DOI:
10.1039/D4SC00926F
(Edge Article)
Chem. Sci., 2024,
15, 8163-8169
Mild and catalytic electrocyclizations of heptatrienyl anions†
Received
6th February 2024
, Accepted 25th April 2024
First published on 26th April 2024
Abstract
We report the synthesis of functionalized cycloheptanes by thermal electrocyclization of heptatrienyl anions under mild conditions. In addition, we disclose the first examples of this electrocyclization manifold conducted under catalytic conditions. Previously, electrocyclization of heptatrienyl systems required formation of anions with a strong base, resulting in limited functional group compatibility. We demonstrate that polarization of heptatrienyl anions using strategically positioned electron-withdrawing groups lowers the energy landscape of the reaction by stabilizing both the acyclic heptatrienyl anion and cycloheptadienyl product. Divergent reactivity is observed between aliphatic and aromatic substrates, with the latter requiring only catalytic amounts of base for complete conversion. This can be rationalized by the relative stability of the acyclic and cyclic anions and their ability to participate in a chain reaction process.
1. Introduction
Ring construction is a priority task in the synthesis of value-added complex molecules. While the synthesis of small rings is routine, medium-sized rings are often difficult to access. Cycloheptanes are commonly found in drug candidates and complex naturally occurring compounds with biological activity of possible therapeutic value. Natural products bearing complex cycloheptanes have indeed served as drug leads or are drugs themselves, as exemplified by englerin,1 and ingenol2 (Fig. 1). A major challenge in their synthesis involves the preparation of a highly functionalized cycloheptane ring.
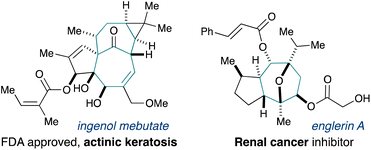 |
| Fig. 1 Selected natural product drug leads bearing functionalized cycloheptanes. | |
In the main, classical cycloheptane synthesis relies on the release of ring strain. For example, the de Mayo reaction3 and the divinyl cyclopropane rearrangement4,5 employ the release of ring strain from cyclobutane or cyclopropane intermediates respectively to drive cycloheptane synthesis.6 Another common approach involves one-carbon ring expansion of cyclohexanes, enabled by the Tiffeneau-Demjanov7 and pinacol-type rearrangements,8 or the ring expansion of cyclohexane-fused cyclopropanols.9 Besides ring-closing metathesis, other methods relying on transition metal catalysts have been developed, and they often feature high energy building blocks such as methylenecyclopropanes or vinylcyclopropanols.10,11
Electrocyclization reactions are frequently used to make functionalized cyclopentanes12,13 and cyclohexanes. In contrast, although the electrocyclization of all-carbon heptatrienyl anions has been known for over fifty years, its use in synthesis remains very limited.14 Like other electrocyclizations, this process is thermodynamically driven largely by the formation of a strong sigma C–C bond at the expense of a weaker pi C
C bond. Early studies by Kleinschmidt, Kergomard, Kloozterziel and Bates15–18 relied on strongly basic conditions to generate heptatrienyl anions (eqn (1)). Sporadic studies of this electrocyclization manifold have appeared over the years,19–23 yet all reports involve strongly basic intermediates generated with a stoichiometric base, limiting the functional group tolerance and overall utility of this electrocyclization. We aim to transform this electrocyclization manifold into a more useful method for synthesis and in this report we detail our initial efforts towards this goal.
2. Reaction design and development
Since electrocyclization is largely driven by the formation of a sigma bond, we reasoned that the basicity of the heptatrienyl and cycloheptadienyl anions should be largely irrelevant, provided they are similar (Fig. 2). Put differently, if both substrate and product anions were stabilized by strategically positioned and similar functional groups, the electrocyclization would remain thermodynamically favorable and would proceed under significantly milder conditions. The location of charge-stabilizing groups in the substrates would be guided by the alternating charge localization in the HOMO of the acyclic substrate and cyclic product anions (see inset). Originally we envisioned that if the pKa profile of the substrate, product and base would be within a narrow range, this could perhaps enable a catalytic process in which the conjugate acid formed by deprotonation of the heptatriene could protonate the cycloheptadienyl anion resulting from electrocyclization. Incorporation of activating functional groups would have the added benefit of facilitating substrate synthesis and could be exploited in downstream reactions.
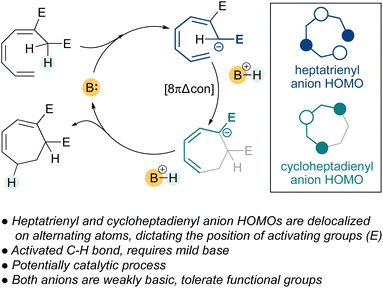 |
| Fig. 2 Design principles for the mild and catalytic electrocyclization of heptatrienes to cycloheptadienes. | |
To begin our study, we prepared a model substrate from cyclopentanone using a Vilsmeier-Haack bromoformylation, a Suzuki coupling, and a Horner–Wadsworth–Emmons reaction (see ESI for details†). This route provides substrates that are pre-organized for electrocyclization and that bear adjacent and nearly identical activating groups. The triene derived from cyclopentanone in this way (S1) was treated with various bases to promote the electrocyclization. Use of an excess of TBAF as the base in THF at ambient temperature provided the desired cycloheptadiene (1) in 59% yield (Table 1, entry 1), however no turnover was observed when TBAF was used in catalytic amounts (entry 2). We then used DBU as a soluble organic base in a variety of solvents. No reaction took place in DCM (entry 3), and a satisfactory 70% yield of the electrocyclization product was obtained in MeCN (entry 4). The yield improved to 80% upon changing to more polar DMSO (entry 5) and to 89% upon increasing the concentration to 1.0 M. Unfortunately, using 10 mol% of base provided only 5% yield of the product. Other amine bases tested failed to give the desired product.
Table 1 Optimization of the electrocyclization of aliphatic substrates

|
|
Change from optimal conditions |
|
1 |
1.1 equiv. TBAF in THF |
59% |
2 |
0.1 equiv. TBAF in THF (0.1 M) |
6% |
3 |
DCM instead of DMSO (0.1 M) |
0% |
4 |
MeCN instead of DMSO (0.1 M) |
70% |
5 |
0.1 instead of 1.0 M |
80% |
6 |
none
|
89%
|
7 |
0.1 equiv. DBU |
5% |
Unexpectedly, we found that the intermediate β-bromoenals prepared from other ketones (i.e., cycloheptanone and cyclooctenone) were unstable and this limited access to substrates through our initial approach. The triene products were also unexpectedly unstable and this led us to investigate substrates bearing an embedded benzene ring. These benzotrienes can be accessed from the corresponding ortho-bromobenzaldehydes through a Suzuki coupling and a Horner–Wadsworth–Emmons reaction (See ESI for full details†). We note in passing that all known examples of electrocyclization of heptatrienes that incorporate at least one aromatic ring require strongly basic conditions.14,24–26 In contrast to the aliphatic substrate, the reaction of benzotriene S3 using TBAF in THF at ambient temperature failed (Table 2, entry 1), however a 57% yield of benzocycloheptadiene 3 was obtained at elevated temperature (entry 2). Changing the solvent and base to DMSO and DBU provided a very satisfying 90% yield of isolated product (entry 3). Remarkably, the use of a catalytic amount of DBU under similar conditions provided 86% yield of product (entry 4), even at higher concentrations (entry 5). These are the mildest conditions ever reported for electrocyclization of heptatrienyl anions, and the first demonstration of catalysis for this electrocyclization manifold.
Table 2 Optimization of the electrocyclization of aliphatic substrates
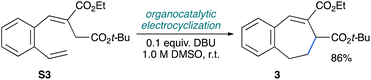
|
|
Change from optimal conditions |
|
1 |
1.1 equiv. TBAF in THF (0.1 M) |
0% |
2 |
1.1 equiv. TBAF in THF (0.1 M), 60 °C |
57% |
3 |
1.1 equiv. DBU in DMSO (0.1 M) |
90% |
4 |
0.1 equiv. DBU in DMSO (0.1 M) |
86%
|
5 |
none
|
86%
|
3. Substrate scope studies
Satisfied with the mild conditions established for this electrocyclization, we began substrate scope studies. The aliphatic substrates we could prepare (Fig. 3, 1 and 2) both cyclized in good or moderate yield but required a stoichiometric base. The parent aromatic compound (3) cyclizes in excellent yield with a catalytic amount of base, and these conditions translate to the chloro-, trifluoromethyl- and fluoro-substituted substrates (4–6). The methoxy-substituted substrate (7) required a stoichiometric base and heating to give a satisfactory yield. This is perhaps not surprising because the methoxy-substituent is electron-donating by resonance, in contrast to all previous entries. Substrates bearing alkyl (8) or aryl groups (9 and 10) on the vinyl fragment also required excess base to reach completion and provided inseparable mixtures of diastereomers. These mixtures likely reflect the thermodynamic position, resulting from equilibration of the stereogenic center alpha to the ester group under reaction conditions, and should not be interpreted as representative of the anion geometry prior to electrocyclization. We prepared geometric isomers of the substrate bearing a nitrile group to stabilize the cyclized product and found that the E-isomer cyclized readily to 11, however the Z-isomer (S12) did not cyclize under a variety of conditions, which suggests that the alkene bearing the nitrile cannot isomerize to adopt the necessary helical geometry prior to electrocyclization. Attempts to make the complementary compound to nitrile 11, with the nitrile and ester groups switched (not shown), failed due to instability of the Horner–Wadsworth–Emmons reagent, which is prone to decomposition by elimination of the cyanide anion. A substrate bearing a terminal ketone group cyclized to give product 13 in 30% yield using 10 mol% DBU and marginally better yield using a stoichiometric base. The complementary substrate with the ketone and ester groups switched (not shown) could not be prepared due to low nucleophilicity of the Horner–Wadsworth–Emmons reagent. Finally, two substrates bearing a pyridine backbone rather than a benzene backbone (14 and 15) both underwent cyclization. These last two examples demonstrate nicely the subtle aspects of this electrocyclization. In the case of 14, the charge in the electrocyclized anion is not resonance-stabilized by the imine-like moiety embedded in the pyridine and, as a result, is sufficiently basic to allow use of a catalytic base. In contrast, in the case of 15, the charge in the electrocyclized anion is directly stabilized by the imine-like moiety in the pyridine, making it insufficiently basic to deprotonate the conjugate acid of the catalytic base or the acyclic heptatriene substrate.
 |
| Fig. 3 Substrate scope of the electrocyclization of heptatrienyl systems. | |
4. Theoretical and mechanistic studies
To gain some understanding of this electrocyclization reaction we conducted a theoretical study with a simpler model system, using amidine as the base and implicit DMSO solvent (Fig. 4).27 The reactions begins with deprotonation of the acidic position with amidine base (R to INT1) via transition state TSC1··H··N. INT1 represents the helical anion hydrogen-bonded to the amidinium ion in DMSO solution. Electrocyclization ensues through transition state TSC1··C7 (the aliphatic TS is shown), which is hydrogen-bonded to the amidinium ion (H-bond omitted for clarity), yielding INT2, the cylcoheptadienyl anion as an ion pair with amidinium. Progression from INT2 to INT3 corresponds to the departure of the amidinium ion from C1 and approach of amidinium to C-6, forming INT3, which has the same cycloheptadienyl anion as INT2 but hydrogen bonded to amidinium. INT3 leads to the final product through proton transfer viaTSC··H··N.
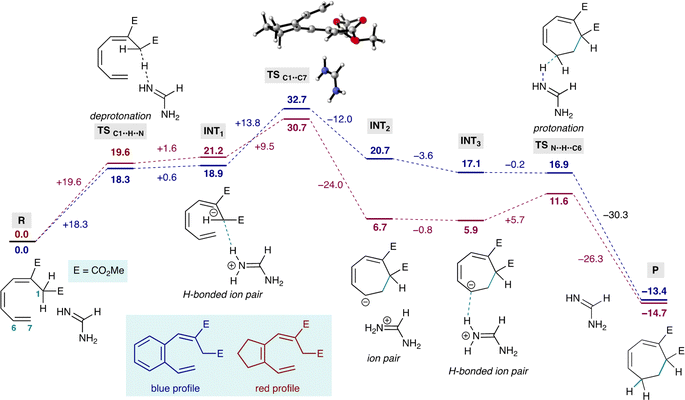 |
| Fig. 4 Calculated reaction coordinate for electrocyclization of heptatrienyl systems bearing a fused aromatic (blue) or aliphatic (red) ring, using amidine base and implicit DMSO solvent. The fused rings are omitted in the reaction profile for clarity. All energies are in kcal mol−1. | |
This study reveals that the electrocyclization of the aliphatic system (red profile) is exergonic by −14.7 kcals mol−1 and the electrocyclization of the aromatic case (blue profile) is exergonic by −13.4 kcals mol−1. This study also reveals that both electrocyclizations have similar overall activation barriers (see ESI for computational results†), with the transition state for electrocyclization occurring at 30.7 kcals mol−1 for the aliphatic case, and 32.7 kcals mol−1 for the aromatic case (Fig. 4). Interestingly, these calculations also suggest that the cyclized aromatic anion is less stable, and therefore more basic than the aliphatic counterpart (c.f.INT2).
The relative energies of the acyclic and cyclic anions immediately before and after electrocyclization (c.f.INT1 and INT2, Fig. 4) reveal that electrocyclization of the aliphatic anion is favourable, and that the electrocyclization of the aromatic anion is energetically uphill. This can be understood intuitively, considering that the aliphatic cycloheptadienyl anion benefits from stabilization by conjugation to the electron-withdrawing group at C2. While the same is true for the aromatic anion, this also involves perturbation of aromaticity, which is reflected in its higher energy.
The dichotomy in the relative stabilities of the acyclic and cyclic anions suggested that a chain reaction may be possible for the aromatic system, with the anionic product of one electrocyclization event acting as the base for the next electrocyclization. To gain insight into this mechanistic scenario we studied the hypothetical reaction of a cycloheptadienyl anion removing the activated proton of the heptatriene substrate to give the corresponding cycloheptadiene and the heptatrienyl anion, for both the parent aromatic and aliphatic systems. In the aromatic case this elementary step is exergonic by −17.5 kcal mol−1 and has an energy barrier of 12.5 kcal mol−1 (eqn (2)).
In contrast, the aliphatic case is almost isoergonic (−0.9 kcal mol−1) and has an energy barrier of 25.4 kcal mol−1 (eqn (3)). These findings are consistent with the requirement of a catalytic base to cyclize the aromatic substrate (S3 to 3, Table 2) and the need to use a stoichiometric base to cyclize the aliphatic substrate (S1 to 1, Table 1).
To determine if a chain reaction is indeed possible, we subjected these substrates to electrocyclization conditions using a strong phosphazene base (P2Et), which has a considerably greater pKBH+ than DBU in organic solvents.28,29 This avoids the possibility of the electrocyclization proceeding through a closed cycle (as in Fig. 2) because the product anion cannot deprotonate the phosphazenium conjugate acid. Treatment of the aromatic substrate S18 with 10% of the P2Et phosphazene base in organic solvents (DMSO, MeCN and THF) resulted in complete conversion of the substrate (NMR) and provided isolated yields of 77–81% of product 18 (eqn (4)). In contrast, when the aliphatic substrate S19 was subjected to the same conditions, little conversion was observed by NMR spectroscopy (eqn (5)).
The observations and calculation for the electrocyclization of aromatic substrates using a catalytic base and proceeding through a chain reaction mechanism are summarized in Fig. 5. This clearly illustrates that although the equilibrium between the acyclic and cyclic anion is slightly favoured towards the acyclic form, it is pulled forward by the exergonic protonation of the cyclic anion by an equivalent of substrate acting as the acid.
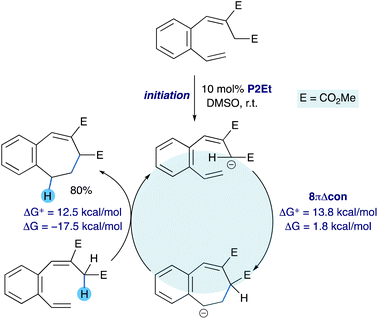 |
| Fig. 5 Chain reaction mechanism for the electrocyclization of heptatrienyl anions bearing an aromatic backbone. | |
5. Discussion
Although the electrocyclization of heptatrienyl anions has been known for over half a century, it has remained obscure and underdeveloped as a tool for synthesis. This may be partly due to the harsh conditions originally reported for anion generation, which lowers functional group tolerance. Indeed, all known examples14 of electrocyclization of heptatrienyl anions use strongly basic intermediates. Given the relative dearth of methods for cycloheptane synthesis we set out to transform this fundamental reactivity into a broadly useful method. Our reaction design stems from the recognition that sigma bond formation is a considerable driving force for electrocyclizations, and that therefore anion basicity in the present electrocyclization manifold should be largely irrelevant as long as both acyclic and cyclic anions in the equilibrium are similarly basic. The relative acidity (or basicity of the resulting anions) can be tuned by installation of suitable electron withdrawing groups at strategic positions to stabilize the anions. The position of these groups is guided by the nature of the highest occupied molecular orbitals of the acyclic and cyclic anions, as we show in Fig. 2. In this initial report we utilized substrates with electron-withdrawing groups with a 1,2-relative disposition, however, as Fig. 2 makes clear, there are other substitution patterns (e.g. 1,4-disubstitution, and so on) that will also achieve this strategic goal. Finally, we originally envisioned a closed catalytic cycle in which a mild exogenous base would serve to generate the stabilized heptatrienyl anion and subsequently protonate the cycloheptadienyl anion.
During our studies we found that aliphatic heptatriene substrates cyclized upon treatment with stoichiometric amounts of the mild organic base DBU. These are the mildest conditions for electrocyclization of heptatrienyl anions ever reported and on their own constitute a useful addition to the synthetic toolbox. Surprisingly, the aliphatic substrates and their products are somewhat unstable, and in addition, other substrates could not be easily made by our synthetic strategy. This obstacle will likely yield other variants of this electrocyclization, using substrates with electron-withdrawing groups at different positions of the heptatrienyl system, for example. We then focused our efforts to the more stable substrate class bearing aromatic rings. These also cyclize under very mild conditions, using DBU as the base, and to our delight the reaction also proceeds using a catalytic base. It bears emphasizing that this is the first demonstration of catalysis for the electrocyclization of heptatrienyl anions.
The divergence in reactivity between the two substrate classes led us to explore the reactions by computation. The reactions have similar activation barriers and thermodynamic driving forces but differ in the relative stability of the acyclic and cyclic anions. Mechanistic experiments using the aromatic substrates and catalytic amounts of strong phosphazene base, which forms a weak conjugate acid, support a mechanism in which the cyclized anion acts as the base for the next electrocyclization event. This is not possible in the electrocyclization of aliphatic substrates because the resulting cycloheptadienyl anion is more stable than the acyclic anion. In contrast, the cyclized anion derived from the aromatic substrate is slightly less stable than the acyclic anion and can deprotonate another molecule of substrate, resulting in a chain reaction mechanism.
6. Concluding remarks
Overall, in this study we have disclosed new design principles for the electrocyclization of heptatrienyl anions, which we hope to extend to other substrate classes in the future. Implementation of this design resulted in the mildest conditions for electrocyclization of heptatrienyl anions ever reported. Finally, we also report the first examples of catalytic electrocyclization of heptatrienyl systems and provide evidence for a chain reaction process.
Author contributions
Faizan Rasheed conducted proof-of-principle experiments, helped with substrate scope studies and helped manage the project. Andrei Nikolaev provided mechanistic insights, conducted experiments that revealed the chain reaction mechanism, and helped with substrate scope. Anmol Dhesi conducted reaction optimization with the aliphatic substrates and helped with substrate scope. Tao Zeng conducted all theoretical work. You Xuan Guo conducted optimization studies with the aromatic substrates and helped with substrate scope. Yarkali Krishna and Samira Komijani helped with substrate scope. Arturo Orellana conceived of this study and managed the project.
Conflicts of interest
There are no conflicts to declare.
Acknowledgements
This work was supported by the Natural Sciences and Engineering Council of Canada through discovery grants for TZ (RGPIN-2016-06276) and AO. TZ thanks York University for funding and the Digital Research Alliance of Canada for computational resources. Faizan Rasheed acknowledges the Dr Douglas Butler Award in Organic Chemistry from Dalton Pharma Services and the Michael Pollard Bursary. Art Orellana thanks professor Jean-Francois Poisson and the SERCO team for hosting a sabbatical stay at Université Grenoble Alpes.
Notes and references
- R. Ratnayake, D. Covell, T. T. Ransom, K. R. Gustafson and J. A. Beutler, Org. Lett., 2009, 11, 57 CrossRef CAS PubMed.
- K. Zechmeister, F. Brandl, W. Hoppe, E. Hecker, H. J. Opferkuch and W. Adolf, Tetrahedron Lett., 1970, 11, 4075 CrossRef.
- P. De Mayo, Acc. Chem. Res., 1971, 4, 41 CrossRef CAS.
- T. Hudlicky, R. Fan, J. W. Reed and K. G. Gadamasetti, Org. React., 1992, 41, 1–133 CAS.
- S. Kruger and T. Gaich, Beilstein J. Org. Chem., 2014, 10, 163 CrossRef PubMed.
-
O. Kulinkovich, Cyclopropanes in Organic Synthesis, Wiley, 2015, ch. 9, pp. 285–332 Search PubMed.
- L. Kürti, B. Czakó and E. J. Corey, Org. Lett., 2008, 10, 5247 CrossRef PubMed.
- L. Jørgensen, S. J. McKerrall, C. A. Kuttruff, F. Ungeheuer, J. Felding and P. S. Baran, Science, 2013, 341, 878 CrossRef PubMed.
- Y. Ito, S. Fujii and T. Saegusa, J. Org. Chem., 1976, 41, 2073 CrossRef CAS.
- P. A. Evans and P. A. Inglesby, J. Am. Chem. Soc., 2008, 130, 12838 CrossRef CAS PubMed.
- P. A. Wender, L. E. Sirois, R. T. Stemmler and T. J. Williams, Org. Lett., 2010, 12, 1604 CrossRef CAS PubMed.
- A. J. Frontier and J. J. Hernandez, Acc. Chem. Res., 2020, 53, 1822 CrossRef CAS PubMed.
- W. T. Spencer III, T. Vaidya and A. J. Frontier, Eur. J. Org. Chem., 2013, 2013, 3621 CrossRef PubMed.
- S. Komijani and A. Orellana, Synthesis, 2024, 56, 701 CrossRef CAS.
- E. A. Zuech, D. L. Crain and R. F. Kleinschmidt, J. Org. Chem., 1968, 33, 771 CrossRef CAS.
- H. Kloosterziel and J. A. A. van Drunen, Recl. Trav. Chim. Pays-Bas, 1969, 88, 1084 CrossRef CAS.
- G. Dauphin, L. David, A. Kergomard and H. Veschambre, Bull. Soc. Chim. Fr., 1970, 3162 CAS.
- R. B. Bates, W. H. Deines, D. A. McCombs and D. E. Potter, J. Am. Chem. Soc., 1969, 91, 4608 CrossRef CAS.
- M. Matsumoto, N. Hoshiya, R. Isobe, Y. Watanabe and N. Watanabe, Tetrahedron Lett., 2004, 45, 3895 CrossRef CAS.
- H. Saitoh, H. K. Ijuin, N. Watanabe and M. Matsumoto, Helv. Chim. Acta, 2013, 96, 1704 CrossRef CAS.
- D. R. Williams, J. T. Reeves, P. P. Nag, W. H. Pitcock and M.-H. Baik, J. Am. Chem. Soc., 2006, 128, 12339 CrossRef CAS PubMed.
- R. Kato, H. Saito, S. Uda, D. Domon, K. Ikeuchi, T. Suzuki and K. Tanino, Org. Lett., 2021, 23, 8878 CrossRef CAS PubMed.
- H. Saito, R. Kato, K. Ikeuchi, T. Suzuki and K. Tanino, Org. Lett., 2021, 23, 9606 CrossRef CAS PubMed.
- L. David and A. Kergomard, C. R. Acad. Bulg. Sci., 1969, 986 CAS.
- G. Dauphin, L. David, P. Duprat, A. Kergomard and H. Veschambre, Synthesis, 1973, 1973, 149 CrossRef.
- F. Auclair, A. Kergomard, H. Veschambre and C. Minot, Tetrahedron, 1975, 31, 2499 CrossRef CAS.
-
CYLview20, Legault, Claude, Y, 2020. http://www.cylview.org Search PubMed.
- R. Schwesinger, H. Schlemper, C. Hasenfratz, J. Willaredt, T. Dambacher, T. Breuer, C. Ottaway, M. Fletschinger, J. Boele, H. Fritz, D. Putzas, H. W. Rotter, F. G. Bordwell, A. V. Satish, G.-Z. Ji, E.-M. Peters, K. Peters, H. G. von Schnering and L. Walz, Liebigs Ann., 1996, 1996, 1055 CrossRef.
- R. Schwesinger and H. Schlemper, Angew. Chem., Int. Ed. Engl., 1987, 26, 1167 CrossRef.
|
This journal is © The Royal Society of Chemistry 2024 |
Click here to see how this site uses Cookies. View our privacy policy here.