DOI:
10.1039/D3SC05796H
(Edge Article)
Chem. Sci., 2024,
15, 3365-3371
Solid-state mechanochemistry for the rapid and efficient synthesis of tris-cyclometalated iridium(III) complexes†
Received
31st October 2023
, Accepted 18th January 2024
First published on 21st January 2024
Abstract
Tris-cyclometalated iridium(III) complexes have received widespread attention as attractive prospective materials for e.g., organic light-emitting diodes (OLEDs), photoredox catalysts, and bioimaging probes. However, their preparation usually requires prolonged reaction times, significant amounts of high-boiling solvents, multistep synthesis, and inert-gas-line techniques. Unfortunately, these requirements represent major drawbacks from both a production-cost and an environmental perspective. Herein, we show that a two-step mechanochemical protocol using ball milling enables the rapid and efficient synthesis of various tris-cyclometalated iridium(III) complexes from relatively cheap iridium(III) chloride hydrate without the use of significant amounts of organic solvent in air. Notably, a direct one-pot procedure is also demonstrated. The present solid-state approach can be expected to inspire the development of cost-effective and timely production methods for these valuable iridium-based complexes, as well as the discovery of new phosphorescent materials, sensors, and catalysts.
Introduction
Tris-cyclometalated iridium(III) complexes such as fac-Ir(ppy)3 have been widely employed as phosphorescent dopants in electroluminescent organic light-emitting diodes (OLEDs), bioimaging probes, sensors, and photoredox catalysts (Fig. 1a).1–5 The development of efficient, low-cost, time-saving, and environmentally friendly synthetic pathways to these complexes has thus attracted considerable attention in a broad range of scientific research fields. To date, a variety of useful procedures for their preparation has already been reported.1,6–8 Among these, a two-step procedure that involves the reaction of iridium(III) chloride hydrate (1) with the pyridine-based ligand (2) to give the chloride-bridged dimer (3), followed by silver-triflate-mediated ligand exchange to give the desired complex 4, has been widely used as one of the most general and practical methods (Fig. 1b).1,6–8 The advantages of this protocol include the facts that the overall yield of 4 is often higher than that in other methods and iridium(III) chloride hydrate is a relatively cheap starting material compared to other iridium-based precursors, such as iridium(III) acetylacetonate.1,2,6–8 However, this established route requires significant amounts of high-boiling organic solvents, inert-gas-line techniques, and long reaction times (commonly 24 h for the first step and 48 h for the second step; Fig. 1b).2,6 In particular, reactions of the poorly soluble chloride-bridged dimer 3 require substantial amounts of solvent, making the second step very slow.1,2,6 These requirements represent major drawbacks from both a production-cost and an environmental perspective. Although microwave irradiation can accelerate the ligand-exchange reactions, a large excess of the ligands (>50 equiv.) is required.9
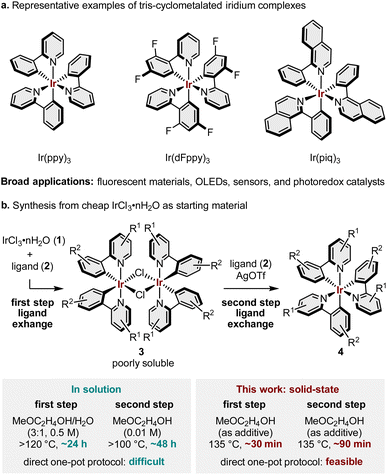 |
| Fig. 1 Synthesis of tris-cyclometalated iridium(III) complexes bearing N-heteroaryl ligands. | |
As a new tool for conducting solvent-less solid-state organic transformations, ball milling has attracted considerable interest.10–13 The advantages of solid-state mechanochemical synthesis include the avoidance of harmful organic solvents, much faster reaction kinetics, and simpler operational handling. In addition to these benefits, poorly soluble materials that are barely reactive under solution-based conditions can often be used as substrates.10,11 Inspired by these attractive features of ball-milling reactions, we envisioned that solid-state mechanochemistry could potentially provide an efficient, rapid, low-cost, and environmentally friendly synthetic route to various tris-cyclometalated iridium(III) complexes.10k,14
In this study, we succeeded in developing a two-step mechanochemical protocol that enables the rapid solid-state synthesis of various tris-cyclometalated iridium(III) complexes (4) from readily available and relatively cheap iridium(III) chloride hydrate (1). This protocol represents a practical synthetic solution to the aforementioned drawbacks of conventional solution-based approaches (Fig. 1b). Specifically, the first ligand exchange is complete within 30 min, while the second ligand exchange with silver triflate is complete within 90 min, showcasing the effectiveness of solid-state mechanochemistry. We further demonstrate that a direct one-pot sequential protocol is feasible under these solid-state conditions. These results can be expected to inspire the development of cost-effective, faster, and environmentally friendly production methods for these valuable complexes, as well as the discovery of new phosphorescent materials, sensors, and catalysts.
Results and discussion
All mechanochemical reactions were conducted using a Retsch MM400 mill (1.5 mL stainless-steel milling jar; 30 Hz; 5 mm stainless-steel ball). First, we attempted the reaction between iridium(III) chloride hydrate (1) and 2-phenylpyridine (2a) at room temperature without any additives (entry 1) (Table 1). Unfortunately, the desired product (3a) was not produced. The addition of 2-methoxyethanol (0.20 μL mg−1) as a liquid-assisted-grinding (LAG) additive did not improve the reactivity at room temperature (entry 2). In order to carry out the reaction at higher temperature, we used a commercially available temperature-controllable heat gun, which was placed directly above the ball-milling jar (for details, see the ESI†).11d The reaction was conducted with the heat gun preset to 200 °C, and the internal temperature of the reaction mixture (100 °C) was determined by thermography immediately after opening the milling jar (for details, see the ESI†). Pleasingly, we found that under these conditions, 3a was obtained in 72% yield (entry 3). Further increasing the reaction temperature (135 °C) by increasing the heat gun preset to 300 °C did not improve the yield of 3a (70%, entry 4). The reaction at 135 °C without the LAG additive decreased the yield of 3a (62%; entry 5). Surprisingly, the reaction was complete within 10 min to afford 3a in 80% yield (entry 6), and 5 min was also sufficient (74%; entry 7). The appearance of the solid-state reaction mixture changed from colorless to a yellow solid (Fig. 2). The amount of 2-methoxyethanol used did not significantly affect the reactivity (entries 8 and 9).
Table 1 Optimization of the first step: solid-state ligand exchange of 1 with 2-phenylpyridine (2a)a
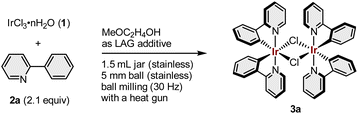
|
Entry |
Amount of MeOC2H4OH (μL mg−1) |
Time (min) |
Internal temp.b (°C) |
Yield (%) of 3ac |
Conditions: 1 (0.20 mmol), 2a (0.42 mmol), 2-methoxyethan-1-ol in a stainless-steel milling jar (1.5 mL) with a stainless-steel ball (5 mm).
The reaction temperature was controlled using a heat gun (for details, see the ESI). The internal temperature was confirmed by thermography immediately after opening the milling jar.
Isolated yields are shown.
|
1 |
0 |
30 |
30 |
<1 |
2 |
0.20 |
30 |
30 |
<1 |
3 |
0.20 |
30 |
100 |
72 |
4 |
0.20 |
30 |
135 |
70 |
5 |
0 |
30 |
135 |
62 |
6 |
0.20 |
10 |
135 |
80 |
7 |
0.20 |
5 |
135 |
74 |
8 |
0.10 |
10 |
135 |
82 |
9 |
0.30 |
10 |
135 |
80 |
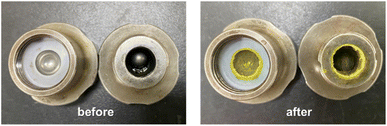 |
| Fig. 2 Reaction mixture before and after ball milling (entry 6, Table 1). | |
Next, we turned our attention to the reaction between 3a and 2a to form the target tris-cyclometalated iridium(III) complex 4a under mechanochemical conditions (Table 2). We found that the reaction with AgOTf and a small amount of 2-methoxyethanol (0.20 μL mg−1) reached completion within 60 min to form 4a in 73% yield. The appearance of the solid-state reaction mixture did not change significantly after the reaction (Fig. 3). Given that the conventional solution-based conditions require a long reaction time (commonly 48 h), it is feasible to conclude that the solid-state mechanochemical strategy greatly accelerates the ligand-exchange process. The addition of AgOTf and high temperature are both essential for this reaction (entries 2 and 3). We found that 30 min of reaction is in general sufficient (70%; entry 4). The use of other silver salts such as Ag2O or Ag2CO3 afforded 4a in lower yield (10%; entry 5 and 14%; entry 6).
Table 2 Optimization of the second step: solid-state ligand exchange of 3a with 2-phenylpyridine (2a) in the presence of silver salta
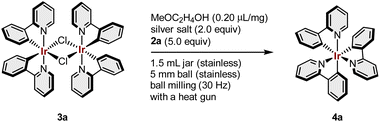
|
Entry |
Silver salt (2.0 equiv.) |
Time (min) |
Internal temp.b (°C) |
Yield (%) of 4ac |
Conditions: 3a (0.05 mmol), silver salt (0.10 mmol), 2a (0.25 mmol), 2-methoxyethan-1-ol (0.20 μL mg−1) in a stainless-steel milling jar (1.5 mL) with a stainless-steel ball (5 mm).
The reaction temperature was controlled using a heat gun (for details, see the ESI). The internal temperature was confirmed by thermography immediately after opening the milling jar.
Isolated yields are shown.
|
1 |
AgOTf |
60 |
135 |
73 |
2 |
None |
60 |
135 |
12 |
3 |
AgOTf |
60 |
30 |
5 |
4 |
AgOTf |
30 |
135 |
70 |
5 |
Ag2O |
60 |
135 |
10 |
6 |
Ag2CO3 |
60 |
135 |
14 |
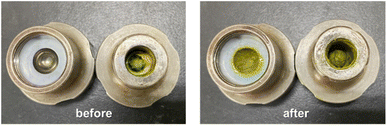 |
| Fig. 3 Reaction mixture before and after ball milling (entry 1, Table 2). | |
With the optimized reaction conditions in hand, we proceeded to synthesize various tris-cyclometalated iridium(III) complexes via the newly developed two-step solid-state protocol. Most of the iridium chloride dimers 3 are sparingly soluble in common organic solvents, which complicates their isolation and characterization. Therefore, the crude products obtained by washing with water and CH2Cl2 after the first ligand exchange were used directly in the second ligand exchange under mechanochemical conditions (for details, see the ESI†). We found that the developed two-step protocol allowed the preparation of iridium complexes that bear fluoro- and trifluoromethyl groups (4a–4d) in good yield (32–61% over two steps) and a short reaction time (10 min for the first step and 60 min for the second step). The reactions with 2-(p-tolyl)pyridine (2e) also proceeded smoothly to give 4e (25% over two steps). Unfortunately, the second ligand exchange did not produce the iridium complex with a diphenylamino group (4f) under the optimized conditions. However, we found that a modification of the conditions, in which N,N-dimethylformamide (DMF) was used as the LAG additive (0.40 μL mg−1) in a 5 mL jar with a 10 mm ball at 20 Hz, allowed the synthesis of 4f in 21% yield over two steps. These modified conditions were also effective for the reaction with 1-phenylisoquinoline (2g) to give 4g in good yield (56% over two steps). Although further investigations are needed, we speculate at present that the higher mechanical impact from the larger ball (10 mm) may be important to achieve better mixing, especially for dimer complexes with particularly low solubility and thus facilitate the second ligand-exchange reaction. The reactions with 1-[3-(trifluoromethyl)phenyl]isoquinoline (2h) and 1-(benzofuran-2-yl)isoquinoline (2i) proceeded to give 4h and 4i 53% and 61% yield over two steps, respectively. It should be noted here that the generation of meridional tris-cyclometalated iridium(III) complexes was not observed in the crude reaction mixtures, and the facial complexes were obtained exclusively under the applied mechanochemical conditions (Table 3).13
Table 3 Solid-state synthesis of various tris-cyclometalated iridium(III) complexesa,b
Conditions: for the first step: 1 (0.20 mmol), 2 (0.42 mmol), 2-methoxyethan-1-ol (0.20 μL mg−1) in a stainless-steel milling jar (1.5 mL) with a stainless-steel ball (5 mm). Crude yields are shown. For the second step: 3 (0.05 mmol), AgOTf (0.10 mmol), 2 (0.25 mmol), 2-methoxyethan-1-ol (0.20 μL mg−1) in a stainless-steel milling jar (1.5 mL) with a stainless-steel ball (5 mm). Isolated yields are shown.
DMF (0.50 μL mg−1) was used in a 5 mL jar with a 10 mm ball.
|
|
In some cases, the conventional solution-based protocol is not efficient, not even under harsh reaction conditions due to the low solubility of the iridium chloride dimers 3.1,2,6 For example, the iridium dimer 3j obtained from the reaction using 2-phenylquinoline (2j) is only very poorly soluble in common organic solvents, and the second ligand exchange did not proceed under solution-based conditions neither at 100 °C nor at 135 °C (Scheme 1). However, we found that our developed solid-state conditions allowed the synthesis of 4j from 3j in 31% yield over two steps. This result demonstrates the high practical utility of this solid-state approach.
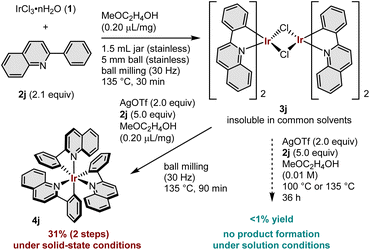 |
| Scheme 1 Mechanochemical synthesis of tris-cyclometalated iridium(III) complex 4j from 2-phenylquinoline 2j. | |
To further showcase the practical utility of this method, we investigated a direct one-pot sequential protocol using mechanochemistry (Scheme 2). For this purpose, the crude mixture including 3 was directly subjected to a one-pot solid-state ligand exchange with AgOTf. We found that the desired tris-cyclometalated iridium complexes (4a, 4b, and 4i) were obtained in good yield over two steps (Scheme 2a). In contrast, our attempt at a one-pot protocol under conventional solution conditions was unsuccessful, and 4a was not obtained (Scheme 2b). These results suggest that the one-pot mechanochemical strategy enables the rapid construction of various tris-cyclometalated iridium complexes under simple operational conditions and can reduce the amount of solvent waste.
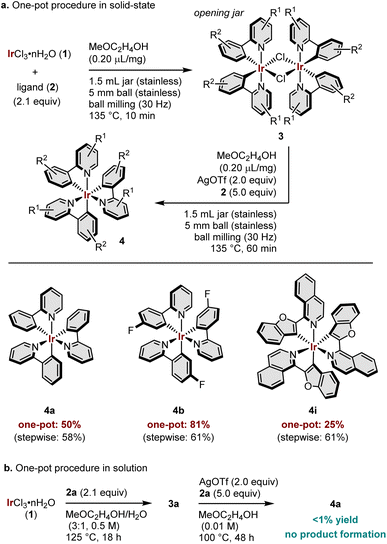 |
| Scheme 2 One-pot sequential ligand-exchange reactions. | |
Acetylacetonato cyclometalated iridium(III) complexes have also been widely used as luminescent materials.1,2,7,8 We found that the reaction of 3a with pentane-2,4-dione in the presence of 2-methoxyethanol (0.20 μL mg−1) as a LAG additive under ball-milling conditions afforded the desired acetylacetonato complex 5 in excellent yield (83%) (Scheme 3). Furthermore, the reaction of 3a with 4,4′-di-tert-butyl-2,2′-bipyridyl under mechanochemical conditions also proceeded smoothly to furnish the corresponding cationic tris-cyclometalated iridium complex in high yield (88%) (Scheme 3).15 Notably, these reactions reach completion within 30 min, while conventional solution conditions usually require >24 h.15 These results highlight the considerable potential of this solid-state mechanochemical synthetic approach for the rapid preparation of various luminescent iridium complexes in an economically and environmentally friendly manner.
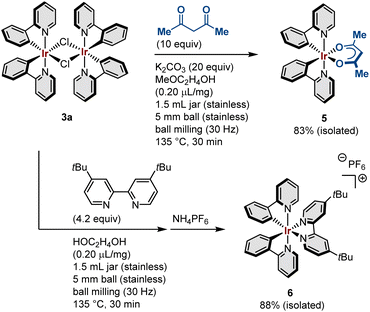 |
| Scheme 3 Mechanochemical synthesis of Ir(ppy)2(acac) complex (5) and [Ir(ppy)2(dtbpy)]PF6 (6). | |
In order to quantify the environmental benefits of this mechanochemical method, we evaluated the E-factor of the present solid-state conditions to those of the previously reported representative solution-based conditions in the literature.2,6,14h,16 The E-factor is an index for the quantitative evaluation of the environmental impact of a chemical process.16 For our solid-state method, the E-factors are 1.39 for the first step and 4.83 for the second step, whereas the E-factors of a representative solution-based method are 2.16 for the first step and 76.88 for the second step (Table 4). This difference is mainly due to the absence of bulk solvents under our solid-state conditions. According to these results, the present solid-state approach is substantially more eco-friendly than conventional solution-based approaches.
Table 4 Calculation of the E-factorsa
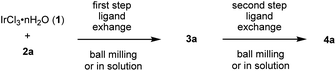
|
Conditions |
E-factor for the first step |
E-factor for the second step |
For the details of the calculations, see the ESI.
|
Mechanochemical conditions (this work) |
1.39 |
4.82 |
Solution-based conditions2,6 |
2.16 |
76.88 |
Conclusions
In conclusion, we have developed a solid-state protocol that allows the rapid synthesis of various tris-cyclometalated iridium(III) complexes from relatively cheap iridium(III) chloride hydrate without the use of significant amounts of high-boiling solvents. Notably, these mechanochemical reactions reach completion in much shorter reaction times than those required for solution-based protocols, and all experimental operations can be carried out in air. The present study can thus be feasibly expected to inspire the development of highly efficient, cost-effective, time-saving, and environmentally friendly routes to valuable iridium-based luminescent materials, sensors, and catalysts.
Data availability
All experimental data is available in the ESI.†
Author contributions
K. K. and H. I. conceived and designed the study. K. K. and H. I. co-wrote the paper. K. K. and T. E. performed the chemical experiments and analyzed the data. All authors discussed the results and commented on the manuscript.
Conflicts of interest
There are no conflicts to declare.
Acknowledgements
This work was supported by the Japan Society for the Promotion of Science (JSPS) via KAKENHI grants 22H00318, 21H01926, 22K18333, and 22H05328; by the JST via CREST grant JPMJCR19R1; by FOREST grant JPMJFR201I; and by the Institute for Chemical Reaction Design and Discovery (ICReDD), which was established by the World Premier International Research Initiative (WPI), MEXT, Japan. The authors also would like to thank Mitsubishi Chemical Corporation for their cooperation and helpful discussions. We thank Mr. Naoki Shizukuishi for his help in cross-checking the experiments.
Notes and references
- For selected reviews and examples of applications of tris-cyclometalated iridium(III) complexes as functional luminescent materials, see:
(a) F. Monti, A. Baschieri, L. Sambri and N. Armaroli, Acc. Chem. Res., 2021, 54, 1492 CrossRef CAS PubMed;
(b) R. D. Costa, E. Ortí, H. J. Bolink, F. Monti, G. Accorsi and N. Armaroli, Angew. Chem., Int. Ed., 2012, 51, 8178 CrossRef CAS PubMed;
(c) Y. Chi and P.-T. Chou, Chem. Soc. Rev., 2010, 39, 638 RSC;
(d) C. Fan and C. Yang, Chem. Soc. Rev., 2014, 43, 6439 RSC;
(e) H. Yersin, A. F. Rausch, R. Czerwieniec, T. Hofbeck and T. Fischer, Coord. Chem. Rev., 2011, 255, 2622 CrossRef CAS;
(f) K. K.-W. Lo and S. P.-Y. Li, RSC Adv., 2014, 4, 10560 RSC;
(g)
E. Zysman-Colman, Iridium(III) in Optoelectronic and Photonics Applications, John Wiley & Sons, 2017 CrossRef;
(h) M. Penconi, A. B. Kajjam, M.-C. Jung, M. Cazzaniga, C. Baldoli, D. Ceresoli, M. E. Thompson and A. Bossi, Chem. Mater., 2022, 34, 574 CrossRef CAS;
(i) A. K. Pal, S. Krotkus, M. Fontani, C. F. R. Mackenzie, D. B. Cordes, A. M. Z. Slawin, I. D. W. Samuel and E. Zysman-Colman, Adv. Mater., 2018, 30, 1804231 CrossRef PubMed;
(j) A. Baschieri, L. Sambri, A. Mazzanti, A. Carlone, F. Monti and N. Armaroli, Inorg. Chem., 2020, 59, 16238 CrossRef CAS PubMed;
(k) J.-H. Kim, S.-Y. Kim, S. Jang, S. Yi, D. W. Cho, H.-J. Son and S. O. Kang, Inorg. Chem., 2019, 58, 16112 CrossRef CAS PubMed.
- For selected pioneering studies on the synthesis and characterization of tris-cyclometalated iridium(III) complexes, see:
(a) K. A. King, P. J. Spellane and R. J. Watts, J. Am. Chem. Soc., 1985, 107, 1431 CrossRef CAS;
(b) M. A. Baldo, S. Lamansky, P. E. Burrows, M. E. Thompson and S. R. Forrest, Appl. Phys. Lett., 1999, 75, 4 CrossRef CAS;
(c) V. V. Grushin, N. Herron, D. D. LeCloux, W. J. Marshall, V. A. Petrov and Y. Wang, Chem. Commun., 2001, 1494 RSC;
(d) S. Lamansky, P. Djurovich, D. Murphy, F. Abdel-Razzaq, H.-E. Lee, C. Adachi, P. E. Burrows, S. R. Forrest and M. E. Thompson, J. Am. Chem. Soc., 2001, 123, 4304 CrossRef CAS PubMed;
(e) A. B. Tamayo, B. D. Alleyne, P. I. Djurovich, S. Lamansky, I. Tsyba, N. N. Ho, R. Bau and M. E. Thompson, J. Am. Chem. Soc., 2003, 125, 7377 CrossRef CAS PubMed;
(f) A. Tsuboyama, H. Iwawaki, M. Furugori, T. Mukaide, J. Kamatani, S. Igawa, T. Moriyama, S. Miura, T. Takiguchi, S. Okada, M. Hoshino and K. Ueno, J. Am. Chem. Soc., 2003, 125, 12971 CrossRef CAS PubMed;
(g) Y. You and S. Y. Park, J. Am. Chem. Soc., 2005, 127, 12438 CrossRef CAS PubMed.
- For selected examples of applications as pH or anion sensors, see:
(a) R. S. Vadavi, H. Kim, K. M. Lee, T. Kim, J. Lee, Y. S. Lee and M. H. Lee, Organometallics, 2012, 31, 31 CrossRef CAS;
(b) Q. Zhao, F. Li, S. Liu, M. Yu, Z. Liu, T. Yi and C. Huang, Inorg. Chem., 2008, 47, 9256 CrossRef CAS PubMed;
(c) A. Kando, Y. Hisamatsu, H. Ohwada, T. Itoh, S. Moromizato, M. Kohno and S. Aoki, Inorg. Chem., 2015, 54, 5342 CrossRef CAS PubMed.
- For a selected example of an application as a bioimaging probe, see: A. Baschieri, S. Muzzioli, V. Fiorini, E. Matteucci, M. Massi, L. Sambri and S. Stagni, Organometallics, 2014, 33, 6154 CrossRef CAS.
- For selected reviews of applications as photoredox catalysts, see:
(a) A. Y. Chan, I. B. Perry, N. B. Bissonnette, B. F. Buksh, G. A. Edwards, L. I. Frye, O. L. Garry, M. N. Lavagnino, B. X. Li, Y. Liang, E. Mao, A. Millet, J. V. Oakley, N. L. Reed, H. A. Sakai, C. P. Seath and D. W. C. MacMillan, Chem. Rev., 2022, 122, 1485 CrossRef CAS PubMed;
(b) C. K. Prier, D. A. Rankic and D. W. C. MacMillan, Chem. Rev., 2013, 113, 5322 CrossRef CAS PubMed;
(c) K. L. Skubi, T. R. Blum and T. P. Yoon, Chem. Rev., 2016, 116, 10035 CrossRef CAS PubMed.
- M. G. Colombo, T. C. Brunold, T. Riedener, H. U. Guedel, M. Fortsch and H.-B. Buergi, Inorg. Chem., 1994, 33, 545 CrossRef CAS.
- K. Dedeian, P. I. Djurovich, F. O. Garces, G. Carlson and R. J. Watts, Inorg. Chem., 1991, 30, 1685 CrossRef CAS.
- S. Lamansky, P. Djurovich, D. Murphy, F. Abdel-Razzaq, R. Kwong, I. Tsyba, M. Bortz, B. Mui, R. Bau and M. E. Thompson, Inorg. Chem., 2001, 40, 1704 CrossRef CAS PubMed.
- For selected examples of the use of microwave irradiation, see:
(a) H. Konno and Y. Sasaki, Chem. Lett., 2003, 32, 252 CrossRef CAS;
(b) S. Ikawa, S. Yagi, T. Maeda, H. Nakazumi, H. Fujiwara, S. Koseki and Y. Sakurai, Inorg. Chem. Commun., 2013, 38, 14 CrossRef CAS.
- For recent reviews on organic synthesis using mechanochemistry, see:
(a) S. L. James, C. J. Adams, C. Bolm, D. Braga, P. Collier, T. Friščić, F. Grepioni, K. D. M. Harris, G. Hyett, W. Jones, A. Krebs, J. Mack, L. Maini, A. G. Orpen, I. P. Parkin, W. C. Shearouse, J. W. Steed and D. C. Waddell, Chem. Soc. Rev., 2012, 41, 413 RSC;
(b) G.-W. Wang, Chem. Soc. Rev., 2013, 42, 7668 RSC;
(c) J.-L. Do and T. Friščić, ACS Cent. Sci., 2017, 3, 13 CrossRef CAS PubMed;
(d) J. G. Hernández and C. Bolm, J. Org. Chem., 2017, 82, 4007 CrossRef PubMed;
(e) J. G. Hernández, Chem.–Eur. J., 2017, 23, 17157 CrossRef PubMed;
(f) T. K. Achar, A. Bose and P. Mal, Beilstein J. Org. Chem., 2017, 13, 1907 CrossRef CAS PubMed;
(g) J.-L. Do and T. Friščić, Synlett, 2017, 28, 2066 CrossRef CAS;
(h) D. Tan and T. Friščić, Eur. J. Org
Chem., 2018, 2018, 18 CrossRef CAS;
(i) J. L. Howard, Q. Cao and D. L. Browne, Chem. Sci., 2018, 9, 3080 RSC;
(j) J. Andersen and J. Mack, Green Chem., 2018, 20, 1435 RSC;
(k) N. R. Rightmire and T. P. Hanusa, Dalton Trans., 2016, 45, 2352 RSC;
(l) M. Leonardi, M. Villacampa and J. C. Menéndez, Chem. Sci., 2018, 9, 2042 RSC;
(m) D. Tan, L. Loots and T. Friščić, Chem. Commun., 2016, 52, 7760 RSC;
(n) D. Tan and F. García, Chem. Soc. Rev., 2019, 48, 2274 RSC;
(o) C. Bolm and J. G. Hernández, Angew. Chem., Int. Ed., 2019, 58, 3285 CrossRef CAS PubMed;
(p) T. Friščić, C. Mottillo and H. M. Titi, Angew. Chem., Int. Ed., 2020, 59, 1018 CrossRef PubMed;
(q) K. Kubota and H. Ito, Trends Chem., 2020, 2, 1066 CrossRef CAS;
(r) I. N. Egorov, S. Santra, D. S. Kopchuk, I. S. Kovalev, G. V. Zyryanov, A. Majee, B. C. Ranu, V. L. Rusinov and O. N. Chupakhin, Green Chem., 2020, 22, 302 RSC;
(s) A. Porcheddu, E. Colacino, L. De Luca and F. Delogu, ACS Catal., 2020, 10, 8344 CrossRef CAS;
(t) P. Ying, J. Yu and W. Su, Adv. Synth. Catal., 2021, 363, 1246 CrossRef CAS;
(u) K. J. Ardila-Fierro and J. G. Hernández, ChemSusChem, 2021, 14, 2145 CrossRef CAS PubMed;
(v) A. C. Jones, J. A. Leitch, S. E. Raby-Buck and D. L. Browne, Nat. Synth., 2022, 1, 763 CrossRef;
(w) V. Martinez, T. Stolar, B. Karadeniz, I. Brekalo and K. Užarević, Nat. Rev. Chem, 2023, 7, 51 CrossRef CAS PubMed;
(x) K. Kubota, Bull. Chem. Soc. Jpn., 2023, 96, 913 CrossRef CAS.
- For selected examples of solid-state organic transformations using ball milling from our group, see:
(a) K. Kubota, Y. Pang, A. Miura and H. Ito, Science, 2019, 366, 1500 CrossRef CAS PubMed;
(b) K. Kubota, R. Takahashi and H. Ito, Chem. Sci., 2019, 10, 5837 RSC;
(c) K. Kubota, T. Seo, K. Koide, S. Hasegawa and H. Ito, Nat. Commun., 2019, 10, 111 CrossRef PubMed;
(d) T. Seo, T. Toyoshima, K. Kubota and H. Ito, J. Am. Chem. Soc., 2021, 143, 6165 CrossRef CAS PubMed;
(e) Y. Pang, J. W. Lee, K. Kubota and H. Ito, Angew. Chem., Int. Ed., 2020, 59, 22570 CrossRef CAS PubMed;
(f) K. Kubota, N. Toyoshima, D. Miura, J. Jiang, S. Maeda, M. Jin and H. Ito, Angew. Chem., Int. Ed., 2021, 60, 16003 CrossRef CAS PubMed;
(g) R. Takahashi, A. Hu, P. Gao, Y. Gao, Y. Pang, T. Seo, J. Jiang, S. Maeda, H. Takaya, K. Kubota and H. Ito, Nat. Commun., 2021, 12, 6691 CrossRef CAS PubMed;
(h) R. Takahashi, P. Gao, K. Kubota and H. Ito, Chem. Sci., 2023, 14, 499 RSC;
(i) T. Seo, K. Kubota and H. Ito, J. Am. Chem. Soc., 2023, 145, 6823 CrossRef CAS PubMed.
-
(a)
K. Tanaka, Solvent-Free Organic Synthesis, Wiley-VCH, Weinheim, 2009 Search PubMed;
(b)
F. Toda, Organic Solid-State Reactions, Springer, Berlin, Heidelberg, 2004 Search PubMed;
(c) F. Toda, Acc. Chem. Res., 1995, 28, 480 CrossRef CAS;
(d) K. Tanaka and F. Toda, Chem. Rev., 2000, 100, 1025 CrossRef CAS PubMed.
- T. Karatsu, T. Nakamura, S. Yagai, A. Kitamura, K. Yamaguchi, Y. Matsushima, T. Iwata, Y. Hori and T. Hagiwara, Chem. Lett., 2003, 32, 886 CrossRef CAS.
- For selected examples of mechanochemical synthesis of organometallic compounds, see:
(a) N. R. Rightmire, T. P. Hanusa and A. L. Rheingold, Organometallics, 2014, 33, 5952 CrossRef CAS;
(b) R. F. Koby, T. P. Hanusa and N. D. Schley, J. Am. Chem. Soc., 2018, 140, 15934 CrossRef CAS PubMed;
(c) A. Beillard, T.-X. Métro, X. Bantreil, J. Martinez and F. Lamaty, Chem. Sci., 2017, 8, 1086 RSC;
(d) F. Quintin, J. Pinaud, F. Lamaty and X. Bantreil, Organometallics, 2020, 39, 636 CrossRef CAS;
(e) A. Beillard, F. Quintin, J. Gatignol, P. Retailleau, J.-L. Renaud, S. Gaillard, T.-X. Métro, F. Lamaty and X. Bantreil, Dalton Trans., 2020, 49, 12592 RSC;
(f)
F. Leon and F. García, Comprehensive Coordination Chemistry III, 2021, vol. 9, p. 620 Search PubMed;
(g) J. Wang, R. Ganguly, L. Yongxin, J. Díaz, H. S. Soo and F. García, Dalton Trans., 2016, 45, 7941 RSC;
(h) J. A. Cabeza, J. F. Reynes, F. García, P. García-Álvarez and R. García-Soriano, Chem. Sci., 2023, 14, 12477 RSC;
(i) J. G. Hernández, N. A. J. Macdonald, C. Mottillo, I. S. Butler and T. Friščić, Green Chem., 2014, 16, 1087 RSC;
(j) J.-L. Do, D. Tan and T. Friščić, Angew. Chem., Int. Ed., 2018, 57, 2667 CrossRef CAS PubMed;
(k) K. Budny-Godlewski, I. Justyniak, M. Leszczyński and J. Lewiński, Chem. Sci., 2019, 10, 7149 RSC;
(l) J. Lewiński, M. Dutkiewicz, M. Lesiuk, W. Śliwiński, K. Zelga, I. Justyniak and J. Lipkowski, Angew. Chem. Int. Ed., 2010, 49, 8266 CrossRef PubMed;
(m) K. Budny-Godlewski, M. K. Leszczyński, A. Tulewicz, I. Justyniak, D. Pinkowicz, B. Sieklucka, K. Kruczała, Z. Sojka and J. Lewiński, ChemSusChem., 2021, 14, 3887 CrossRef CAS PubMed.
- J. D. Slinker, A. A. Gorodetsky, M. S. Lowry, J. Wang, S. Parker, R. Rohl, S. Bernhard and G. G. Malliaras, J. Am. Chem. Soc., 2004, 126, 2763 CrossRef CAS PubMed.
-
(a) R. A. Sheldon, Green Chem., 2007, 9, 1273 RSC;
(b) V. K. Singh, A. Chamberlain-Clay, H. C. Ong, F. León, G. Hum, M. Y. Par, P. Daley-Dee and F. García, ACS Sustainable Chem. Eng., 2021, 9, 1152 CrossRef CAS.
|
This journal is © The Royal Society of Chemistry 2024 |
Click here to see how this site uses Cookies. View our privacy policy here.