitions mentioned above, labelling reactions as biocompatible and bioorthogonal if they broadly meet the criteria. However, it is not our intention to clearly distinguish between biocompatible and bioorthogonal reactions, as current classifications are not definitive. Comprehensive reviews that consolidate current developments and challenges in this field are unavailable. Therefore, we have undertaken the task of reviewing conventional methods and highlighting emerging trends in biocompatible strategies for peptide macrocyclisation.
Strategies for peptide macrocyclisation
Macrolactam cyclisation
As outlined above, homodetic cyclic peptides are cyclised via an amide bond, resulting in a macrolactam ring. Ribosomes facilitate the biosynthesis of polypeptides (proteins) through a complex biomolecular machinery that forms amide bonds by linking α-amino acids as illustrated in Scheme 1A and B.45,46 In this process of translation from RNA to proteins, nature utilises an ester bond between the terminal amino acid of the growing peptide chain and its corresponding tRNA for activation (P-site) in order to react with the amine of the incoming amino acid (A-site). While translation proceeds with fully unprotected amino acids under physiological conditions with a speed of 20 amino acids per second,47 synthetic chemistry usually requires bulky protecting groups, additives, sensitising coupling reagents and excessive volumes of toxic organic solvents to generate peptide chains over many hours if not days.48–51
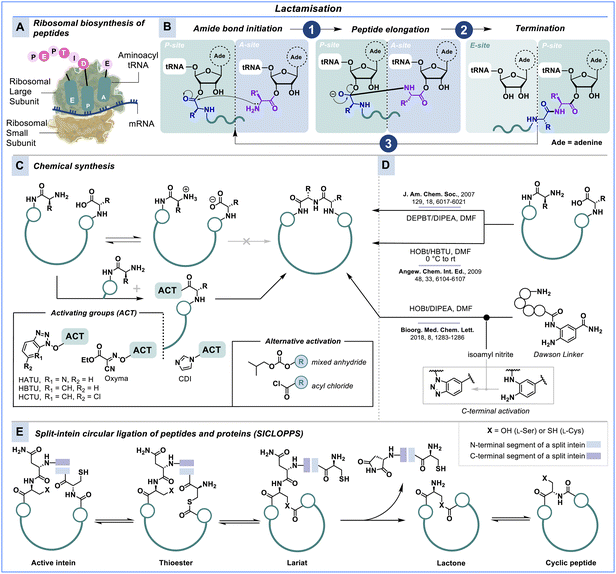 |
| Scheme 1 Amide bond formation and macrolactam cyclisation. [A] Ribosomal translation from mRNA to proteins. [B] Addition of an amino acid to the growing peptide chain in the ribosome. [C] Chemical synthesis of peptides via activation of carboxylic acids. [D] Examples of synthetic methods for macrolactam cyclisation. [E] Split-intein circular ligation. | |
Given its significance in translation and the formation of naturally occurring cyclic peptides, amide bond formation is a widely employed and important method for constructing macrocyclic peptides;52–55 however, most of these chemical strategies are bioincompatible (Scheme 1C). For example, Smith & co-workers used diphenylphosphoryl azide (DPPA) as coupling agent in DMF to form a tricyclic homodetic peptide.56 Peptide macrocyclisation using DPPA also tolerates backbone modifications like N-methylation or thioamides, as demonstrated by Kessler, Chatterjee & co-workers.57,58 The bicyclic octapeptide celogentin C was synthesised using HBTU as the coupling agent in DMF,59 and the antibiotic peptide lysobactin was accessed by treating the linear peptide with DEPBT in DMF.60 The process of macrolactamisation is slow, as illustrated in these examples, taking from ten hours to three days to form the macrolactam ring. Alternatively, macrolactam cycles can be generated using the Dawson linker (Fmoc–Dbz) introduced in 2008.61 Oishi & co-workers furnished a head-to-tail macrolactam cyclised peptide using this versatile linker.62 Following C-terminal activation, head-to-tail macrocyclisation was achieved in only 2 h at room temperature in DMF (Scheme 1D).
The lack of biocompatible synthetic approaches for macrolactam cyclisation is one of the main reasons why peptide display technologies like phage or mRNA display rely on non-canonical cyclisation methods, as discussed below. An alternative strategy for genetically encoded macrocyclic peptide libraries that supports pure head-to-tail macrocyclisation is the split-intein circular ligation of peptides and proteins (SICLOPPS), which uses protein splicing to generate cyclic peptide libraries (Scheme 1E).40,63,64
Disulfide bond formation
An alternative way to cyclise peptides commonly observed in nature is through the formation of disulfide bonds between cysteine residues. Examples include oxytocin, which forms a cyclic peptide from one disulfide bond (Fig. 1),4 and cyclotides, which are macrolactam cyclic peptides that possess three internal disulfide bonds, making them a highly constrained class of peptides (Scheme 2A).65,66 Disulfide bond formation is dependent on the spatial proximity of the two cysteine residues, the pH and the redox environment. Alkaline pH promotes disulfide formation due to increased cysteine nucleophilicity (pKa = 8.5). Air is sufficient for oxidation to disulfides; however, since the intracellular environment is mostly reducing (e.g., glutathione), most disulfide bonds are unstable in the cytosol.
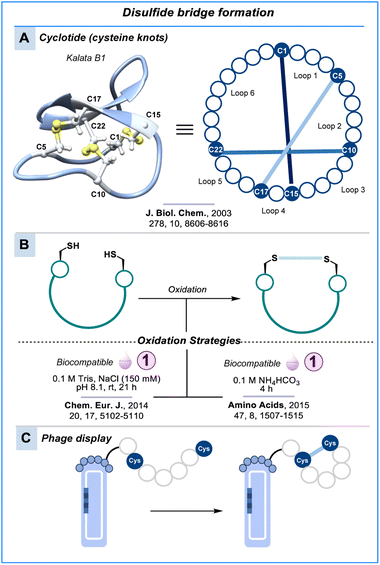 |
| Scheme 2 Disulfide bridge formation. [A] The cyclotide kalata B1 is an example for a highly constrained macrocyclic peptide with three disulfide bonds. [B] Examples of biocompatible chemical conditions to induce disulfide bridge formation by oxidation. [C] Formation of cyclic peptides in phage display using disulfide bonds. | |
Despite their susceptibility to reducing conditions, including enzymes like reductases,67,68 disulfide-bridged peptides have received widespread attention due to their diverse bioactivity, ability for cell penetration, proteolytic stability and resilience to some harsh conditions such as high temperature, acidic pH and chaotropic agents.69
Synthetic methods to form disulfides rely on the simple oxidation of cysteine residues. Often, these methods can proceed in buffer at near-neutral pH and room temperature, deeming them highly biocompatible (Scheme 2B). For example, oxidative folding of cysteine-rich peptides can be accomplished in Tris buffer at pH 8.0, as illustrated in the folding of the 63-mer snakin-1 peptide at room temperature over 21 h.70 The oxidative folding of a CXCR4 antagonist peptide followed a similar approach using NH4HCO3 buffer.71 Alternatively, using iodine as an oxidising agent, cysteine-rich peptides can rapidly cyclise within only a few minutes.72–74 The liability of disulfide bonds to reducing conditions has motivated chemists to explore various bioisosteric crosslinks.75–78
An area of significant importance where disulfide bonds have been used routinely to form macrocyclic peptides under biocompatible conditions is phage display (Scheme 2C).40,79 As the panning and modification of the phage occur outside the cellular environment, the disulfide bond is useful to constrain peptides for screening purposes. Recently, the strategy was expanded to highly constrained disulfide-directed multicyclic peptide libraries.80
Thio- and selenoether formation
A modification involving cysteine (and occasionally selenocysteine) that forms chemically more stable conjugates than the previously discussed disulfide bond is thioether formation. Naturally occurring antimicrobial peptides like lanthipeptides and sactipeptides feature such a thioether bridge.81 In nature, phosphorylated serine or threonine residues undergo enzymatic dehydration and subsequent Michael reaction with a cysteine residue to form the lanthipeptide bridge (Scheme 3A).82,83 In the past, lanthipeptides were synthesised using Fmoc-SPPS compatible building block strategies;77,84–87 however, with the advent of biocompatible strategies, libraries of lanthipeptides with multiple thioether crosslinks can be generated using phage and yeast display platforms.88,89
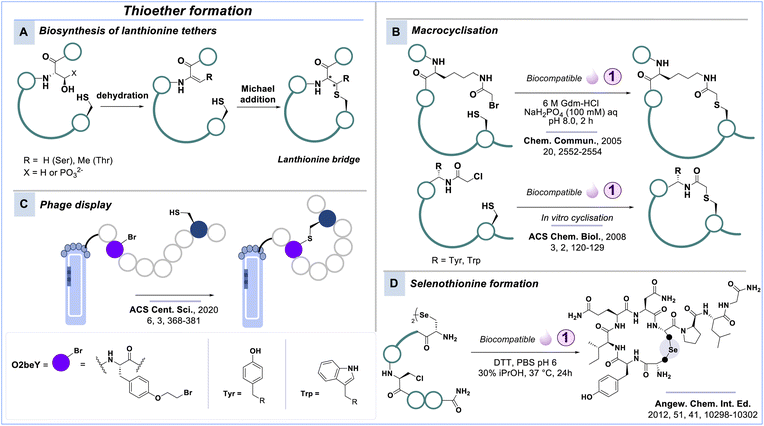 |
| Scheme 3 Thioether macrocyclisation. [A] Biosynthetic formation of the lanthionine bridge. [B] Examples of biocompatible strategies used to form thioethers in macrocyclic peptides. [C] Macrocyclic Organo-Peptide Hybrid Phage Display (MOrPH-PhD). [D] Example for alternative selenoether formation. | |
Brunel and Dawson90 synthesised helical peptides with thioether staples by alkylating a cysteine residue with a primary alkyl halide in aqueous buffer at pH 8.4 and room temperature (Scheme 3B). This biocompatible methodology was later adapted in the seminal works of Suga & co-workers that combine an engineered ribozyme (flexizyme) with the in vitro mRNA display technique in the Random Nonstandard Peptides Integrated Discovery (RaPID).39,91–94 The RaPID platform allows reprogramming the genetic code to introduce noncanonical amino acids, for example, displaying α-N-chloroacetyl groups that spontaneously form thioethers with a downstream cysteine residue during in vitro translation (Scheme 3B).95–98
A related chemical approach was recently integrated into the phage display platform by Fasan & co-workers, in which they screened combinatorial libraries of thioether-bridged macrocycles against multiple protein targets and identified high affinity binders.99 In this strategy titled Macrocyclic Organo-Peptide Hybrid Phage Display (MOrPH-PhD), macrocyclisation of peptide libraries is aided by the regioselective and biocompatible reaction between a cysteine residue and O-(2-bromomethyl)-tyrosine (O2beY) (Scheme 3C). The approach requires three plasmids, one of which encodes the tRNA/aminoacyl-tRNA synthetase pair necessary for incorporation of the unnatural amino acid carrying the electrophile.
The alkylation of selenocysteine presents an intriguing alternative to using cysteine; however, it is important to consider the increased susceptibility of selenocysteine (pKa = 5.4) to oxidation and dimerisation compared to cysteine (pKa = 8.6). Alewood & co-workers successfully constructed a selenoether bridge in oxytocin using biocompatible conditions (Scheme 3D).100 Importantly, the cysteine analogue failed to form the same linkage, highlighting the valuable applications of selenocysteine alkylation.
Dithiol bisalkylation
Thioether modification of cysteine residues can also be accomplished in a dual manner through bisalkylation of two cysteines. This two-component peptide stapling approach has been employed to access various ring sizes, tackle metabolism, improve bioactivity and stabilise α-helical conformation.101,102 Cornish & co-workers103 prepared a series of cyclic amylin-(1–8) analogues with various linkers under biocompatible conditions (Scheme 4A). Dithiol bisalkylation was performed by incubating the linear peptides with two reduced cysteine residues in various aqueous buffers ranging from pH 7.8 to 8.8 with yields between 10% and 67%.
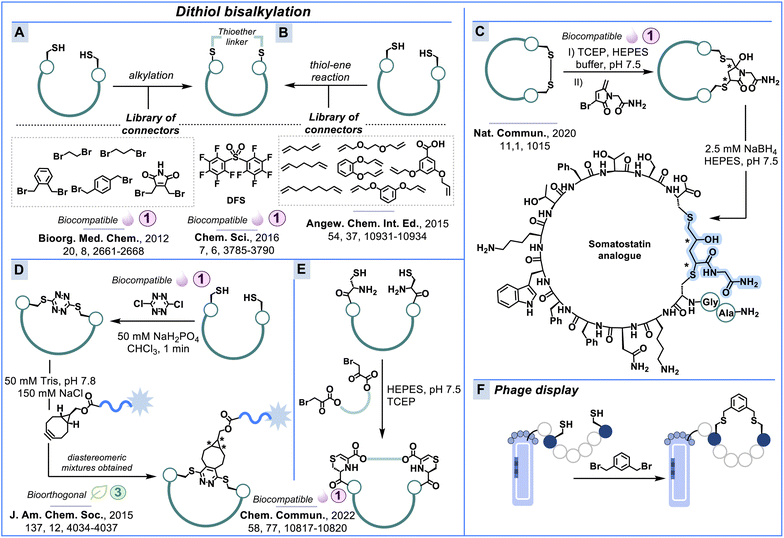 |
| Scheme 4 Peptide dithiol bisalkylation. Bridging two cysteine residues using [A] haloalkyl connectors or [B] di-enes to perform thiol–ene chemistry. [C] Cysteine stapling with a 3-bromo-5-methylene pyrrolone derivative. [D] S,S-Tetrazine staples allow further modifcation under bioorthogonal conditions. [F] Cysteine stapling during phage display with DBMB. | |
Derda & co-workers introduced decafluoro-biphenylsulfone (DFS) as a new perfluoroarene (fAr) for biocompatible peptide and protein modification (Scheme 4A).104 While many SNAr-reagents suffer from nonselective nucleophilic substitution, low reactivity and poor water solubility,105–107 DFS and perfluoropyridine are capable of site-selective modification in the presence of only low ratios of organic co-solvents, e.g., Tris-buffer with 5% DMF or 10% acetonitrile. DFS reacts quicker with cationic peptides (rate constant of 100–180 M−1 s−1) than uncharged peptides (50–80 M−1 s−1) and modified 35–45% of thiols in a library of >109 disulfide heptapeptides displayed on intact M13 bacteriophage. To select chemically stable peptide binders on phage, Heinis & co-workers have also explored cysteine alkylation using α,α′-dibromo-m-xylene (DBMB) (Scheme 4F).108 Peptide stapling with DBMB resulted in high-affinity ligands of β-catenin with α-helical conformation.
The thiol–ene reaction is an alternative way to alkylate cysteine residues using UV irradiation.109–111 Examples that implemented thiol–ene chemistry into peptide synthesis include the works of Wang and Chou109,112 and Beyermann & co-workers.113,114 Wang and Chou used a radical initiator in NMP as the solvent to afford various dithiol-bisalkylated peptide macrocycles in excellent yields (Scheme 4B). Beyermann & co-workers demonstrated that the thiol–ene reaction can proceed under biocompatible conditions using a photoswitchable amino acid with a vinyl handle. Spring & co-workers used divinyltriazine staples for biocompatible cysteine modification.115 Buchwald, Pentelute & co-workers further expanded the toolbox of staples by introducing a biocompatible Pd-mediated cysteine arylation.116
Another approach for selective cysteine modification in peptides and proteins utilises the thia-Michael addition.117 Zhou & co-workers introduced 5-methylene pyrrolone and its halo-derivatives as Michael acceptors which form cysteine conjugates at physiological pH (Scheme 4C). This bioconjugation strategy has been used for protein modification and disulfide replacement in the hormone somatostatin (Scheme 4C).118,119
Based on the Staudinger phosphonite reaction (SPhR), Hackenberger & co-workers recently introduced vinylphosphonite to cyclise peptides between azide and cysteine residues.120 They cyclised an azido containing BCL-9-derived peptide and investigated its stability and biophysical properties. At neutral to basic pH, the phosphonamidate was stable, helicity of the stapled peptide was successfully increased, and the stapled peptide inhibited the interaction between native BCL-9 and β-catenin. They further extended this strategy to labelling monoclonal antibodies and GFP.
Smith & co-workers developed a facile incorporation of s-tetrazine into peptides containing two unprotected cysteine residues.121 Peptides can be unstapled with UV light and the Diels–Alder reactivity of S,S-tetrazine provides a bioorthogonal handle for the installation of probes (Scheme 4D). Neumann & co-workers have recently reported a related selective tetrazine–thiol exchange (TeTEx) strategy for peptide macrocyclisation which is SPPS compatible and effective without any activation reagents.122 We reported a peptide staple inspired by the natural product lanthionine ketenamine.123 This reaction between 1,2-aminothiols and α-bromopyruvates is a combination of cysteine alkylation and imine formation and allows for the use of various aliphatic or PEG-based linkers (Scheme 4E).
Macrolactone cyclisation
An alternative to the formation of amide bonds is the creation of an ester bond, for example, between serine/threonine and the C-terminal carboxylate. Depsipeptides are natural products with such a characteristic bond in the peptidyl backbone. It has been suggested that replacing the –NH with an O distorts the α-helical and β-sheet structures by decreasing hydrogen bonding.124–126 Depsipeptides display antimicrobial,127,128 anti-tumour129 and antiviral activity.130 A prominent example is the antibiotic teixobactin (Scheme 5A).
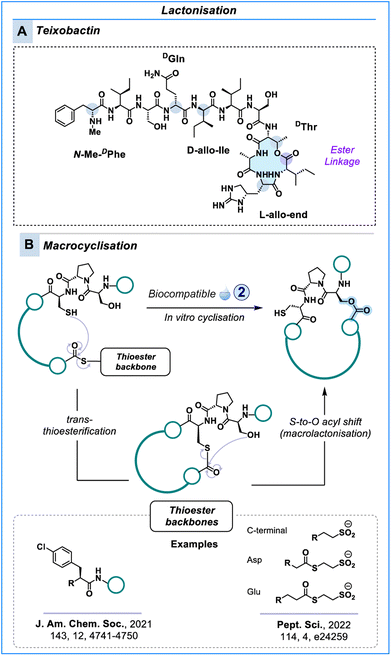 |
| Scheme 5 Macrolactone peptides. [A] Teixobactin is a natural antimicrobial depsipeptide with a macrolactone ring. [B] In vitro synthesis of macrolacton peptide libraries using S-to-O acyl shift. | |
Esterification does not spontaneously occur by mixing a carboxylic acid with an alcohol at neutral pH. Typically, activation of the carboxylate is necessary to allow for ester formation to occur in solution phase.131 Often this is difficult to perform during Fmoc-SPPS chemistry with many factors impacting acylation, making the synthesis of depsipeptides challenging tasks.132
Recently, Suga & co-workers introduced a biocompatible approach to prepare cyclic depsipeptides in line with their work to create macrocyclic libraries for RaPID (Scheme 5B).133,134 The strategy exploits the spontaneous S-to-O acyl shift in N-acetylated peptides comprising an N-terminal Ser/Thr–Pro–Cys–Gly motif and a C-terminal thioester. The linear peptide is subjected to buffer at pH 7.5 where it undergoes esterification via a two-step mechanism. An initial trans-thioesterification between the cysteine and thioester is followed by a rapid S-to-O acyl shift from the nucleophilic alcohol of Ser/Thr to establish an ester crosslink (Scheme 5B). Although, the methodology is compatible with peptides of diverse ring sizes and sequences, the Ser/Thr–Pro–Cys–Gly motif is pivotal for acyl transfer.
Serine/threonine ligation
A way to use serine or threonine to form backbone amide linkages instead of esters involves the serine/threonine ligation (STL), which involves the reaction between an N-terminal serine or threonine residue and a C-terminal salicylaldehyde ester (Scheme 6A).135 This technique has been predominantly used to couple two or more peptide fragments to chemically synthesise proteins.136 Li & co-workers have contributed significantly to developing this technique and could successfully synthesise several well-folded proteins, such as the ACYP enzyme,135 MUC1 glycopeptide antigen,136 IL-25
137 and nuclear protein HMGA1a.138 The ligation proceeds at rather mild conditions in pyridine–acetate buffer (1
:
1) without any metal additives. As a complementary strategy to native chemical ligation (NCL) discussed below, STL has certain advantages such as the relatively high abundance of serine and threonine in proteins (12.7%) compared to cysteine (1.5%), no need for N-terminal modification, and compatibility with most of the amino acids at the C-terminus.139
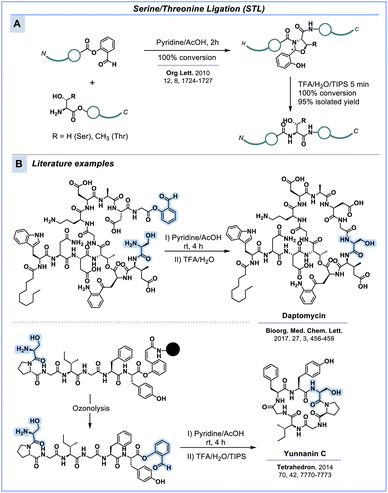 |
| Scheme 6 Serine/threonine ligation (STL) as an alternative to native chemical ligation (NCL) to [A] conjugate peptide fragments and [B] construct macrocyclic peptides. | |
Since STL leads to native amide linkage in the peptide, this strategy has also been explored in the total synthesis of peptide-based antibiotics like daptomycin,140,141 yunnanin C,142 (Scheme 5B) cyclomontanin B143 and their analogues. Liu & co-workers investigated the scope of STL for the synthesis of small and large macrocycles and observed minimal oligomerisation.144 STL is a two-step process in which a C-terminal aldehyde reacts with the α-amine of N-terminal serine or threonine to from an oxazolidine intermediate. Spontaneous O-to-N acyl transfer then forms an N,O-benzylidene acetal which upon acidolysis generates the amide linkage (Scheme 6A). The preferred pH range for the reaction is 4 to 6. Despite some rather harsh conditions, like the final acidolysis, there might be scope for further optimisation to make this strategy biocompatible.
Native chemical ligation
A related technique that uses N-terminal cysteine instead of serine/threonine to form amide bonds is native chemical ligation (NCL), which is broadly employed for protein synthesis but also useful for peptide macrocyclisation. While SPPS is widely used, there remain some technical drawbacks, such as diketopiperazine (DKP) and aspartimide formation, epimerisation of α-carbons and β-elimination of cysteine thiols due to repeated exposure to organic base.145,146 Another drawback is aggregation from inter-/intramolecular β-sheet formation during SPPS,147 which can lead to incomplete solvation of the resin-bound peptide and subsequently reduce coupling efficiency. Therefore, it is extremely challenging to efficiently construct peptides comprising more than 50 amino acids through standard SPPS, unless advanced flow chemistry is implemented as shown by Pentelute & co-workers.148
Addressing this challenge, Kent & co-workers149 devised NCL which involves a pair of peptide precursors, one fashioned with a C-terminal thioester and the other with the N-terminal unprotected cysteine. In NCL an initial trans-thioesterification takes place as the nucleophilic cysteine thiol undergoes an SN2 reaction with the corresponding carbonyl of the thioester fragment, establishing the new thioester crosslink. The proximity of the peptide permits the free amine to undergo an intramolecular rearrangement, which subsequently forms an amide bond and restores a thiol residue (Scheme 7A).149–153 Selenocysteine has proven an important addition in the synthesis of long polypeptides.154–157
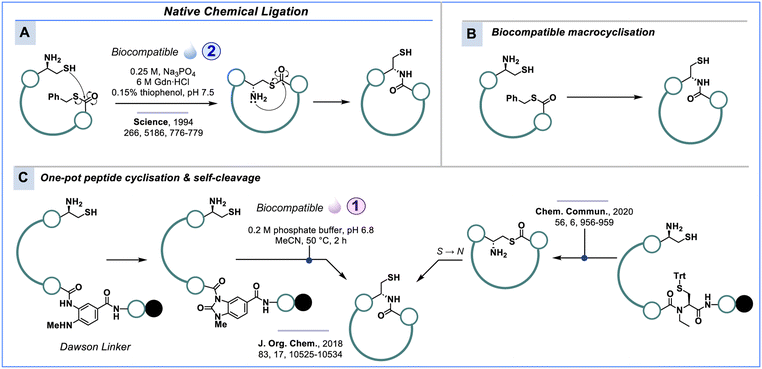 |
| Scheme 7 Native chemical ligation (NCL). [A] Fundamental N-to-S acyl shift mechanism. [B] Biocompatible NCL with an N-terminal aminothiol and C-terminal thioester. [C] Examples of NCL using safety catch linkers for direct on-resin C-terminal modification. | |
The introduction of NCL laid the foundations for constructing larger linear polypeptides linked via a native amide backbone, and has inspired researchers like Tulla-Puche and Barany,158 Olsen & co-workers,159 Stockdill & co-workers,160 and Hojo & co-workers161 to adopt the strategy to generate peptide macrocycles with a native amide crosslink (Scheme 7B).
Gless and Olsen generated a peptide macrocycle in aqueous solution using the Dawson linker (MeNbz).159,162 After transformation to the urea intermediate, the activated C-terminus reacts with N-terminal cysteine at 50 °C and pH 6.8 to release the cyclic peptide from the resin (Scheme 7C). Surrogate linker systems have also been explored by Hojo & co-workers.161 The N-ethylcysteine linker forms a thioester bond via N-to-S migration to enable intramolecular NCL (Scheme 7C).
Thiazoline and thiazole formation
Another method that relies on the unique properties of N-terminal cysteine is the nitrile-aminothiol click reaction used to form thiazolines.163 Oxidation of the thiazoline leads to thiazole, which is a small heterocycle that appears in a variety of bioactive compounds, including peptides.164 The unique architecture of the thiazole ring in azole-based peptides makes it an attractive motif in drug discovery.165 The thiazole unit in the peptide backbone can influence the folding architecture of peptides.166–169 Flow-on effects from the small thiazole graft can stabilise the 2D structure by improving the intramolecular H-bond networks,164,170 as well as increasing cell membrane permeability by reducing the number of H-bond donors and minimise water solvation of amide bonds.171 In nature, thiazole crosslinks are formed through a post-translational transformation of cysteine via the mechanism shown in Scheme 8A.172,173 While no biocompatible synthetic methods for thiazole formation have been reported yet, several peptides containing thiazoles have been synthesised using building blocks with the pre-installed thiazole motif (Scheme 8B), for example, dolastatin 3,174,175 cyclodidemnamide B,176 didmolamides A and B,177 obyanamide,178 scleritodermin A,179,180 aeruginazole A,181 sanguinamide A,182 marthiapeptide A183 and venturamides A and B.184
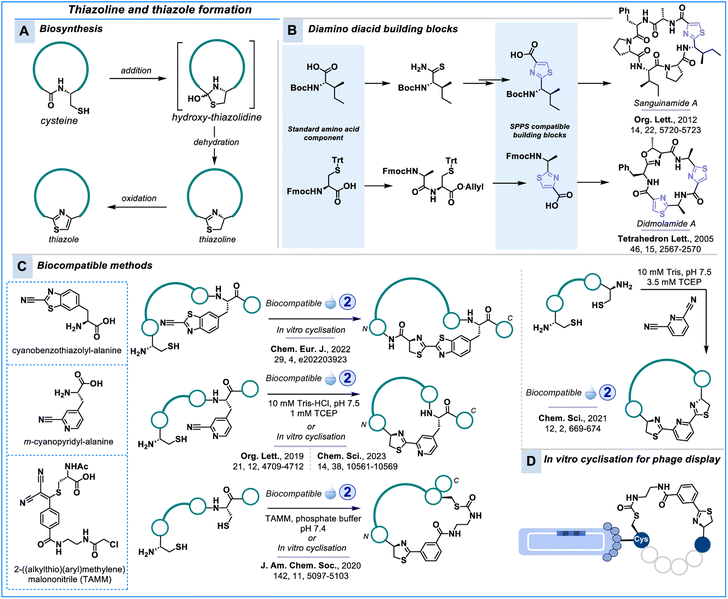 |
| Scheme 8 Thiazole and thiazoline peptide macrocyclisation. [A] Biochemical transformation of cysteine via a stepwise addition, dehydration and oxidation to the thiazole ring. [B] Thiazole-based peptides prepared from diamino diacid building blocks. [C] Biocompatible peptide macrocyclisation and stapling using the condensation reaction between 1,2-aminothiols and heteroaromatic nitriles or the malononitrile derivative TAMM. [D] Phage display peptide stapling with TAMM. | |
The thiazoline heterocycle is yet another amide bioisostere that is part of bioactive natural products and synthetic peptides.185–188 Inspired by the biosynthesis of firefly luciferin from 2-cyano-6-hydroxybenzothiazole and L-cysteine,189 the condensation reaction between electrophilic nitriles and 1,2-aminothiols has been exploited for biocompatible macrocyclisation of synthetic and genetically encoded peptide libraries alike (Scheme 8C).190–193
Cyanobenzothiazole (CBT) and m-cyanopyridine (Scheme 8C) are the two most commonly used moieties. The reaction of 2-cyanobenzothiazole and related nitriles with 1,2-aminothiol has been extensively explored in bioconjugation, enabling applications ranging from nanoparticle design to imaging techniques.194–203 Most recently, this reaction was employed in the in vitro translation of genetically encoded peptide libraries.204
The alternative m-cyanopyridyl-alanine (Cpa) can be used directly in SPPS to construct macrocyclic peptides,205 or the cynaopyridine motif can be constructed on a peptide side chain during SPPS.206 Cpa has recently also found application in genetically encoded libraries.207 Additionally, dicyanopyridine reagents can be employed for peptide stapling or to generate bicyclic peptides.37,208 Cpa can also be selectively incorporated into proteins.209
Wu, Tsai & co-workers reported that 2-((alkylthio)(aryl)methylene)malononitrile (TAMM) can also be specific for 1,2-aminothiols.210 Combination with a chloroacetamide for classic cysteine alkylation delivered a biocompatible handle to staple an N-terminal cysteine and a central cysteine residue which was also applied to phage display (Scheme 8D). Jongkees, Nitsche & co-workers recently reported a similar strategy where they combined the selective reactivity of N-terminal cysteine and non-N-terminal cysteine to construct bicyclic peptide libraries.211
Aldehyde and ketone modification
The lack of reactive carbonyl species in canonical amino acids renders aldehydes and ketones alternatives for selective peptide and protein modification. While oxime ligation has been extensively investigated for bioconjugation, it remains relatively underexplored in the context of peptide macrocyclisation, especially concerning genetically encoded peptide libraries. Oxime ligation involves the reaction between an aldehyde or ketone with a hydroxylamine to create a stable oxime bond (C
N–O) in E and Z isomers (Scheme 9A).212,213 Compared to general bioconjugation and peptide conjugation,214,215 examples of peptide stapling and macrocyclisation via oxime ligation are scarce in the literature.216–218 We have demonstrated a peptide cyclisation strategy utilising intramolecular oxime ligation between an alkoxyamine amino acid and an N-terminal α-ketoamide (Scheme 9A).219
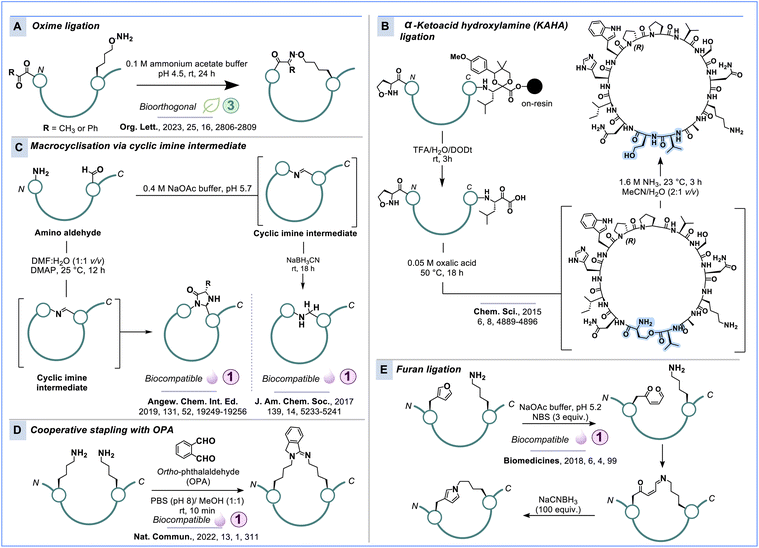 |
| Scheme 9 Peptide macrocyclisation techniques based on ketone and aldehyde reactivity. [A] Oxime ligation. [B] KAHA ligation. [C] Reductive amination and “CyClick”. [D] OPA stapling. [E] Ligation chemistry based on oxidation of furan to an aldehyde (keto–enal) and subsequent reduction to produce a pyrrole ring with lysine. | |
A related approach involving the use of α-ketoacids at the C-terminus and hydroxy- or alkoxyamines at the N-terminus is the α-ketoacid-hydroxylamine (KAHA) ligation, a method that has been extensively investigated by Bode & co-workers for the chemical synthesis of proteins (Scheme 9B).220 KAHA ligation offers the advantage of forming a stable amide bond without requiring any additives or coupling reagents. In the context of synthesising peptide macrocycles, Bode & co-workers described the in situ cyclisation of linear, unprotected peptides using KAHA ligation, employing (S)-5-oxaproline as the hydroxylamine moiety (Scheme 9B).221 Although KAHA ligation has not yet been integrated into display screening platforms, its ability to operate under mild aqueous conditions holds promise for biocompatible peptide macrocyclisation.
While imines formed through the reaction of aldehydes or ketones with amines are typically transient under biocompatible conditions, they can serve as crucial intermediates for the creation of more stable linkages. Baran, Malins & co-workers have harnessed this phenomenon to synthesise macrocyclic peptides through reductive amination, employing the biocompatible reducing agent sodium cyanoborohydride (Scheme 9C).222 If the imine intermediate is not trapped by hydride or other externally added nucleophiles, it can engage in a selective intramolecular macrocyclisation with the N-terminus, as reported by Raj & co-workers.223,224 This “CyClick” strategy forms a 4-imidazolidinone heterocycle from the N-terminal amine of a peptide and an aldehyde (Scheme 9C). Raj & co-workers have further expanded on the use of nitroalkane tethers for the site-specific stapling of aldehydes within peptide chains. This reaction occurs under physiological temperature and pH conditions and has been applied for late-stage peptide bioconjugation and cyclisation.225 Recently, the same group reported an arene triazene peptide macrocyclisation strategy which is rapid, reversible and biocompatible.226 Furthermore, this technique introduces an inherent chromophore at the cyclisation site, facilitating peptide detection by UV absorption.
Chen & co-workers have also explored peptide stapling through the utilisation of aldehydes and basic amino acid side chains. Initially, they devised a macrocyclisation method that involves lysine residues in an unprotected peptide, using an excess of formaldehyde with the assistance of nearby tyrosine or arginine residues in close proximity (referred to as KaY/KaR stapling).227 Although not totally biocompatible, the reaction proceeds under milder conditions and has excellent residue and position selectivity. Further progress towards biocompatible macrocyclisation by Chen & co-workers led to amine crosslinking in a peptide chain using o-phthalaldehyde (OPA).228 This method, an adaptation of the classic OPA–amine–thiol condensation, leverages either two lysine residues arranged side chain-to-side chain or one lysine residue in combination with an N-terminal amine (head-to-side chain) within a peptide chain. Both amines readily condense with OPA to generate an isoindolin-1-imine linker in aqueous buffer at pH 8 (Scheme 9D). Bell & Malins recently reported lysine stapling with 2,6-pyridinedialdehyde linkers and reductive amination.229
Furan-based side chains in peptides can undergo oxidation and ring-opening to generate aldehydes or ketones, which can subsequently be further modified, such as for macrocyclisation. Madder, Suga & co-workers developed an in vitro translation system for furylalanine, which can react with a lysine side chain to eventually form a pyrrole ring in the absence of competing nucleophiles (Scheme 9E).230 Recently, this strategy was further expanded by incorporating hydrazine or alkoxyamine-based amino acids in close proximity to the furan moiety.231
Ring closing metathesis
Like the example involving furylalanine, incorporating noncanonical amino acids offers an additional level of chemical orthogonality, enabling, for example, ring closing metathesis (RCM) and azide–alkyne cycloadditions between peptide side chains. Peptide macrocycles with all-hydrocarbon crosslinks accessed by RCM have emerged as useful surrogates of disulfide-bonds and for site-selective peptide stapling (Scheme 10). In pioneering studies, Miller and Grubbs232,233 installed a rigid substructure into a peptide framework and Clark and Ghadiri234 prepared β-sheet-like cylinders to explore supramolecular structures that exploit self-assembly of peptide aggregates. Yoshiya & co-workers235 utilised a water-soluble Ru-catalyst called AquaMet236 to transform this chemistry towards biocompatible conditions. RCM was performed by incubating unprotected peptides in a neutral buffer followed by the addition of AquaMet at 60 °C for 2 h. While 60 °C might prevent certain applications, this work certainly lays the foundation for biocompatible peptide macrocyclisation using RCM. Work towards olefinic ligation in protein models have been a research of interest of Davis & co-workers237–239 and have previously been reviewed.240,241
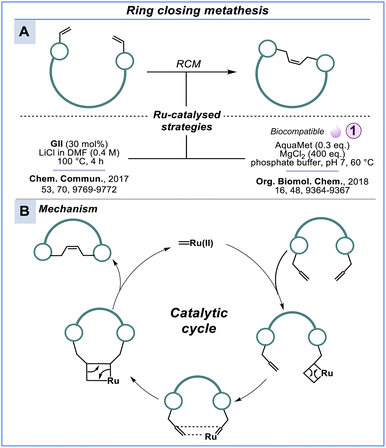 |
| Scheme 10 Ring closing metathesis. [A] All-hydrocarbon-crosslink strategies using ruthenium-based catalysis (Grubbs). [B] Simplified catalytic mechanism of a Ru-catalysed RCM reaction. | |
Azide–alkyne cycloaddition
The pioneering work of Meldal & co-workers242 and Sharpless & co-workers243 on the Cu-catalysed [3 + 2]-azide–alkyne cycloaddition (CuAAC) has evolved into an important synthetic strategy to link molecules via a [1,2,3]-triazole heterocycle. The triazole heterocycle itself has made significant contributions to a diverse array of bioactivities and has generated substantial interest as a distinctive bioisostere.244,245 Bertozzi & co-workers developed a copper-free, strain-promoted [3 + 2]-azide–alkyne cycloaddition (SPAAC), which has emerged as immensely important reaction in the field of bioconjugation, particularly involving living systems.246 Meldal, Sharpless and Bertozzi were jointly recognised for the development of ‘click chemistry and biorthogonal chemistry’ with The Nobel Prize in Chemistry in 2022 (Scheme 11).41,247–249
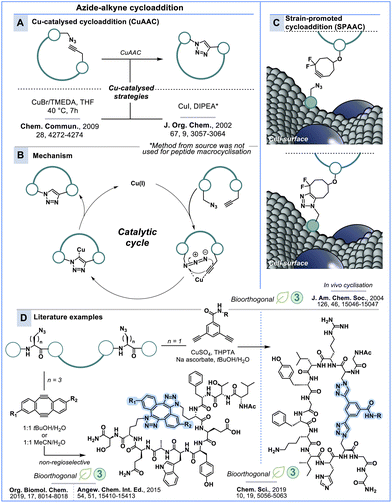 |
| Scheme 11 The azide–alkyne cycloaddition in peptide macrocyclisation and bioconjugation. [A] Cu-catalysed azide–alkyne cycloaddition (CuAAC) [B] The mechanism of CuAAC. [C] Strain-promoted azide–alkyne cycloaddition (SPAAC) on the cell surface. [D] Examples for CuAAC and SPAAC in biocompatible/bioorthogonal peptide stapling. | |
In its classic form, the 1,3-cycloaddition reaction involves a 1,3-dipole and a dipolarophile motif, where the dipolarophile and 1,3-dipole participate in a concerted pericyclic mechanism to form a five-membered ring via a [3 + 2]-cycloaddition.250 In CuAAC, an alkyne and azide serve as the dipolarophile and 1,3-dipole, respectively, catalysed by Cu(I) to selectively form the 1,4-substituted product heterocycle (Scheme 11B).242,243 While CuAAC is commonly used for conjugation, its reliance on copper makes it incompatible with living systems.251 Furthermore, copper can non-specifically bind to and precipitate proteins,252,253 hence, complicating protein conjugation and peptide modifications for screenings in the presence of drug targets. To address these challenges, Bertozzi & co-workers246 developed copper-free SPAAC inspired by the early works of Wittig and Krebs.254 They demonstrated that live cells displaying N-azidoacetyl sialic acid (SiaNAz) on the cell surface can be conjugated to a cyclooctyne–biotin probe (Scheme 11C). Further advancement of SPAAC employed difluorinated cyclooctyne (termed DIFO) to dramatically accelerate the reaction rate.255,256
CuAAc and SPAAC have been used extensively in macrocyclisation and stapling of flexible peptides to render them with defined bioactive conformations (Scheme 11D). Spring & co-workers have stabilised linear diazido peptides in defined α-helical conformations by reacting them with dialkynyl linkers in the presence of Cu-catalyst.257 This ‘double click’ stapling approach conferred improved biophysical and pharmacological properties of peptides. Further, they have employed the SPAAC reaction for the in situ generation of stapled p53-derived peptides binding to MDM2 in cell culture (Scheme 11D).36,258 The prototype double-SPAAC derived peptide displayed improved helicity, proteolytic stability and binding affinity. A follow-up study aimed at improving the water solubility of the staples by introducing permanent charges.259 These peptides displayed low nanomolar affinity and were also found to penetrate cells, thus highlighting the versatile utility of this bioorthogonal macrocyclisation chemistry.
Conclusion & future perspectives
Peptides, whether in their linear or macrocyclic forms, have evolved into promising pharmaceuticals. The discoveries of insulin, ACTH, salmon calcitonin, oxytocin and other constrained peptides ignited significant interest in macrocyclic peptide therapeutics during the early days of drug discovery. The successful translation of numerous peptide drugs into clinical use in recent decades has driven advances in peptide chemistry beyond natural amide and disulfide bond formation.
Researchers have directed their efforts towards alternative strategies for macrocyclisation and late-stage modification to enhance the pharmacokinetic properties of peptides. With the recent broad recognition of click chemistry and bioorthogonal chemistry, we anticipate a surge in research in the years to come, driving further advancements in biocompatible techniques for peptide macrocyclisation and expanding the toolkit of chemical methods for protein and cellular ligation.
With the rise of biological platforms employing mRNA, yeast surface and phage display, there is a growing demand for biocompatible chemistry to synthesise large libraries of cyclic peptides. Attaining mild, selective and orthogonal cyclisation on the nano- and picomolar concentration scale has become an essential requirement. Fortunately, significant progress has been made in the development of biocompatible ligation techniques which we have highlighted here.
In addition to the primarily chemical strategies discussed in this perspective, there has been significant interest in enzyme-mediated macrocyclisation,260,261 expressed protein ligation262,263 and SICLOPPS for peptide macrocyclisation within living cells.40,63 The field of chemoenzymatic ligation has experienced rapid advancements, notably with non-ribosomal peptide synthetases,264 asparaginyl endopeptidases265 and subtiligase derivatives,266 allowing for the cyclisation of various classes of peptides under physiological conditions. While the details of these biochemical methodologies are beyond the scope of this perspective, it is evident that they carry significant potential for broadening the horizons of biocompatible peptide macrocyclisation.
Data availability
The datasets supporting this article have been uploaded as part of the ESI (Table S1†).
Author contributions
J. H., P. G. and C. N. wrote the manuscript.
Conflicts of interest
There are no conflicts to declare.
Abbreviations
ACT | Activating group |
ACTH | Adrenocorticotropic hormone |
ACYP | Acylphosphatase-2 |
Ade | Adenine |
CAS | Chemical Abstracts Service |
CBT | 2-Cyanobenzothiazole |
CDI | Carbonyldiimidazole |
Cpa |
m-Cyanopyridyl-alanine |
CuAAC | Copper(I)-catalysed azide–alkyne cycloaddition |
DBMB | 2,4-Dibromo-5-methoxybenzaldehyde |
Dbz | Diaminobenzoic acid |
DEBPT | (3-(Diethoxyphosphoryloxy)-1,2,3-benzotriazin-4(3H)-one) |
DFS | Decafluoro-diphenylsulfone |
DIPEA |
N,N-Diisopropylethylamine |
DKP | Diketopiperazine |
DMF | Dimethyl formamide |
DPPA | Diphenyl phosphoryl azide |
fAr | Perfluoroarenes |
FDA | Food and Drug Administration |
GFP | Green fluorescent protein |
GII | Second generation Grubbs' catalyst |
HEPES |
N-2-Hydroxyethylpiperazine-N′-2-ethanesulfonic acid |
HATU | Hexafluorophosphate azabenzotriazole tetramethyl uronium |
HBTU | Hexafluorophosphate benzotriazole tetramethyl uronium |
HCTU | 2-(6-Chloro-1H-benzotriazole-1-yl)-1,1,3,3-tetramethylaminium hexafluorophosphate |
HMGA1a | High-mobility group protein |
HOBt | Hydroxybenzotriazole |
IL-25 | Interleukin 25 |
α-KAHA | Alpha-ketoacid-hydroxylamine |
KaY/KaR | Lysine–formaldehyde–tyrosine/lysine–formaldehyde–arginine |
MeNbz |
N-Methyl benzotriazole |
MOrPH-PhD | Macrocyclic organo-peptide hybrid phage display |
MUC1 | Mucin 1 |
NCL | Native chemical ligation |
NMP |
N-Methyl-2-pyrrolidone |
O2beY |
o-(2-Bromomethyl)tyrosine |
OPA |
o-Phthalaldehyde |
PEG | Polyethylene glycol |
RaPID | Random nonstandard peptide integrated discovery |
RCM | Ring closing metathesis |
RNA | Ribonucleic acid |
SiaNAz |
N-Azidoacetyl sialic acid |
SICLOPPS | Split-intein circular ligation of peptides and proteins |
SPAAC | Strain-promoted azide–alkyne cycloaddition |
SPhR | Staudinger phosphonite reaction |
SPPS | Solid phase peptide synthesis |
STL | Serine/threonine ligation |
TAMM | 2-((Alkylthio)(aryl)methylene)malononitrile |
TeTEx | Tetrazine–thiol exchange |
TCEP | Tris(2-carboxyethyl)phosphine |
Acknowledgements
We thank the Australian Research Council for funding support, including a Discovery Project (DP230100079) and Future Fellowship (FT220100010) awarded to CN.
References
- I. Vecchio, C. Tornali, N. L. Bragazzi and M. Martini, The discovery of insulin: an important milestone in the history of medicine, Front. Endocrinol., 2018, 9, 613 CrossRef.
- D. Fridmanis, A. Roga and J. Klovins, ACTH receptor (MC2R) specificity: what do we know about underlying molecular mechanisms?, Front. Endocrinol., 2017, 8, 13 CrossRef PubMed.
- F. R. Singer, Clinical efficacy of salmon calcitonin in Paget's disease of bone, Calcif. Tissue Int., 1991, 49, S7–S8 CrossRef PubMed.
- C. E. C. den Hertog, A. N. J. A. de Groot and P. W. J. van Dongen, History and use of oxytocics, Eur. J. Obstet. Gynecol. Reprod. Biol., 2001, 94, 8–12 CrossRef CAS.
- L. Bendicksen, A. S. Kesselheim and B. N. Rome, The vexing voyage of vasopressin: the consequences of granting market exclusivity to unapproved drugs, Chest, 2022, 162, 433–435 CrossRef PubMed.
- M. Pavel, F. Borson-Chazot, A. Cailleux, D. Hörsch, H. Lahner, R. Pivonello, L. Tauchmanova, C. Darstein, H. Olsson and F. Tiberg, Octreotide SC depot in patients with acromegaly and functioning neuroendocrine tumors: a phase 2, multicenter study, Cancer Chemother. Pharmacol., 2019, 83, 375–385 CrossRef CAS PubMed.
- O. Al Musaimi, D. Al Shaer, B. G. de la Torre and F. Albericio, 2017 FDA peptide harvest, Pharmaceuticals, 2018, 11, 42 CrossRef.
- D. Al Shaer, O. Al Musaimi, F. Albericio and B. G. de la Torre, 2018 FDA TIDES harvest, Pharmaceuticals, 2019, 12, 52 CrossRef CAS PubMed.
- D. Al Shaer, O. Al Musaimi, F. Albericio and B. G. de la Torre, 2019 FDA TIDES (peptides and oligonucleotides) harvest, Pharmaceuticals, 2020, 13, 40 CrossRef CAS.
- O. Al Musaimi, D. Al Shaer, F. Albericio and B. G. de la Torre, 2020 FDA TIDES (peptides and oligonucleotides) harvest, Pharmaceuticals, 2021, 14, 145 CrossRef.
- O. Al Musaimi, D. Al Shaer, F. Albericio and B. G. de la Torre, 2022 FDA TIDES (peptides and oligonucleotides) harvest, Pharmaceuticals, 2023, 16, 336 CrossRef PubMed.
- B. G. de la Torre and F. Albericio, The pharmaceutical industry in 2022: an analysis of FDA drug approvals from the perspective of molecules, Molecules, 2023, 28, 1038 CrossRef CAS PubMed.
- E. Marsault and M. L. Peterson, Macrocycles are great cycles: applications, opportunities, and challenges of synthetic macrocycles in drug discovery, J. Med. Chem., 2011, 54, 1961–2004 CrossRef CAS PubMed.
- C. Morrison, Constrained peptides' time to shine?, Nat. Rev. Drug Discovery, 2018, 17, 531–533 CrossRef CAS PubMed.
- N. Tsomaia, Peptide therapeutics: targeting the undruggable space, Eur. J. Med. Chem., 2015, 94, 459–470 CrossRef CAS PubMed.
- M. A. Abdalla and L. J. McGaw, Natural cyclic peptides as an attractive modality for therapeutics: a mini review, Molecules, 2018, 23, 2080 CrossRef.
- A. A. Vinogradov, Y. Yin and H. Suga, Macrocyclic peptides as drug candidates: recent progress and remaining challenges, J. Am. Chem. Soc., 2019, 141, 4167–4181 CrossRef CAS.
- T. A. Cardote and A. Ciulli, Cyclic and macrocyclic peptides as chemical tools to recognise protein surfaces and probe protein–protein interactions, ChemMedChem, 2016, 11, 787–794 CrossRef CAS PubMed.
- S. E. Northfield, C. K. Wang, C. I. Schroeder, T. Durek, M.-W. Kan, J. E. Swedberg and D. J. Craik, Disulfide-rich macrocyclic peptides as templates in drug design, Eur. J. Med. Chem., 2014, 77, 248–257 CrossRef CAS PubMed.
- D. J. Craik, Y. Young Shim, U. Göransson, G. P. Moss, N. Tan, P. D. Jadhav, J. Shen and M. J. Reaney, Nomenclature of homodetic cyclic peptides produced from ribosomal precursors: an IUPAC task group interim report, Pept. Sci., 2016, 106, 917–924 CrossRef CAS.
- A. T. Bockus, C. M. McEwen and R. S. Lokey, Form and function in cyclic peptide natural products: a pharmacokinetic perspective, Curr. Top. Med. Chem., 2013, 13, 821–836 CrossRef CAS.
- A. P. Zavascki, L. Z. Goldani, J. Li and R. L. Nation, Polymyxin B for the treatment of multidrug-resistant pathogens: a critical review, J. Antimicrob. Chemother., 2007, 60, 1206–1215 CrossRef CAS.
- J. A. Orwa, C. Govaerts, R. Busson, E. Roets, A. Van Schepdael and J. Hoogmartens, Isolation and structural characterization of polymyxin B components, J. Chromatogr. A, 2001, 912, 369–373 CrossRef CAS.
- R. Dahiya, S. Dahiya, N. K. Fuloria, S. Kumar, R. Mourya, S. V. Chennupati, S. Jankie, H. Gautam, S. Singh and S. K. Karan, Natural bioactive thiazole-based peptides from marine resources: structural and pharmacological aspects, Mar. Drugs, 2020, 18, 329 CrossRef CAS.
- R. Ribeiro, E. Pinto, C. Fernandes and E. Sousa, Marine cyclic peptides: antimicrobial activity and synthetic strategies, Mar. Drugs, 2022, 20, 397 CrossRef CAS.
- J. D. Hegemann, M. Zimmermann, X. Xie and M. A. Marahiel, Lasso peptides: an intriguing class of bacterial natural products, Acc. Chem. Res., 2015, 48, 1909–1919 CrossRef CAS.
- M. O. Maksimov, S. J. Pan and A. J. Link, Lasso peptides: structure, function, biosynthesis, and engineering, Nat. Prod. Rep., 2012, 29, 996–1006 RSC.
- O. McAuliffe, R. P. Ross and C. Hill, Lantibiotics: structure, biosynthesis and mode of action, FEMS Microbiol. Rev., 2001, 25, 285–308 CrossRef CAS.
- D. J. Craik, M.-H. Lee, F. B. Rehm, B. Tombling, B. Doffek and H. Peacock, Ribosomally-synthesised cyclic peptides from plants as drug leads and pharmaceutical scaffolds, Bioorg. Med. Chem., 2018, 26, 2727–2737 CrossRef CAS PubMed.
- X. Wang, M. Lin, D. Xu, D. Lai and L. Zhou, Structural diversity and biological activities of fungal cyclic peptides, excluding cyclodipeptides, Molecules, 2017, 22, 2069 CrossRef.
- K. De Smet and R. Contreras, Human antimicrobial peptides: defensins, cathelicidins and histatins, Biotechnol. Lett., 2005, 27, 1337–1347 CrossRef CAS PubMed.
- E. M. Kościuczuk, P. Lisowski, J. Jarczak, N. Strzałkowska, A. Jóźwik, J. Horbańczuk, J. Krzyżewski, L. Zwierzchowski and E. Bagnicka, Cathelicidins: family of antimicrobial peptides. A review, Mol. Biol. Rep., 2012, 39, 10957–10970 CrossRef PubMed.
- S. Ullrich and C. Nitsche, Bicyclic peptides: paving the road for therapeutics of the future, Pept. Sci., 2023 DOI:10.1002/pep2.24326.
- R. B. Merrifield, Solid phase peptide synthesis. I. The synthesis of a tetrapeptide, J. Am. Chem. Soc., 1963, 85, 2149–2154 CrossRef CAS.
- R. Merrifield, Solid-phase peptide synthesis. III. An improved synthesis of bradykinin, Biochemistry, 1964, 3, 1385–1390 CrossRef CAS.
- Y. H. Lau, Y. Wu, M. Rossmann, B. X. Tan, P. de Andrade, Y. S. Tan, C. Verma, G. J. McKenzie, A. R. Venkitaraman, M. Hyvönen and D. R. Spring, Double strain-promoted macrocyclization for the rapid selection of cell-active stapled peptides, Angew. Chem., Int. Ed., 2015, 54, 15410–15413 CrossRef CAS PubMed.
- R. Morewood and C. Nitsche, A biocompatible stapling reaction for in situ generation of constrained peptides, Chem. Sci., 2021, 12, 669–674 RSC.
- S. Voss, J. Rademann and C. Nitsche, Peptide–bismuth bicycles: in situ access to stable constrained peptides with superior bioactivity, Angew. Chem., Int. Ed., 2022, 134, e202113857 CrossRef.
- Y. Goto and H. Suga, The RaPID platform for the discovery of pseudo-natural macrocyclic peptides, Acc. Chem. Res., 2021, 54, 3604–3617 CrossRef CAS.
- C. Sohrabi, A. Foster and A. Tavassoli, Methods for generating and screening libraries of genetically encoded cyclic peptides in drug discovery, Nat. Rev. Chem, 2020, 4, 90–101 CrossRef CAS.
- E. M. Sletten and C. R. Bertozzi, Bioorthogonal chemistry: fishing for selectivity in a sea of functionality, Angew. Chem., Int. Ed., 2009, 48, 6974–6998 CrossRef CAS.
- P. Wu, The Nobel prize in chemistry 2022: fulfilling demanding applications with simple reactions, ACS Chem. Biol., 2022, 17, 2959–2961 CrossRef CAS PubMed.
- R. E. Bird, S. A. Lemmel, X. Yu and Q. A. Zhou, Bioorthogonal chemistry and its applications, Bioconjugate Chem., 2021, 32, 2457–2479 CrossRef CAS.
- D. Bauer, S. M. Sarrett, J. S. Lewis and B. M. Zeglis, Click chemistry: a transformative technology in nuclear medicine, Nat. Protoc., 2023, 18, 1659–1668 CrossRef CAS PubMed.
- A. Ben-Shem, N. Garreau de Loubresse, S. Melnikov, L. Jenner, G. Yusupova and M. Yusupov, The Structure of the eukaryotic ribosome at 3.0 Å resolution, Science, 2011, 334, 1524 CrossRef CAS.
- D. N. Wilson and J. H. Doudna Cate, The structure and function of the eukaryotic ribosome, Cold Spring Harbor Perspect. Biol., 2012, 4, a011536 Search PubMed.
- A. Prabhakar, J. Choi, J. Wang, A. Petrov and J. D. Puglisi, Dynamic basis of fidelity and speed in translation: coordinated multistep mechanisms of elongation and termination, Protein Sci., 2017, 26, 1352–1362 CrossRef CAS PubMed.
- K. J. McKnelly, W. Sokol and J. S. Nowick, Anaphylaxis induced by peptide coupling agents: lessons learned from repeated exposure to HATU, HBTU, and HCTU, J. Org. Chem., 2019, 85, 1764–1768 CrossRef.
- J. Miralles, J. Negro, J. Alonso, M. Garcia, F. Sanchez-Gascon and J. Soriano, Occupational rhinitis and bronchial asthma due to TBTU and HBTU sensitization, J. Invest. Allergol. Clin. Immunol., 2003, 13, 133–134 CAS.
- V. R. Pattabiraman and J. W. Bode, Rethinking amide bond synthesis, Nature, 2011, 480, 471–479 CrossRef CAS.
- K. G. Varnava and V. Sarojini, Making solid-phase peptide synthesis greener: a review of the literature, Chem.–Asian J., 2019, 14, 1088–1097 CrossRef CAS PubMed.
- J. N. Lambert, J. P. Mitchell and K. D. Roberts, The synthesis of cyclic peptides, J. Chem. Soc., Perkin Trans. 1, 2001, 471–484 RSC.
- J. S. Davies, The cyclization of peptides and depsipeptides, J. Pept. Sci., 2003, 9, 471–501 CrossRef CAS.
- C. J. White and A. K. Yudin, Contemporary strategies for peptide macrocyclization, Nat. Chem., 2011, 3, 509–524 CrossRef CAS.
- Y. H. Lau, P. De Andrade, Y. Wu and D. R. Spring, Peptide stapling techniques based on different macrocyclisation chemistries, Chem. Soc. Rev., 2015, 44, 91–102 RSC.
- R. Hirschmann, W. Yao, B. Arison, L. Maechler, A. Rosegay, P. A. Sprengeler and A. B. Smith III, The first synthesis of a tricyclic homodetic peptide employing coordinated orthogonal protection, Tetrahedron Lett., 1996, 37, 5637–5640 CrossRef CAS.
- J. Chatterjee, B. Laufer and H. Kessler, Synthesis of N-methylated cyclic peptides, Nat. Protoc., 2012, 7, 432–444 CrossRef CAS.
- H. Verma, B. Khatri, S. Chakraborti and J. Chatterjee, Increasing the bioactive space of peptide macrocycles by thioamide substitution, Chem. Sci., 2018, 9, 2443–2451 RSC.
- B. Ma, D. N. Litvinov, L. He, B. Banerjee and S. L. Castle, Total synthesis of celogentin C, Angew. Chem., Int. Ed., 2009, 48, 6104–6107 CrossRef CAS.
- A. Guzman-Martinez, R. Lamer and M. S. VanNieuwenhze, Total synthesis of lysobactin, J. Am. Chem. Soc., 2007, 129, 6017–6021 CrossRef CAS PubMed.
- J. B. Blanco-Canosa and P. E. Dawson, An efficient Fmoc-SPPS approach for the generation of thioester peptide precursors for use in native chemical ligation, Angew. Chem., Int. Ed., 2008, 120, 6957–6961 CrossRef.
- T. Ohara, M. Kaneda, T. Saito, N. Fujii, H. Ohno and S. Oishi, Head-to-tail macrocyclization of cysteine-free peptides using an o-aminoanilide linker, Bioorg. Med. Chem. Lett., 2018, 28, 1283–1286 CrossRef CAS PubMed.
- A. Tavassoli, SICLOPPS cyclic peptide libraries in drug discovery, Curr. Opin. Chem. Biol., 2017, 38, 30–35 CrossRef CAS PubMed.
- K. R. Lennard and A. Tavassoli, Peptides come round: using SICLOPPS libraries for early stage drug discovery, Chem.–Eur. J., 2014, 20, 10608–10614 CrossRef CAS.
- S. J. de Veer, M.-W. Kan and D. J. Craik, Cyclotides: from structure to function, Chem. Rev., 2019, 119, 12375–12421 CrossRef CAS.
- K. J. Rosengren, N. L. Daly, M. R. Plan, C. Waine and D. J. Craik, Twists, knots, and rings in proteins. Structural definition of the cyclotide framework, J. Biol. Chem., 2003, 278, 8606–8616 CrossRef CAS.
- D. Ziegler, Role of reversible oxidation-reduction of enzyme thiols-disulfides in metabolic regulation, Annu. Rev. Biochem., 1985, 54, 305–329 CrossRef CAS.
- B. Arunachalam, U. T. Phan, H. J. Geuze and P. Cresswell, Enzymatic reduction of disulfide bonds in lysosomes: characterization of a gamma-interferon-inducible lysosomal thiol reductase (GILT), Proc. Natl. Acad. Sci. U.S.A., 2000, 97, 745–750 CrossRef CAS.
- C. K. Wang and D. J. Craik, Designing macrocyclic disulfide-rich peptides for biotechnological applications, Nat. Chem. Biol., 2018, 14, 417–427 CrossRef CAS.
- P. W. Harris, S. H. Yang, A. Molina, G. López, M. Middleditch and M. A. Brimble, Plant antimicrobial peptides snakin-1 and snakin-2: chemical synthesis and insights into the disulfide connectivity, Chem.–Eur. J., 2014, 20, 5102–5110 CrossRef CAS.
- E. Calce, R. M. Vitale, A. Scaloni, P. Amodeo and S. De Luca, Air oxidation method employed for the disulfide bond formation of natural and synthetic peptides, Amino Acids, 2015, 47, 1507–1515 CrossRef CAS PubMed.
- O. A. Shepperson, C. C. Hanna, M. A. Brimble, P. W. R. Harris and A. J. Cameron, Total synthesis of novel antimicrobial β-hairpin capitellacin via rapid flow-based SPPS assembly and regioselective on-resin disulfide cyclisation, Int. J. Pept. Res. Ther., 2021, 28, 32 CrossRef.
- A. Chowdhury, V. Gour, B. K. Das, S. Chatterjee and A. Bandyopadhyay, Rapid and highly productive assembly of a disulfide bond in solid-phase peptide macrocyclization, Org. Lett., 2023, 25, 1280–1284 CrossRef CAS.
- S. Dissanayake, J. He, S. H. Yang, M. A. Brimble, P. W. Harris and A. J. Cameron, Flow-based Fmoc-SPPS preparation and SAR study of cathelicidin-PY reveals selective antimicrobial activity, Molecules, 2023, 28, 1993 CrossRef CAS.
- F. Oldach, R. Al Toma, A. Kuthning, T. Caetano, S. Mendo, N. Budisa and R. D. Süssmuth, Congeneric lantibiotics from ribosomal in vivo peptide synthesis with noncanonical amino acids, Angew. Chem., Int. Ed., 2012, 51, 415–418 CrossRef CAS PubMed.
- W. Liu, A. S. Chan, H. Liu, S. A. Cochrane and J. C. Vederas, Solid supported chemical syntheses of both components of the lantibiotic lacticin 3147, J. Am. Chem. Soc., 2011, 133, 14216–14219 CrossRef CAS PubMed.
- V. R. Pattabiraman, S. M. McKinnie and J. C. Vederas, Solid-supported synthesis and biological evaluation of the lantibiotic peptide bis (desmethyl) lacticin 3147 A2, Angew. Chem., Int. Ed., 2008, 47, 9472–9475 CrossRef CAS.
- S. M. McKinnie, A. C. Ross, M. J. Little and J. C. Vederas, The solid phase supported peptide synthesis of analogues of the lantibiotic lactocin S, MedChemComm, 2012, 3, 971–975 RSC.
- M. A. McLafferty, R. B. Kent, R. C. Ladner and W. Markland, M13 bacteriophage displaying disulfide-constrained microproteins, Gene, 1993, 128, 29–36 CrossRef CAS PubMed.
- S. Lu, S. Fan, S. Xiao, J. Li, S. Zhang, Y. Wu, C. Kong, J. Zhuang, H. Liu, Y. Zhao and C. Wu, Disulfide-directed multicyclic peptide libraries for the discovery of peptide ligands and drugs, J. Am. Chem. Soc., 2023, 145, 1964–1972 CrossRef CAS PubMed.
- T. L. Grove, P. M. Himes, S. Hwang, H. Yumerefendi, J. B. Bonanno, B. Kuhlman, S. C. Almo and A. A. Bowers, Structural insights into thioether bond formation in the biosynthesis of sactipeptides, J. Am. Chem. Soc., 2017, 139, 11734–11744 CrossRef CAS PubMed.
- D. Field, C. Hill, P. D. Cotter and R. P. Ross, The dawning of a ‘golden era’ in lantibiotic bioengineering, Mol. Microbiol., 2010, 78, 1077–1087 CrossRef CAS.
- C. T. Lohans and J. C. Vederas, Structural characterization of thioether-bridged bacteriocins, J. Antibiot., 2014, 67, 23–30 CrossRef CAS PubMed.
- W. Liu, A. S. H. Chan, H. Liu, S. A. Cochrane and J. C. Vederas, Solid supported chemical syntheses of both components of the lantibiotic lacticin 3147, J. Am. Chem. Soc., 2011, 133, 14216–14219 CrossRef CAS PubMed.
- A. C. Ross, H. Liu, V. R. Pattabiraman and J. C. Vederas, Synthesis of the lantibiotic lactocin S using peptide cyclizations on solid phase, J. Am. Chem. Soc., 2010, 132, 462–463 CrossRef CAS.
- R. S. Narayan and M. S. VanNieuwenhze, Versatile and stereoselective syntheses of orthogonally protected β-methylcysteine and β-methyllanthionine, Org. Lett., 2005, 7, 2655–2658 CrossRef CAS PubMed.
- S. Bregant and A. B. Tabor, Orthogonally protected lanthionines: synthesis and use for the solid-phase synthesis of an analogue of nisin ring C, J. Org. Chem., 2005, 70, 2430–2438 CrossRef CAS.
- K. J. Hetrick, M. C. Walker and W. A. van der Donk, Development and application of yeast and phage display of diverse lanthipeptides, ACS Cent. Sci., 2018, 4, 458–467 CrossRef CAS.
- J. H. Urban, M. A. Moosmeier, T. Aumüller, M. Thein, T. Bosma, R. Rink, K. Groth, M. Zulley, K. Siegers, K. Tissot, G. N. Moll and J. Prassler, Phage display and selection of lanthipeptides on the carboxy-terminus of the gene-3 minor coat protein, Nat. Commun., 2017, 8, 1500 CrossRef.
- F. M. Brunel and P. E. Dawson, Synthesis of constrained helical peptides by thioether ligation: application to analogs of gp41, Chem. Commun., 2005, 2552–2554 RSC.
- Y. Goto, A. Ohta, Y. Sako, Y. Yamagishi, H. Murakami and H. Suga, Reprogramming the translation initiation for the synthesis of physiologically stable cyclic peptides, ACS Chem. Biol., 2008, 3, 120–129 CrossRef CAS.
- Y. Goto, T. Katoh and H. Suga, Flexizymes for genetic code reprogramming, Nat. Protoc., 2011, 6, 779 CrossRef CAS.
- H. Saito, D. Kourouklis and H. Suga, An in vitro evolved precursor tRNA with aminoacylation activity, EMBO J., 2001, 20, 1797–1806 CrossRef CAS PubMed.
- T. Passioura and H. Suga, A RaPID way to discover nonstandard macrocyclic peptide modulators of drug targets, Chem. Commun., 2017, 53, 1931–1940 RSC.
- C. Nitsche, T. Passioura, P. Varava, M. C. Mahawaththa, M. M. Leuthold, C. D. Klein, H. Suga and G. Otting,
De Novo discovery of nonstandard macrocyclic peptides as noncompetitive inhibitors of the Zika virus NS2B-NS3 protease, ACS Med.
Chem. Lett., 2019, 10, 168–174 CrossRef CAS PubMed.
- J. Johansen-Leete, S. Ullrich, S. E. Fry, R. Frkic, M. J. Bedding, A. Aggarwal, A. S. Ashhurst, K. B. Ekanayake, M. C. Mahawaththa and V. M. Sasi, Antiviral cyclic peptides targeting the main protease of SARS-CoV-2, Chem. Sci., 2022, 13, 3826–3836 RSC.
- J. Johansen-Leete, T. Passioura, S. R. Foster, R. P. Bhusal, D. J. Ford, M. Liu, S. A. Jongkees, H. Suga, M. J. Stone and R. J. Payne, Discovery of potent cyclic sulfopeptide chemokine inhibitors via reprogrammed genetic code mRNA display, J. Am. Chem. Soc., 2020, 142, 9141–9146 CrossRef CAS PubMed.
- A. A. Vinogradov, Y. Zhang, K. Hamada, J. S. Chang, C. Okada, H. Nishimura, N. Terasaka, Y. Goto, K. Ogata and T. Sengoku,
De novo discovery of thiopeptide pseudo-natural products acting as potent and selective TNIK kinase inhibitors, J. Am. Chem. Soc., 2022, 144, 20332–20341 CrossRef CAS PubMed.
- A. E. Owens, J. A. Iannuzzelli, Y. Gu and R. Fasan, MOrPH-PhD: an integrated phage display platform for the discovery of functional genetically encoded peptide macrocycles, ACS Cent. Sci., 2020, 6, 368–381 CrossRef CAS PubMed.
- A. D. de Araujo, M. Mobli, G. F. King and P. F. Alewood, Cyclization of peptides by using selenolanthionine bridges, Angew. Chem., Int. Ed., 2012, 51, 10298–10302 CrossRef PubMed.
- L. Peraro, T. Siegert and J. Kritzer, Conformational restriction of peptides using dithiol bis-alkylation, Methods Enzymol., 2016, 580, 303–332 CAS.
- D. P. Fairlie and A. Dantas de Araujo, Stapling peptides using cysteine crosslinking, Pept. Sci., 2016, 106, 843–852 CrossRef CAS.
- R. Kowalczyk, P. W. Harris, M. A. Brimble, K. E. Callon, M. Watson and J. Cornish, Synthesis and evaluation of disulfide bond mimetics of amylin-(1–8) as agents to treat osteoporosis, Bioorg. Med. Chem., 2012, 20, 2661–2668 CrossRef CAS.
- S. Kalhor-Monfared, M. R. Jafari, J. T. Patterson, P. I. Kitov, J. J. Dwyer, J. M. Nuss and R. Derda, Rapid biocompatible macrocyclization of peptides with decafluoro-diphenylsulfone, Chem. Sci., 2016, 7, 3785–3790 RSC.
- A. M. Gold, Sulfhydryl groups of rabbit muscle glycogen phosphorylase b. Reaction with dinitrophenylating agents, Biochemistry, 1968, 7, 2106–2115 CrossRef CAS.
- A. M. Spokoyny, Y. Zou, J. J. Ling, H. Yu, Y.-S. Lin and B. L. Pentelute, A perfluoroaryl-cysteine SNAr chemistry approach to unprotected peptide stapling, J. Am. Chem. Soc., 2013, 135, 5946–5949 CrossRef CAS PubMed.
- Y. Zou, A. M. Spokoyny, C. Zhang, M. D. Simon, H. Yu, Y.-S. Lin and B. L. Pentelute, Convergent diversity-oriented side-chain macrocyclization scan for unprotected polypeptides, Org. Biomol. Chem., 2014, 12, 566–573 RSC.
- P. Diderich, D. Bertoldo, P. Dessen, M. M. Khan, I. Pizzitola, W. Held, J. Huelsken and C. Heinis, Phage selection of chemically stabilized α-helical peptide ligands, ACS Chem. Biol., 2016, 11, 1422–1427 CrossRef CAS PubMed.
- Y. Wang and D. H. C. Chou, A thiol–ene coupling approach to native peptide stapling and macrocyclization, Angew. Chem., Int. Ed., 2015, 54, 10931–10934 CrossRef CAS PubMed.
- A. A. Aimetti, R. K. Shoemaker, C.-C. Lin and K. S. Anseth, On-resin peptide macrocyclization using thiol–ene click chemistry, Chem. Commun., 2010, 46, 4061–4063 RSC.
- C. E. Hoyle and C. N. Bowman, Thiol–ene click chemistry, Angew. Chem., Int. Ed., 2010, 49, 1540–1573 CrossRef CAS PubMed.
- Y. Wang, B. J. Bruno, S. Cornillie, J. M. Nogieira, D. Chen, T. E. Cheatham III, C. S. Lim and D. H.-C. Chou, Application of thiol–yne/thiol–ene reactions for peptide and protein macrocyclizations, Chem.–Eur. J., 2017, 23, 7087–7092 CrossRef CAS PubMed.
- C. Hoppmann, R. Kühne and M. Beyermann, Intramolecular bridges formed by photoswitchable click amino acids, Beilstein J. Org. Chem., 2012, 8, 884–889 CrossRef CAS PubMed.
- C. Hoppmann, P. Schmieder, N. Heinrich and M. Beyermann, Photoswitchable click amino acids: light control of conformation and bioactivity, ChemBioChem, 2011, 12, 2555–2559 CrossRef CAS PubMed.
- N. S. Robertson, S. J. Walsh, E. Fowler, M. Yoshida, S. M. Rowe, Y. Wu, H. F. Sore, J. S. Parker and D. R. Spring, Macrocyclisation and functionalisation of unprotected peptides via divinyltriazine cysteine stapling, Chem. Commun., 2019, 55, 9499–9502 RSC.
- A. J. Rojas, C. Zhang, E. V. Vinogradova, N. H. Buchwald, J. Reilly, B. L. Pentelute and S. L. Buchwald, Divergent unprotected peptide macrocyclisation by palladium-mediated cysteine arylation, Chem. Sci., 2017, 8, 4257–4263 RSC.
- M. Ahangarpour, I. Kavianinia and M. A. Brimble, Thia-Michael addition: the route to promising opportunities for fast and cysteine-specific modification, Org. Biomol. Chem., 2023, 21, 3057–3072 RSC.
- Y. Zhang, C. Zang, G. An, M. Shang, Z. Cui, G. Chen, Z. Xi and C. Zhou, Cysteine-specific protein multi-functionalization and disulfide bridging using 3-bromo-5-methylene pyrrolones, Nat. Commun., 2020, 11, 1015 CrossRef CAS PubMed.
- Y. Zhang, X. Zhou, Y. Xie, M. M. Greenberg, Z. Xi and C. Zhou, Thiol specific and tracelessly removable bioconjugation via Michael addition to 5-methylene pyrrolones, J. Am. Chem. Soc., 2017, 139, 6146–6151 CrossRef CAS PubMed.
- M.-A. Kasper, M. Glanz, A. Oder, P. Schmieder, J. P. von Kries and C. P. R. Hackenberger, Vinylphosphonites for Staudinger-induced chemoselective peptide cyclization and functionalization, Chem. Sci., 2019, 10, 6322–6329 RSC.
- S. P. Brown and A. B. Smith III, Peptide/protein stapling and unstapling: introduction of s-tetrazine, photochemical release, and regeneration of the peptide/protein, J. Am. Chem. Soc., 2015, 137, 4034–4037 CrossRef CAS PubMed.
- D. W. T. Geers, K. Gavriel and K. Neumann, Rapid, traceless and facile peptide cyclization enabled by tetrazine-thiol exchange, J. Pept. Sci., 2023, e3548 CrossRef PubMed.
- R. Morewood and C. Nitsche, Bioinspired peptide stapling generates stable enzyme inhibitors, Chem. Commun., 2022, 58, 10817–10820 RSC.
- J. A. Scheike, C. Baldauf, J. Spengler, F. Albericio, M. T. Pisabarro and B. Koksch, Amide-to-ester substitution in coiled coils: the effect of removing hydrogen bonds on protein structure, Angew. Chem., Int. Ed., 2007, 46, 7766–7769 CrossRef CAS PubMed.
- Y. Fu, J. Gao, J. Bieschke, M. A. Dendle and J. W. Kelly, Amide-to-E-olefin versus amide-to-ester backbone H-bond perturbations: Evaluating the O–O repulsion for extracting H-bond energies, J. Am. Chem. Soc., 2006, 128, 15948–15949 CrossRef CAS PubMed.
- S. Deechongkit, P. E. Dawson and J. W. Kelly, Toward assessing the position-dependent contributions of backbone hydrogen bonding to β-sheet folding thermodynamics employing amide-to-ester perturbations, J. Am. Chem. Soc., 2004, 126, 16762–16771 CrossRef CAS PubMed.
- K. Jin, I. H. Sam, K. H. L. Po, D. a. Lin, E. H. Ghazvini Zadeh, S. Chen, Y. Yuan and X. Li, Total synthesis of teixobactin, Nat. Commun., 2016, 7, 1–6 Search PubMed.
- A. M. Giltrap, L. J. Dowman, G. Nagalingam, J. L. Ochoa, R. G. Linington, W. J. Britton and R. J. Payne, Total synthesis of teixobactin, Org. Lett., 2016, 18, 2788–2791 CrossRef CAS.
- R. W. Sparidans, E. Stokvis, J. M. Jimeno, L. López-Lázaro, J. H. Schellens and J. H. Beijnen, Chemical and enzymatic stability of a cyclic depsipeptide, the novel, marine-derived, anti-cancer agent kahalalide F, Anticancer Drugs, 2001, 12, 575–582 CrossRef CAS PubMed.
- M. Bilayethossain, D. V. D. Helm, J. Antel, G. M. Sheldrick, A. J. Weinheimer and S. Sanduja, Crystal and molecular structure of didemnin A, an antiviral depsipeptide, Int. J. Pept. Protein Res., 1996, 47, 20–27 CrossRef.
- I. Avan, C. D. Hall and A. R. Katritzky, Peptidomimetics via modifications of amino acids and peptide bonds, Chem. Soc. Rev., 2014, 43, 3575–3594 RSC.
- D. Palpal-Latoc, M. A. Brimble, P. Harris and A. J. Horsfall, Factors influencing on-resin depsipeptide bond formation: case studies on daptomycin- and brevicidine-derived sequences, Org. Biomol. Chem., 2023, 21, 4052–4060 RSC.
- M. Nagano, Y. Huang, R. Obexer and H. Suga, One-pot in vitro ribosomal synthesis of macrocyclic depsipeptides, J. Am. Chem. Soc., 2021, 143, 4741–4750 CrossRef CAS.
- M. Nagano, Y. Huang, R. Obexer and H. Suga, Chemical peptide macrolactonization via intramolecular S-to-S-to-O acyl transfer, Pept. Sci., 2022, 114, e24259 CrossRef CAS.
- X. Li, H. Y. Lam, Y. Zhang and C. K. Chan, Salicylaldehyde ester-induced chemoselective peptide ligations: enabling generation of natural peptidic linkages at the serine/threonine sites, Org. Lett., 2010, 12, 1724–1727 CrossRef CAS.
- C. Xu, H. Y. Lam, Y. Zhang and X. Li, Convergent synthesis of MUC1 glycopeptides via serine ligation, Chem. Commun., 2013, 49, 6200–6202 RSC.
- C. L. Lee, H. Liu, C. T. T. Wong, H. Y. Chow and X. Li, Enabling N-to-C Ser/Thr ligation for convergent protein synthesis via combining chemical ligation approaches, J. Am. Chem. Soc., 2016, 138, 10477–10484 CrossRef CAS PubMed.
- T. Li, H. Liu and X. Li, Chemical synthesis of HMGA1a proteins with post-translational modifications via Ser/Thr ligation, Org. Lett., 2016, 18, 5944–5947 CrossRef CAS PubMed.
- H. Liu and X. Li, Serine/threonine ligation: origin, mechanistic aspects, and applications, Acc. Chem. Res., 2018, 51, 1643–1655 CrossRef CAS PubMed.
- Y. Zhang, C. Xu, H. Y. Lam, C. L. Lee and X. Li, Protein chemical synthesis by serine and threonine ligation, Proc. Natl. Acad. Sci. U.S.A., 2013, 110, 6657–6662 CrossRef CAS PubMed.
- D. a. Lin, H. Y. Lam, W. Han, N. Cotroneo, B. A. Pandya and X. Li, Structure-activity relationship of daptomycin analogues with substitution at (2S, 3R) 3-methyl glutamic acid position, Bioorg. Med. Chem. Lett., 2017, 27, 456–459 CrossRef CAS PubMed.
- C. T. T. Wong, H. Y. Lam and X. Li, Effective synthesis of cyclic peptide yunnanin C and analogues via Ser/Thr ligation (STL)-mediated peptide cyclization, Tetrahedron, 2014, 70, 7770–7773 CrossRef CAS.
- C. T. T. Wong, H. Y. Lam and X. Li, Effective synthesis of kynurenine-containing peptides via on-resin ozonolysis of tryptophan residues: synthesis of cyclomontanin B, Org. Biomol. Chem., 2013, 11, 7616–7620 RSC.
- J.-F. Zhao, X.-H. Zhang, Y.-J. Ding, Y.-S. Yang, X.-B. Bi and C.-F. Liu, Facile synthesis of peptidyl salicylaldehyde esters and its use in cyclic peptide synthesis, Org. Lett., 2013, 15, 5182–5185 CrossRef CAS.
- M. Amblard, J.-A. Fehrentz, J. Martinez and G. Subra, Methods and protocols of modern solid phase peptide synthesis, Mol. Biotechnol., 2006, 33, 239–254 CrossRef CAS PubMed.
- R. Behrendt, P. White and J. Offer, Advances in Fmoc solid-phase peptide synthesis, J. Pept. Sci., 2016, 22, 4–27 CrossRef CAS PubMed.
- E. T. Sletten, M. Nuno, D. Guthrie and P. H. Seeberger, Real-time monitoring of solid-phase peptide synthesis using a variable bed flow reactor, Chem. Commun., 2019, 55, 14598–14601 RSC.
- A. J. Mijalis, D. A. Thomas, M. D. Simon, A. Adamo, R. Beaumont, K. F. Jensen and B. L. Pentelute, A fully automated flow-based approach for accelerated peptide synthesis, Nat. Chem. Biol., 2017, 13, 464–466 CrossRef CAS PubMed.
- P. E. Dawson, T. W. Muir, I. Clark-Lewis and S. B. Kent, Synthesis of proteins by native chemical ligation, Science, 1994, 266, 776–779 CrossRef CAS PubMed.
- L. R. Malins and R. J. Payne, Recent extensions to native chemical ligation for the chemical synthesis of peptides and proteins, Curr. Opin. Chem. Biol., 2014, 22, 70–78 CrossRef CAS PubMed.
- A. C. Conibear, E. E. Watson, R. J. Payne and C. F. Becker, Native chemical ligation in protein synthesis and semi-synthesis, Chem. Soc. Rev., 2018, 47, 9046–9068 RSC.
- S. S. Kulkarni, J. Sayers, B. Premdjee and R. J. Payne, Rapid and efficient protein synthesis through expansion of the native chemical ligation concept, Nat. Rev. Chem, 2018, 2, 0122 CrossRef CAS.
- V. Agouridas, O. El Mahdi, V. Diemer, M. Cargoet, J.-C. M. Monbaliu and O. Melnyk, Native chemical ligation and extended methods: mechanisms, catalysis, scope, and limitations, Chem. Rev., 2019, 119, 7328–7443 CrossRef CAS PubMed.
- L. R. Malins, N. J. Mitchell and R. J. Payne, Peptide ligation chemistry at selenol amino acids, J. Pept. Sci., 2014, 20, 64–77 CrossRef CAS PubMed.
- N. J. Mitchell, L. R. Malins, X. Liu, R. E. Thompson, B. Chan, L. Radom and R. J. Payne, Rapid additive-free selenocystine–selenoester peptide ligation, J. Am. Chem. Soc., 2015, 137, 14011–14014 CrossRef CAS PubMed.
- L. R. Malins and R. J. Payne, Synthesis and utility of β-selenol-phenylalanine for native chemical ligation–deselenization chemistry, Org. Lett., 2012, 14, 3142–3145 CrossRef CAS PubMed.
- L. R. Malins, A. M. Giltrap, L. J. Dowman and R. J. Payne, Synthesis of β-thiol phenylalanine for applications in one-pot ligation–desulfurization chemistry, Org. Lett., 2015, 17, 2070–2073 CrossRef CAS PubMed.
- J. Tulla-Puche and G. Barany, On-resin native chemical ligation for cyclic peptide synthesis, J. Org. Chem., 2004, 69, 4101–4107 CrossRef CAS PubMed.
- B. H. Gless and C. A. Olsen, Direct peptide cyclization and one-pot modification using the MeDbz linker, J. Org. Chem., 2018, 83, 10525–10534 CrossRef CAS PubMed.
- C. A. Arbour, K. J. Belavek, R. Tariq, S. Mukherjee, J. K. Tom, A. Isidro-Llobet, M. E. Kopach and J. L. Stockdill, Bringing macrolactamization full circle: self-cleaving head-to-tail macrocyclization of unprotected peptides via mild N-acyl urea activation, J. Org. Chem., 2018, 84, 1035–1041 CrossRef.
- G. Serra, L. Posada and H. Hojo, On-resin synthesis of cyclic peptides via tandem N-to-S acyl migration and intramolecular thiol additive-free native chemical ligation, Chem. Commun., 2020, 56, 956–959 RSC.
- J. B. Blanco-Canosa, B. Nardone, F. Albericio and P. E. Dawson, Chemical protein synthesis using a second-generation N-acylurea linker for the preparation of peptide-thioester precursors, J. Am. Chem. Soc., 2015, 137, 7197–7209 CrossRef CAS PubMed.
- C. Nitsche, The cyanopyridine–aminothiol click reaction: expanding horizons in chemical biology, Synlett, 2023 DOI:10.1055/a-2214-7612.
- I. V. Smolyar, A. K. Yudin and V. G. Nenajdenko, Heteroaryl rings in peptide macrocycles, Chem. Rev., 2019, 119, 10032–10240 CrossRef CAS.
- M. F. Arshad, A. Alam, A. A. Alshammari, M. B. Alhazza, I. M. Alzimam, M. A. Alam, G. Mustafa, M. S. Ansari, A. M. Alotaibi and A. A. Alotaibi, Thiazole: a versatile standalone moiety contributing to the development of various drugs and biologically active agents, Molecules, 2022, 27, 3994 CrossRef CAS PubMed.
-
J. Y. Mak, W. Xu and D. P. Fairlie, Thiazoles in peptides and peptidomimetics, Peptidomimetics I, 2017, vol. 48, pp. 235–266 Search PubMed.
- G. D. Rose, P. J. Fleming, J. R. Banavar and A. Maritan, A backbone-based theory of protein folding, Proc. Natl. Acad. Sci. U.S.A., 2006, 103, 16623–16633 CrossRef CAS PubMed.
- R. Jwad, D. Weissberger and L. Hunter, Strategies for fine-tuning the conformations of cyclic peptides, Chem. Rev., 2020, 120, 9743–9789 CrossRef CAS PubMed.
- G. Abbenante, D. Fairlie, L. Gahan, G. Hanson, G. Pierens and A. van den Brenk, Conformational control by thiazole and oxazoline rings in cyclic octapeptides of marine origin. Novel macrocyclic chair and boat conformations, J. Am. Chem. Soc., 1996, 118, 10384–10388 CrossRef CAS.
- H. S. Soor, S. D. Appavoo and A. K. Yudin, Heterocycles: versatile control elements in bioactive macrocycles, Bioorg. Med. Chem., 2018, 26, 2774–2779 CrossRef CAS PubMed.
- D. S. Nielsen, H. N. Hoang, R. J. Lohman, T. A. Hill, A. J. Lucke, D. J. Craik, D. J. Edmonds, D. A. Griffith, C. J. Rotter and R. B. Ruggeri, Improving on nature: making a cyclic heptapeptide orally bioavailable, Angew. Chem., Int. Ed., 2014, 53, 12059–12063 CrossRef CAS PubMed.
- T. L. Schneider, B. Shen and C. T. Walsh, Oxidase domains in epothilone and bleomycin biosynthesis: thiazoline to thiazole oxidation during chain elongation, Biochemistry, 2003, 42, 9722–9730 CrossRef CAS PubMed.
- T. L. Schneider and C. T. Walsh, Portability of oxidase domains in nonribosomal peptide synthetase modules, Biochemistry, 2004, 43, 15946–15955 CrossRef CAS PubMed.
- G. R. Pettit, Y. Kamano, C. W. Holzapfel, W. J. Van Zyl, A. A. Tuinman, C. L. Herald, L. Baczynskyj and J. M. Schmidt, Antineoplastic agents. 150. The structure and synthesis of dolastatin 3, J. Am. Chem. Soc., 1987, 109, 7581–7582 CrossRef CAS.
- U. Schmidt, R. Utz, A. Lieberknecht, H. Griesser, B. Potzolli, J. Bahr, K. Wagner and P. Fischer, Amino acids and peptides; 59.1 synthesis of biologically active cyclopeptides; 9.2 synthesis of 16 isomers of dolastatin 3; 3 I. synthesis of the 2-(1-aminoalkyl)-thiazole-4-carboxylic acids, Synthesis, 1987, 1987, 233–236 CrossRef.
- A. Arrault, A. Witczak-Legrand, P. Gonzalez, N. Bontemps-Subielos and B. Banaigs, Structure and total synthesis of cyclodidemnamide B, a cycloheptapeptide from the ascidian Didemnum molle, Tetrahedron Lett., 2002, 43, 4041–4044 CrossRef CAS.
- S.-L. You and J. W. Kelly, Total synthesis of didmolamides A and B, Tetrahedron Lett., 2005, 46, 2567–2570 CrossRef CAS.
- W. Zhang, Z.-H. Ma, D. Mei, C.-X. Li, X.-L. Zhang and Y.-X. Li, Total synthesis and reassignment of stereochemistry of obyanamide, Tetrahedron, 2006, 62, 9966–9972 CrossRef CAS.
- S. Liu, Y.-M. Cui and F.-J. Nan, Total synthesis of the originally proposed and revised structures of scleritodermin A, Org. Lett., 2008, 10, 3765–3768 CrossRef CAS PubMed.
- D. Sellanes, E. Manta and G. Serra, Toward the total synthesis of scleritodermin A: preparation of the C1–N15 fragment, Tetrahedron Lett., 2007, 48, 1827–1830 CrossRef CAS PubMed.
- P. Bruno, S. Pena, X. Just-Baringo, F. Albericio and M. Álvarez, Total synthesis of aeruginazole A, Org. Lett., 2011, 13, 4648–4651 CrossRef CAS PubMed.
- D. S. Nielsen, H. N. Hoang, R.-J. Lohman, F. Diness and D. P. Fairlie, Total synthesis, structure, and oral absorption of a thiazole cyclic peptide, sanguinamide A, Org. Lett., 2012, 14, 5720–5723 CrossRef CAS PubMed.
- Y. Zhang, M. A. Islam and S. R. McAlpine, Synthesis of the natural product marthiapeptide A, Org. Lett., 2015, 17, 5149–5151 CrossRef CAS PubMed.
- Y. Liu, P. He, Y. Zhang, X. Zhang, J. Liu and Y. Du, One-pot enantiomeric synthesis of thiazole-containing amino acids: total synthesis of venturamides A and B, J. Org. Chem., 2018, 83, 3897–3905 CrossRef CAS PubMed.
- Z. Lu, M. K. Harper, C. D. Pond, L. R. Barrows, C. M. Ireland and R. M. Van Wagoner, Thiazoline peptides and a tris-phenethyl urea from Didemnum molle with anti-HIV activity, J. Nat. Prod., 2012, 75, 1436–1440 CrossRef CAS PubMed.
- E. H. White, F. McCapra and G. F. Field, The structure and synthesis of firefly luciferin, J. Am. Chem. Soc., 1963, 85, 337–343 CrossRef CAS.
- B. A. Johnson, H. Anker and F. L. Meleney, Bacitracin: a new antibiotic produced by a member of the B. subtilis group, Science, 1945, 102, 376–377 CrossRef CAS PubMed.
- Q.-Y. Chen, Y. Liu, W. Cai and H. Luesch, Improved total synthesis and biological evaluation of potent apratoxin S4 based anticancer agents with differential stability and further enhanced activity, J. Med. Chem., 2014, 57, 3011–3029 CrossRef CAS PubMed.
- K. Niwa, Y. Nakajima and Y. Ohmiya, Applications of luciferin biosynthesis: bioluminescence assays for L-cysteine and luciferase, Anal. Biochem., 2010, 396, 316–318 CrossRef CAS.
- A. Berteotti, F. Vacondio, A. Lodola, M. Bassi, C. Silva, M. Mor and A. Cavalli, Predicting the reactivity of nitrile-carrying compounds with cysteine: a combined computational and experimental study, ACS Med. Chem. Lett., 2014, 5, 501–505 CrossRef CAS PubMed.
- O. V. Maltsev, V. Walter, M. J. Brandl and L. Hintermann, Medium buffer effects on the condensation of l-cysteine and aryl nitriles to (R)-2-aryl-4,5-dihydrothiazole-4-carboxylic acids, Synthesis, 2013, 45, 2763–2767 CrossRef CAS.
- T. Toyama, T. Saitoh, Y. Takahashi, K. Oka, D. Citterio, K. Suzuki and S. Nishiyama, Click reaction based on the biosynthesis of firefly luciferin, Chem. Lett., 2017, 46, 753–755 CrossRef CAS.
- Y. Yuan and G. Liang, A biocompatible, highly efficient click reaction and its applications, Org. Biomol. Chem., 2014, 12, 865–871 RSC.
- H. Ren, F. Xiao, K. Zhan, Y.-P. Kim, H. Xie, Z. Xia and J. Rao, A biocompatible condensation reaction for the labeling of terminal cysteine residues on proteins, Angew. Chem., Int. Ed., 2009, 48, 9658–9662 CrossRef CAS PubMed.
- G. Liang, H. Ren and J. Rao, A biocompatible condensation reaction for controlled assembly of nanostructures in living cells, Nat. Chem., 2010, 2, 54–60 CrossRef CAS.
- Y. Deng, S. Liu, K. Mei, A.-M. Tang, C.-Y. Cao and G.-L. Liang, Multifunctional small molecule for controlled assembly of oligomeric nanoparticles and crosslinked polymers, Org. Biomol. Chem., 2011, 9, 6917–6919 RSC.
- D. Ye, G. Liang, M. L. Ma and J. Rao, Controlling intracellular macrocyclization for the imaging of protease activity, Angew. Chem., Int. Ed., 2011, 50, 2275–2279 CrossRef CAS PubMed.
- Y. Yuan, J. Zhang, M. Wang, B. Mei, Y. Guan and G. Liang, Detection of glutathione in vitro and in cells by the controlled self-assembly of nanorings, Anal. Chem., 2013, 85, 1280–1284 CrossRef CAS PubMed.
- S. Sinha, D. Ahire, S. Wagh, D. Mullick, R. Sistla, K. Selvakumar, J. C. Cortes, S. P. Putlur, S. Mandlekar and B. M. Johnson, Electrophilicity of pyridazine-3-carbonitrile, pyrimidine-2-carbonitrile, and pyridine-carbonitrile derivatives: a chemical model to describe the formation of thiazoline derivatives in human liver microsomes, Chem. Res. Toxicol., 2014, 27, 2052–2061 Search PubMed.
- D. Ye, P. Pandit, P. Kempen, J. Lin, L. Xiong, R. Sinclair, B. Rutt and J. Rao, Redox-triggered self-assembly of gadolinium-based MRI probes for sensing reducing environment, Bioconjugate Chem., 2014, 25, 1526–1536 CrossRef CAS PubMed.
- D. Ye, A. J. Shuhendler, L. Cui, L. Tong, S. S. Tee, G. Tikhomirov, D. W. Felsher and J. Rao, Bioorthogonal cyclization-mediated in situ self-assembly of small-molecule probes for imaging caspase activity in vivo, Nat. Chem., 2014, 6, 519–526 CrossRef CAS PubMed.
- Y. Wang, X. Hu, J. Weng, J. Li, Q. Fan, Y. Zhang and D. Ye, A photoacoustic probe for the imaging of tumor apoptosis by caspase-mediated macrocyclization and self-assembly, Angew. Chem., Int. Ed., 2019, 58, 4886–4890 CrossRef CAS PubMed.
- Y. Cheng, H. Peng, W. Chen, N. Ni, B. Ke, C. Dai and B. Wang, Rapid and specific post-synthesis modification of DNA through a biocompatible condensation of 1,2-aminothiols with 2-cyanobenzothiazole, Chem.–Eur. J., 2013, 19, 4036–4042 CrossRef CAS PubMed.
- M. Liu, R. Yoshisada, A. Amedi, A. J. P. Hopstaken, M. N. Pascha, C. A. M. de Haan, D. P. Geerke, D. A. Poole and S. A. K. Jongkees, An efficient, site-selective and spontaneous peptide macrocyclisation during in vitro translation, Chem.–Eur. J., 2023, 29, e202203923 CrossRef CAS PubMed.
- C. Nitsche, H. Onagi, J.-P. Quek, G. Otting, D. Luo and T. Huber, Biocompatible macrocyclization between cysteine and 2-cyanopyridine generates stable peptide inhibitors, Org. Lett., 2019, 21, 4709–4712 CrossRef CAS PubMed.
- N. A. Patil, J.-P. Quek, B. Schroeder, R. Morewood, J. r. Rademann, D. Luo and C. Nitsche, 2-Cyanoisonicotinamide conjugation: a facile approach to generate potent peptide inhibitors of the Zika virus protease, ACS Med. Chem. Lett., 2021, 12, 732–737 CrossRef CAS PubMed.
- S. E. Iskandar, J. M. Pelton, E. T. Wick, D. L. Bolhuis, A. S. Baldwin, M. J. Emanuele, N. G. Brown and A. A. Bowers, Enabling genetic code expansion and peptide macrocyclization in mRNA display via a promiscuous orthogonal aminoacyl-tRNA synthetase, J. Am. Chem. Soc., 2023, 145, 1512–1517 CrossRef CAS.
- S. Ullrich, J. George, A. E. Coram, R. Morewood and C. Nitsche, Biocompatible and selective generation of bicyclic peptides, Angew. Chem., Int. Ed., 2022, 61, e202208400 CrossRef CAS.
- E. H. Abdelkader, H. Qianzhu, J. George, R. L. Frkic, C. J. Jackson, C. Nitsche, G. Otting and T. Huber, Genetic encoding of cyanopyridylalanine for in-cell protein macrocyclization by the nitrile–aminothiol click reaction, Angew. Chem., Int. Ed., 2022, 61, e202114154 CrossRef CAS PubMed.
- X. Zheng, Z. Li, W. Gao, X. Meng, X. Li, L. Y. P. Luk, Y. Zhao, Y.-H. Tsai and C. Wu, Condensation of 2-((alkylthio)(aryl)methylene)malononitrile with 1,2-aminothiol as a novel bioorthogonal reaction for site-specific protein modification and peptide cyclization, J. Am. Chem. Soc., 2020, 142, 5097–5103 CrossRef CAS PubMed.
- M. Liu, R. Morewood, R. Yoshisada, M. N. Pascha, A. J. P. Hopstaken, E. Tarcoveanu, D. A. Poole, C. A. M. de Haan, C. Nitsche and S. A. K. Jongkees, Selective thiazoline peptide cyclisation compatible with mRNA display and efficient synthesis, Chem. Sci., 2023, 14, 10561–10569 RSC.
- S. Ulrich, D. Boturyn, A. Marra, O. Renaudet and P. Dumy, Oxime ligation: a chemoselective click-type reaction for accessing multifunctional biomolecular constructs, Chem.–Eur. J., 2014, 20, 34–41 CrossRef CAS PubMed.
- D. K. Kölmel and E. T. Kool, Oximes and hydrazones in bioconjugation: mechanism and catalysis, Chem. Rev., 2017, 117, 10358–10376 CrossRef.
- A. Hering, N. Braga Emidio and M. Muttenthaler, Expanding the versatility and scope of the oxime ligation: rapid bioconjugation to disulfide-rich peptides, Chem. Commun., 2022, 58, 9100–9103 RSC.
- S. Duflocq, J. Zhou, F. Huguenot, M. Vidal and W.-Q. Liu, One-pot oxime ligation from peptides bearing thiazolidine and aminooxyacetyl groups, RSC Adv., 2020, 10, 17681–17685 RSC.
- C. M. Haney, M. T. Loch and W. S. Horne, Promoting peptide α-helix formation with dynamic covalent oxime side-chain cross-links, Chem. Commun., 2011, 47, 10915–10917 RSC.
- D. E. Streefkerk, M. Schmidt, J. H. Ippel, T. M. Hackeng, T. Nuijens, P. Timmerman and J. H. van Maarseveen, Synthesis of constrained tetracyclic peptides by consecutive CEPS, CLIPS, and oxime ligation, Org. Lett., 2019, 21, 2095–2100 CrossRef CAS PubMed.
- K. D. Roberts, J. N. Lambert, N. J. Ede and A. M. Bray, Preparation of cyclic peptide libraries using intramolecular oxime formation, J. Pept. Sci., 2004, 10, 659–665 CrossRef CAS.
- L. J. Davies, L. M. Shuttleworth, X. Zhang, S. Peng and C. Nitsche, Bioorthogonal peptide macrocyclization using oxime ligation, Org. Lett., 2023, 25, 2806–2809 CrossRef CAS PubMed.
- J. W. Bode, R. M. Fox and K. D. Baucom, Chemoselective amide ligations by decarboxylative condensations of N-alkylhydroxylamines and α-ketoacids, Angew. Chem., Int. Ed., 2006, 45, 1248–1252 CrossRef CAS PubMed.
- F. Rohrbacher, G. Deniau, A. Luther and J. W. Bode, Spontaneous head-to-tail cyclization of unprotected linear peptides with the KAHA ligation, Chem. Sci., 2015, 6, 4889–4896 RSC.
- L. R. Malins, J. N. deGruyter, K. J. Robbins, P. M. Scola, M. D. Eastgate, M. R. Ghadiri and P. S. Baran, Peptide macrocyclization inspired by non-ribosomal imine natural products, J. Am. Chem. Soc., 2017, 139, 5233–5241 CrossRef CAS PubMed.
- V. Adebomi, R. D. Cohen, R. Wills, H. A. H. Chavers, G. E. Martin and M. Raj, CyClick chemistry for the synthesis of cyclic peptides, Angew. Chem., Int. Ed., 2019, 58, 19073–19080 CrossRef CAS PubMed.
- H. Shao, V. Adebomi, A. Bruce, M. Raj and K. N. Houk, Intramolecular hydrogen bonding enables a zwitterionic mechanism for macrocyclic peptide formation: computational mechanistic studies of CyClick chemistry, Angew. Chem., Int. Ed., 2023, 62, e202307210 CrossRef CAS.
- S. Mahesh, V. Adebomi, Z. P. Muneeswaran and M. Raj, Bioinspired nitroalkylation for selective protein modification and peptide stapling, Angew. Chem., Int. Ed., 2020, 59, 2793–2801 CrossRef CAS PubMed.
- O. Nwajiobi, A. K. Verma and M. Raj, Rapid arene triazene chemistry for macrocyclization, J. Am. Chem. Soc., 2022, 144, 4633–4641 CrossRef CAS PubMed.
- B. Li, H. Tang, A. Turlik, Z. Wan, X.-S. Xue, L. Li, X. Yang, J. Li, G. He, K. N. Houk and G. Chen, Cooperative stapling of native
peptides at lysine and tyrosine or arginine with formaldehyde, Angew. Chem., Int. Ed., 2021, 60, 6646–6652 CrossRef CAS.
- B. Li, L. Wang, X. Chen, X. Chu, H. Tang, J. Zhang, G. He, L. Li and G. Chen, Extendable stapling of unprotected peptides by crosslinking two amines with o-phthalaldehyde, Nat. Commun., 2022, 13, 311 CrossRef CAS PubMed.
- H. J. Bell and L. R. Malins, Peptide macrocyclisation via late-stage reductive amination, Org. Biomol. Chem., 2022, 20, 6250–6256 RSC.
- K. W. Decoene, W. Vannecke, T. Passioura, H. Suga and A. Madder, Pyrrole-mediated peptide cyclization identified through genetically reprogrammed peptide synthesis, Biomedicines, 2018, 6, 99 CrossRef CAS PubMed.
- A. Manicardi, A. Theppawong, M. Van Troys and A. Madder, Proximity-induced ligation and one-pot macrocyclization of 1,4-diketone-tagged peptides derived from 2,5-disubstituted furans upon release from the solid support, Org. Lett., 2023, 25, 6618–6622 CrossRef CAS PubMed.
- S. J. Miller and R. H. Grubbs, Synthesis of conformationally restricted amino acids and peptides employing olefin metathesis, J. Am. Chem. Soc., 1995, 117, 5855–5856 CrossRef CAS.
- S. J. Miller, H. E. Blackwell and R. H. Grubbs, Application of ring-closing metathesis to the synthesis of rigidified amino acids and peptides, J. Am. Chem. Soc., 1996, 118, 9606–9614 CrossRef CAS.
- T. D. Clark and M. R. Ghadiri, Supramolecular design by covalent capture. Design of a peptide cylinder via hydrogen-bond-promoted intermolecular olefin metathesis, J. Am. Chem. Soc., 1995, 117, 12364–12365 CrossRef CAS.
- S. Masuda, S. Tsuda and T. Yoshiya, Ring-closing metathesis of unprotected peptides in water, Org. Biomol. Chem., 2018, 16, 9364–9367 RSC.
- K. Skowerski, G. Szczepaniak, C. Wierzbicka, Ł. Gułajski, M. Bieniek and K. Grela, Highly active catalysts for olefin metathesis in water, Catal. Sci. Technol., 2012, 2, 2424–2427 RSC.
- Y. A. Lin, J. M. Chalker, N. Floyd, G. J. Bernardes and B. G. Davis, Allyl sulfides are privileged substrates in aqueous cross-metathesis: application to site-selective protein modification, J. Am. Chem. Soc., 2008, 130, 9642–9643 CrossRef CAS PubMed.
- J. M. Chalker, Y. A. Lin, O. Boutureira and B. G. Davis, Enabling olefin metathesis on proteins: chemical methods for installation of S-allyl cysteine, Chem. Commun., 2009, 3714–3716 RSC.
- Y. A. Lin, O. Boutureira, L. Lercher, B. Bhushan, R. S. Paton and B. G. Davis, Rapid cross-metathesis for reversible protein modifications via chemical access to Se-allyl-selenocysteine in proteins, J. Am. Chem. Soc., 2013, 135, 12156–12159 CrossRef CAS PubMed.
- Y. A. Lin, J. M. Chalker and B. G. Davis, Olefin metathesis for site-selective protein modification, ChemBioChem, 2009, 10, 959–969 CrossRef CAS.
- M. S. Messina and H. D. Maynard, Modification of proteins using olefin metathesis, Mater. Chem. Front., 2020, 4, 1040–1051 RSC.
- C. W. Tornøe, C. Christensen and M. Meldal, Peptidotriazoles on solid phase: [1,2,3]-triazoles by regiospecific copper(I)-catalyzed 1,3-dipolar cycloadditions of terminal alkynes to azides, J. Org. Chem., 2002, 67, 3057–3064 CrossRef PubMed.
- V. V. Rostovtsev, L. G. Green, V. V. Fokin and K. B. Sharpless, A stepwise Huisgen cycloaddition process: copper(I)-catalyzed regioselective “ligation” of azides and terminal alkynes, Angew. Chem., Int. Ed., 2002, 114, 2708–2711 CrossRef.
- E. Bonandi, M. S. Christodoulou, G. Fumagalli, D. Perdicchia, G. Rastelli and D. Passarella, The 1,2,3-triazole ring as a bioisostere in medicinal chemistry, Drug Discovery Today, 2017, 22, 1572–1581 CrossRef CAS PubMed.
- N. G. Aher, V. S. Pore, N. N. Mishra, A. Kumar, P. K. Shukla, A. Sharma and M. K. Bhat, Synthesis and antifungal activity of 1,2,3-triazole containing fluconazole analogues, Bioorg. Med. Chem. Lett., 2009, 19, 759–763 CrossRef CAS PubMed.
- N. J. Agard, J. A. Prescher and C. R. Bertozzi, A strain-promoted [3+2] azide–alkyne cycloaddition for covalent modification of biomolecules in living systems, J. Am. Chem. Soc., 2004, 126, 15046–15047 CrossRef CAS.
- M. Meldal and C. W. Tornøe, Cu-catalyzed azide–alkyne cycloaddition, Chem. Rev., 2008, 108, 2952–3015 CrossRef CAS PubMed.
- H. C. Kolb and K. B. Sharpless, The growing impact of click chemistry on drug discovery, Drug Discovery Today, 2003, 8, 1128–1137 CrossRef CAS PubMed.
- V. D. Bock, H. Hiemstra and J. H. Van Maarseveen, CuI-catalyzed alkyne–azide “click” cycloadditions from a mechanistic and synthetic perspective, Eur. J. Org Chem., 2006, 2006, 51–68 CrossRef.
- R. Huisgen, 1.3-Dipolare Cycloadditionen Rückschau und Ausblick, Angew. Chem., Int. Ed., 1963, 75, 604–637 CrossRef CAS.
- B. Ashish, K. Neeti and K. Himanshu, Copper toxicity: a comprehensive study, Res. J. Recent Sci., 2013, 2277, 2502 Search PubMed.
- E. H. Abdelkader, A. Feintuch, X. Yao, L. A. Adams, L. Aurelio, B. Graham, D. Goldfarb and G. Otting, Protein conformation by EPR spectroscopy using gadolinium tags clicked to genetically encoded p-azido-L-phenylalanine, Chem. Commun., 2015, 51, 15898–15901 RSC.
- C.-T. Loh, B. Graham, E. H. Abdelkader, K. L. Tuck and G. Otting, Generation of pseudocontact shifts in proteins with lanthanides using small “Clickable” nitrilotriacetic acid and iminodiacetic acid tags, Chem.–Eur. J., 2015, 21, 5084–5092 CrossRef CAS PubMed.
- G. Wittig and A. Krebs, Zur Existenz niedergliedriger Cycloalkine, I, Chem. Ber., 1961, 94, 3260–3275 CrossRef CAS.
- J. A. Codelli, J. M. Baskin, N. J. Agard and C. R. Bertozzi, Second-generation difluorinated cyclooctynes for copper-free click chemistry, J. Am. Chem. Soc., 2008, 130, 11486–11493 CrossRef CAS.
- S. T. Laughlin, J. M. Baskin, S. L. Amacher and C. R. Bertozzi,
In vivo imaging of membrane-associated glycans in developing zebrafish, Science, 2008, 320, 664–667 CrossRef CAS.
- Y. H. Lau, Y. Wu, P. de Andrade, W. R. J. D. Galloway and D. R. Spring, A two-component 'double-click' approach to peptide stapling, Nat. Protoc., 2015, 10, 585–594 CrossRef CAS PubMed.
- K. Sharma, A. V. Strizhak, E. Fowler, W. Xu, B. Chappell, H. F. Sore, W. R. J. D. Galloway, M. N. Grayson, Y. H. Lau, L. S. Itzhaki and D. R. Spring, Functionalized double strain-promoted stapled peptides for inhibiting the p53-MDM2 interaction, ACS Omega, 2020, 5, 1157–1169 CrossRef CAS PubMed.
- K. Sharma, A. V. Strizhak, E. Fowler, X. Wang, W. Xu, C. Hatt Jensen, Y. Wu, H. F. Sore, Y. H. Lau, M. Hyvönen, L. S. Itzhaki and D. R. Spring, Water-soluble, stable and azide-reactive strained dialkynes for biocompatible double strain-promoted click chemistry, Org. Biomol. Chem., 2019, 17, 8014–8018 RSC.
- I. Saska, A. D. Gillon, N. Hatsugai, R. G. Dietzgen, I. Hara-Nishimura, M. A. Anderson and D. J. Craik, An asparaginyl endopeptidase mediates in vivo protein backbone cyclization, J. Biol. Chem., 2007, 282, 29721–29728 CrossRef CAS PubMed.
- F. B. Rehm, T. J. Tyler, S. J. de Veer, D. J. Craik and T. Durek, Enzymatic C-to-C protein ligation, Angew. Chem., Int. Ed., 2022, 134, e202116672 CrossRef.
- T. W. Muir, D. Sondhi and P. A. Cole, Expressed protein ligation: a general method for protein engineering, Proc. Natl. Acad. Sci. U. S. A., 1998, 95, 6705–6710 CrossRef CAS PubMed.
- H. Iwai and A. Plückthun, Circular β-lactamase: stability enhancement by cyclizing the backbone, FEBS Lett., 1999, 459, 166–172 CrossRef CAS.
- L. Zhuang, S. Huang, W.-Q. Liu, A. S. Karim, M. C. Jewett and J. Li, Total in vitro biosynthesis of the nonribosomal macrolactone peptide valinomycin, Metab. Eng., 2020, 60, 37–44 CrossRef CAS PubMed.
- G. K. T. Nguyen, S. Wang, Y. Qiu, X. Hemu, Y. Lian and J. P. Tam, Butelase 1 is an Asx-specific ligase enabling peptide macrocyclization and synthesis, Nat. Chem. Biol., 2014, 10, 732–738 CrossRef CAS PubMed.
- A. Toplak, T. Nuijens, P. J. L. M. Quaedflieg, B. Wu and D. B. Janssen, Peptiligase, an enzyme for efficient chemoenzymatic peptide synthesis and cyclization in water, Adv. Synth. Catal., 2016, 358, 2140–2147 CrossRef CAS.
Footnote |
† These authors contributed equally. |
|
This journal is © The Royal Society of Chemistry 2024 |
Click here to see how this site uses Cookies. View our privacy policy here.