DOI:
10.1039/D4SC02839B
(Edge Article)
Chem. Sci., 2024,
15, 14880-14887
Highly stable color-tunable organic long-persistent luminescence from a single-component exciplex copolymer for in vitro antibacterial†
Received
29th April 2024
, Accepted 29th July 2024
First published on 23rd August 2024
Abstract
Developing exciplex-based organic long-persistent luminescence (OLPL) materials with high stability is very important but remains a formidable challenge in a single-component system. Here, we report a facile strategy to achieve highly stable OLPL in an amorphous exciplex copolymer system via through-space charge transfer (TSCT). The copolymer composed of electron donor and acceptor units can not only exhibit effective TSCT for intra/intermolecular exciplex emission but also construct a rigid environment to isolate oxygen and suppress non-radiative decay, thereby enabling stable exciplex-based OLPL emission with color-tunable feature for more than 100 h under ambient conditions. These single-component OLPL copolymers demonstrate robust antibacterial activity against Escherichia coli under visible light irradiation. These results provide a solid example to exploit highly stable exciplex-based OLPL in polymers, shedding light on how the TSCT mechanism may potentially contribute to OLPL in a single-component molecular system and broadening the scope of OLPL applications.
Introduction
Organic long-persistent luminescence (OLPL) has drawn a lot of attention owing to its unique optical phenomenon and important applications in many fields.1–12 A set of strategies including H-aggregation,13,14 host–guest doping,15,16 self-assembly,17,18 exciplex formation19–22 and many other methods10 to realize OLPL have been proposed. Among them, the exciplex strategy, which utilizes the synergistic effect of long-range charge transfer and diffusion between selected donor (D) and acceptor (A) moieties to form stable radical anions and cations as well as gradual electron–hole recombination among radical anions and cations, has been proved to be one of the most successful strategies.23–25 Over the past few years, binary D–A doping26 and trap-assisted ternary D–A doping20 as well as single- and multiple-component ionic crystal systems27–29 have been reported to develop highly efficient exciplex-based OLPL materials. Despite these significant developments, most exciplex-based OLPL materials rely on multi-component charge transportation/recombination under inert environments, wherein the phase separation from a homogeneous mixture in multi-component subjects and strict operating conditions are inevitable, imposing great constraints for practical applications over long timescales.
Notably, besides a multivariate physical blending system, single-component charge-transfer emission can also be achieved by through-space charge transfer (TSCT) in a copolymer system composed of a non-conjugated polymeric backbone as well as pendant D and A units to develop efficient thermally activated delayed fluorescence (TADF) emission.30–33 This TSCT copolymer can support efficient charge transfer to regulate singlet-triplet energy splitting and to modulate the carrier separation/recombination between the physically separated D and A units by controlling the D/A feed ratios; also, the chemically connected copolymer not only can effectively suppress phase separation for long-time operation but also may potentially facilitate the formation of an interpenetrating rigid polymeric environment for inhibition of non-radiative decay. For instance, efficient and full-color TSCT emissions can be realized between physically separated but spatially proximate D and A groups connected by non-conjugated polyethylene and/or polynorbornene backbones.34–36 Inspired by the ingenious strategy in realizing single-component TSCT emission and physically blended exciplex-based OLPL emission, we deduce that the realization of OLPL emission in a single-component copolymer under ambient conditions is reasonable. Theoretically, by carefully implanting pendant D and A groups in a copolymer, the efficient TSCT effect to facilitate the charge transfer process for achieving charge separation between D and A moieties could be anticipated, thus allowing the formation of stable radical anions and cations; thereafter, electrons stabilized by the radical anions can hop among the acceptors for the formation of a stable charge separation state. Eventually, the gradual recombination of electrons and holes originating from radical anions and cations could enable OLPL emission.
To verify our deduction, we design and synthesize a class of exciplex-based OLPL materials through efficient TSCT in a single-component copolymer system by bonding the strong electron-donating molecule N,N-dimethyl-4′-vinyl-[1,1′-biphenyl]-4-amine (DMB) and the strong electron-accepting molecule diphenyl(4-vinylphenyl)phosphine oxide (DPPO) in a non-conjugated polyethylene backbone (Fig. 1a).37,38 In this design, DMB forms a quite stable cation and the phosphine-oxide-based DPPO can not only allow effective charge separation to achieve a stable anion upon interacting with DMB but also afford a rigid environment to suppress non-radiative decay for the realization of OLPL emission. Notionally, an exciplex can boost an effective charge transfer (CT, II) process between the highest occupied molecular orbitals (HOMOs) of DMB and DPPO (Fig. 1b), thus facilitating the construction of stable radical anions and cations; then, electrons can hop from stable DPPO radical anions to DPPO to form charge-separated states (CS, III). Finally, the gradual charge recombination (CR, IV) of DPPO radical anions and DMB radical cations produces OLPL emission (V). OLPL lasts for a long time after photoexcitation ceases. And, because of the small splitting energy between singlet and triplet states (ΔEST) of the TSCT polymer, efficient intersystem crossing (ISC) and reverse ISC (RISC) to boost TADF emission can be achieved (Fig. 1c) for utilization of triplet excitons. Indeed, stable OLPL from a single-component amorphous copolymer was readily achieved and this OLPL emission can remain for more than 100 h under ambient conditions without any encapsulation. More significantly, owing to their highly efficient generation of triplet excitons, OLPL polymers can effectively eliminate at least 78.24% of Escherichia coli (E. coli) when exposed to visible light irradiation via photodynamic therapy. This demonstrates promising antibacterial results for in vitro applications. Our work in purposefully designing and developing single-component exciplex-based OLPL materials through an ingenious TSCT tactic may not only open up a new way for the development of highly stable OLPL but also further propel the application of exciplex-based OLPL in the field of biology.
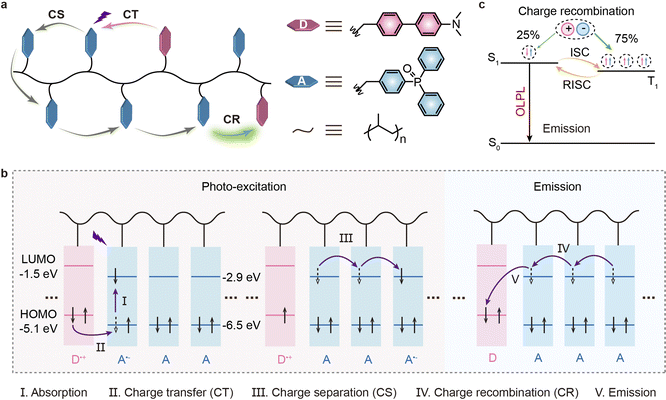 |
| Fig. 1 Schematic representation and mechanism for achieving single-component OLPL polymers. (a) An illustration of a single-component OLPL polymer and chemical structures of the copolymer as well as a plausible OLPL process under UV light. (b) OLPL mechanism of copolymer materials. (c) Exciton generation and utilization in OLPL materials after charge recombination. | |
Results and discussion
Molecular design and characterization
Three copolymers, PDD-99, PDD-199 and PDD-299, were synthesized by radical polymerization of DMB and DPPO monomers with different molar feed ratios of 1
:
99, 1
:
199 and 1
:
299, respectively. In addition, we prepared the homopolymer P-DPPO through the radical polymerization of DPPO for further study of the stable exciplex-based OLPL emission mechanism. The chemical structures of these polymers were characterized by nuclear magnetic resonance spectra, gel permeation chromatography and Fourier transform infrared spectra (Scheme S1, Fig. S1–S7 and Table S1†). Powder X-ray diffraction analysis, thermal property analyses and electrochemical properties are presented in the ESI (Fig. S8–S10 and Table S2†). PDD-99, PDD-199 and PDD-299 can form an amorphous state in the solid form (Fig. S8†) and display good thermal stability (Fig. S9†). The HOMO energy levels of DPPO and DMB were estimated from the onset of the oxidative wave of cyclic voltammetry (CV) experiments (Fig. S10 and Table S2†). And with the aid of optical bandgaps (Eg) determined from the absorption spectra, the lowest unoccupied molecular orbital (LUMO) levels of DMB and DPPO were deduced to be −1.52 and −2.91 eV, respectively. The matched energy levels of the HOMO and LUMO indicate that efficient exciplex emission can be achieved between DMB and DPPO. To gain insight into the electron features of the copolymers, theoretical calculations of HOMO and LUMO electron density and their orbital energy levels were performed. The modeled polymers composed of DMB and DPPO units in molar ratios of 1
:
2, 1
:
5 and 1
:
10 were constructed. As expected, the frontier molecular orbital distributions of HOMO and LUMO localize on the DMB and DPPO (Fig. S11†), respectively, which indicate the TSCT characteristics of the copolymers. And the separated frontier molecular orbitals enable a small ΔEST that facilitates efficient ISC and RISC processes through regulating the molar feed ratios between DMB and DPPO units (Fig. S12†).
Photophysical properties
PDD-99, PDD-199 and PDD-299 powders show broad featureless steady-state photoluminescence (SSPL) spectra with main emission peaks at ∼486 nm, which is substantially redshifted compared to the SSPL emission of DMB and DPPO monomers (Fig. 2a, S13 and S14†). Moreover, the broad SSPL band of PDD-299 shows strong dependence on solvent polarities (Fig. S15†). These results indicate a distinct CT transition between the DMB and DPPO units in PDD-299, suggesting the formation of an exciplex in these single-component copolymers through TSCT.39,40 Strikingly, after turning off the excitation source, PDD-99, PDD-199 and PDD-299 powders exhibit a notable emission with broad OLPL bands (delayed time: 10 ms) at about 486 nm with lifetimes of about 500 ms (Fig. 2a, b, S16, S17 and Table S3†); and, with short delayed time (2 ms), simultaneous emissions involving OLPL (486 nm) and room temperature phosphorescence (530 nm) are observed (Fig. 2a), indicating the presence of multiple luminescent centers (Fig. S18†). The behavior of the lifetime decay profiles of the OLPL band in PDD-299 powder exhibits inverse-power functions of time t−m (m = 0.9), which indicates the presence of CS intermediate states (Fig. 2b) as testified by electron paramagnetic resonance (EPR) measurements showing an enhanced signal after UV excitation (Fig. S19†). These results demonstrate that OLPL from a single-component exciplex copolymer was achieved, though there is still a huge gap in the OLPL duration compared to the reported multi-component systems, possibly due to the fast intra-/intermolecular CR between the DMB and DPPO units in the copolymer. The decay profiles of single-component OLPL copolymers closely depend on the molar feed ratios of the DMB and DPPO units and the intensity of excitation light, which are similar to multi-component OLPL systems.41 When the molar feed ratio between DMB and DPPO units changes from 1
:
99 (PDD-99) to 1
:
199 (PDD-199) to 1
:
299 (PDD-299), the duration of OLPL is significantly improved (Fig. 2b, S16 and S17†). The increased OLPL features may be attributed to the enlarged distance between DMB and DPPO units for reducing the recombination probability of radical cations and anions at higher molar concentrations of DPPO units. The OLPL can be facilely excited by low excitation power and the OLPL properties of PDD-99, PDD-199 and PDD-299 gradually increase when the excitation power density increases from 3.3 to 13.9 mW cm−2 (Fig. 2b, S16 and S17†). These results clearly indicate the gradual accumulation of charge carriers in the developed copolymers. To explore the influence of temperature on the exciplex-based OLPL, OLPL decay measurements of PDD-299 at different temperatures were carried out (Fig. 2c). The stable decay profiles varied from 80 to 300 K indicate that non-radiative transitions by molecular vibrations have been nearly suppressed in PDD-299 powder. The decay profiles of PDD-99 and PDD-199 powders exhibit the same trend as those of PDD-299 (Fig. S20†), showing stable decay profiles at temperatures below 300 K. However, at higher temperatures, the duration for PDD-299 is significantly reduced (Fig. S21†). The suppressed non-radiative deactivation at room temperature may be due to the fact that the main unit of DPPO provides a dense and rigid network environment within the copolymer.
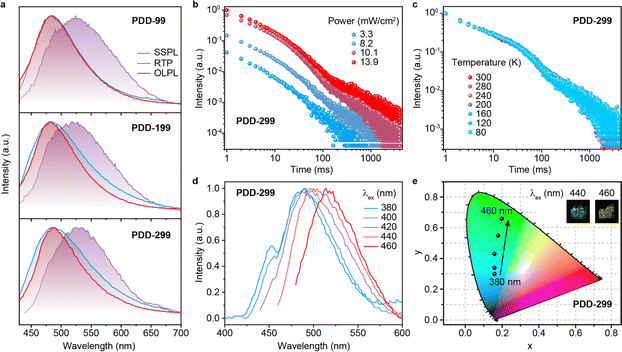 |
| Fig. 2 Photophysical properties of OLPL polymer powders. (a) Steady-state PL, room temperature phosphorescence (delayed time: 2 ms) and OLPL (delayed time: 10 ms) spectra of PDD-99, PDD-199 and PDD-299 (λex = 410 nm) under ambient conditions. (b) Excitation intensity-dependent OLPL decay profiles (λem = 486 nm) of PDD-299 under ambient conditions. (c) Temperature-dependent OLPL decay profiles (λem = 486 nm) of PDD-299 ranging from 300 to 80 K. (d) OLPL spectra (delayed time: 10 ms) and (e) corresponding CIE coordinates of PDD-299 under different excitation wavelengths under ambient conditions. | |
Surprisingly, color-tunable OLPL is observed in the single-component copolymers under different excitation (Fig. 2d, S22 and S23†). With a change of excitation wavelength from 380 nm to 460 nm, the OLPL spectra of PDD-299 exhibit a significant redshift from blue-green to yellowish-green with the main peaks from 480 to 520 nm (Fig. 2d), respectively. PDD-99 and PDD-199 also show obvious color-tunable OLPL emissions with varied emission peaks ranging from 480 to 520 nm when the excitation wavelength is changed from 380 nm to 460 nm (Fig. S22 and S23†) and the corresponding Commission International de l’Eclairage (CIE) coordinates present obvious excitation-dependent properties. As shown in Fig. S24,† the lifetimes of PDD-99, PDD-199 and PDD-299 initially increase when the excitation wavelength increases from 360 to 420 nm, and then the lifetimes gradually decrease when the excitation wavelength increases from 420 to 460 nm. To investigate the origin of the color-tunable properties of the copolymers, we measured the OLPL spectra and decay profiles of homopolymer P-DPPO (Fig. S25 and S26†). The OLPL spectra of P-DPPO exhibit obvious excitation-dependent properties (Fig. S25†), showing varied main emission peaks ranging from 450 to 480 nm when the excitation wavelength is changed from 350 to 430 nm. By comparing the excitation-dependent OLPL spectra between the PDD copolymers and P-DPPO (Fig. S22, S23, and S25†), the main emission peak ranging from 450 to 480 nm of the PDD copolymers should mainly derive from P-DPPO due to the major components of DPPO units in the PDD copolymers; besides, the emission peaks ranging from 480 to 520 nm of the PDD copolymers should principally be caused by exciplex emission. Therefore, the color-tunable OLPL emission in the PDD copolymers may arise from different luminescent centers including homopolymer P-DPPO and exciplex emission upon being excited at different wavelengths.
Mechanism of OLPL polymers
To further verify the involvement of dual luminescent centers in exciplex-based OLPL, additional photophysical property investigations based on PDD-299 and P-DPPO were carried out. Compared to P-DPPO, PDD-299 exhibits broader and red-shifted SSPL and OLPL spectra (Fig. 3a and b), suggesting again the existence of an exciplex as verified by the red-shifted excitation spectra (Fig. 3c and S27†). Moreover, PDD-299 exhibits a main excitation peak at ∼416 nm and a shoulder peak at ∼400 nm, similar to P-DPPO (Fig. 3c). Interestingly, with decreasing temperature, a red-shifted emission peak from 520 to 550 nm is observed and becomes the dominant emission in the OLPL spectra of PDD-299 powder, which is also identical to that of the P-DPPO powder (Fig. S28†), indicating that the emission from homopolymer P-DPPO should be involved in the emission of PDD polymers. To figure out the origin of the emission bands at 530 nm, the concentration-dependent OLPL emission based on P-DPPO was studied. Red-shifted emission bands from 500 to 520 nm are observed with increasing concentration of P-DPPO from 1.5 to 20 mg mL−1 (Fig. S29†) in solution, thus suggesting that the emission peaks from 520 to 550 nm may derive from the radiative decay from the DPPO aggregates due to the shortened distance between DPPO units at low temperatures.42 These observations provide further evidence that the OLPL emission in the PDD copolymers arises from the two emissive species including homopolymer P-DPPO and exciplex emission.
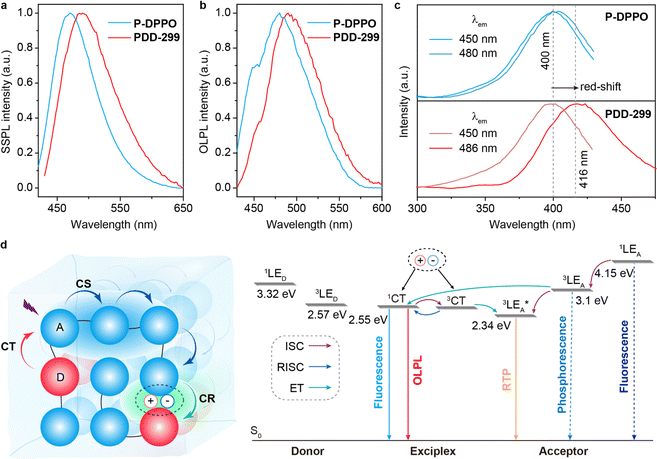 |
| Fig. 3 Proposed mechanism of OLPL materials under ambient conditions. SSPL (a) and OLPL (b, delayed time: 10 ms) spectra of P-DPPO and PDD-299 powders. (c) Excitation spectra for P-DPPO and PDD-299 powders through monitoring various OLPL emission peaks. (d) Scheme for the formation of radical pair through CT, CS and CR processes (left) and plausible energy transfer processes to achieve exciplex-based OLPL emission from a single-component copolymer system. | |
Based on the comprehensive experimental findings, we can propose a plausible mechanism for achieving OLPL in a single-component copolymer system through TSCT under ambient conditions, as illustrated in Fig. 3d. Under photoexcitation, the copolymer system can effectively achieve CT, CS and CR processes between donor and acceptor units to enable exciplex-based OLPL emission. The effective separation of the HOMO on donor and LUMO on acceptor in the exciplex results in a minimal energy gap between the lowest excited singlet (1CT) and triplet (3CT) states of the exciplex.43–45 After the CR process, 1CT and 3CT excitons are generated. Due to the small energy gap between 1CT and the lowest triplet energy of acceptor (3LEA), the processes of ISC and RISC could occur, which is like the process in TADF materials, thus affording OLPL emission from 1CT of the exciplex. Furthermore, as the main component in the copolymer, unit A can also realize OLPL emission, enabling the co-occurrence of emission from exciplex and homopolymer for color-tunable OLPL under different excitations. Notably, due to the relatively large energy gap between 1CT and
of aggregates, the TADF activity between 1CT and
is significantly suppressed, thus leading to much enhanced phosphorescence from aggregates under cryogenic conditions.
Stability performance of OLPL polymers
To verify the much-enhanced stability of exciplex-based OLPL from the single-component copolymer system, a binary exciplex BDD was prepared by physically blending DMB and DPPO at a molar ratio of 1
:
299. As shown in Fig. 4a, the BDD powder shows almost uniform SSPL and OLPL spectra. Compared to SSPL spectra of DMB and DPPO, the red-shifted and broad featureless SSPL spectra of BDD confirm the formation of the exciplex. The decay profiles exhibit obvious power-law emission behavior, which demonstrates that the OLPL emission of BDD also originates from intermediate CS states (Fig. 4b). Although BDD exhibits slightly longer decay times than PDD-299, the stability of BDD is significantly lower than that of PDD-299 under ambient conditions. Compared to the pristine state (Fig. 4c–e), after being kept for 100 hours under ambient conditions, the OLPL intensity of BDD is largely decreased. In contrast, PDD-299 powder exhibits excellent stability (Fig. 4d), showing obvious OLPL emission with a retained OLPL intensity of 70% (Fig. 4e) after being placed for 100 hours under ambient conditions. The poor long-term stability of BDD may be caused by phase separation of the multi-component host–guest system, thus leading to the aggregation of DPPO units. PDD-299 powder presents a much stabler exciplex-based OLPL in a variety of atmospheres than multi-component OLPL materials that need inert environments (Fig. S30†), showing slightly enhanced OLPL intensity in argon and decreased OLPL intensity in oxygen compared to that in air.
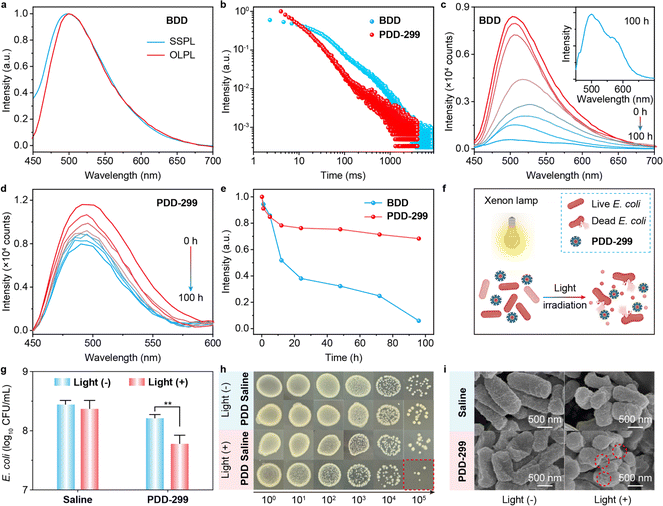 |
| Fig. 4 Stability properties and in vitro antibacterial activity of OLPL polymers. (a) SSPL and OLPL (delayed time: 10 ms) spectra of BDD under ambient conditions. (b) Decay profiles of OLPL emission of BDD (λem = 500 nm) and PDD-299 (λem = 486 nm) under ambient conditions. (c–e) OLPL spectra ((c) and (d); delayed time: 10 ms) and corresponding intensity profiles (e) of BDD (c) and PDD-299 (d) at different kept times under ambient conditions. Inset shows the OLPL (delayed time: 10 ms) spectrum of BDD after being placed in the atmosphere for 100 hours. Note that the excitation wavelength is fixed at 410 nm. (f) Schematic illustration of the inactivation of E. coli killed by PDD-299 under light irradiation. CFU number (g) and original images (h) of E. coli after different treatments (n = 3; mean ± SD). (i) Scanning electron microscope (SEM) images of E. coli after different treatments; red circles indicate bacterial structural damage. | |
In vitro antibacterial activity of OLPL polymers
In light of the effective population of triplet excitons through gradual recombination of radical anions and radical cations, we investigated the potential application of PDD-299 as an in vitro antibacterial (Fig. 4f) through photodynamic therapy for generation of singlet oxygen (Fig. S31†). The generated singlet oxygen can disrupt the antioxidant defense mechanisms of bacterial cells, ultimately leading to the bacterial damage and death. E. coli, a typical Gram-negative bacterium, which can pose potential harm to human health, was selected. Here, using visible light as the excitation source and physiological saline as a standard reference, we investigated the bactericidal capability of PDD-299 through plate colony counting. The 78.24% inactivation efficiency of E. coli induced by PDD-299 after continuous irradiation under visible light for 4 h is significantly higher compared to the inactivation efficiency of saline and the control group without light irradiation (Fig. 4g and h); these results are comparable to those for recently reported in vitro antibacterial materials (Table S4†).46–50 Compared to E. coli treated with saline, which remained intact or was slightly wrinkled after light irradiation, the membrane of E. coli cells treated with PDD-299 showed significant structural damage after light irradiation (Fig. 4i), further indicating the exceptional antibacterial capability of PDD-299 under visible light irradiation.
Conclusions
In summary, we have succeeded in proposing an ingenious approach to achieve color-tunable exciplex-based OLPL with high stability in an amorphous single-component copolymer system. The tactic involves the effective charge transfer between DMB donor and DPPO acceptor through TSCT to enable stable radical anions and cations for affording intra/intermolecular exciplex emission. The single-component copolymers yield quite stable exciplex-based OLPL emission for 100 h under various atmospheres due to the rigid polymer for isolating oxygen and suppressing non-radiative decay. In addition, benefiting from the dual luminescent centers of localized emissions from DPPO homopolymer and charge transfer exciplex, color-tunable single-component OLPL polymers are also realized under different excitation wavelengths. Moreover, the single-component OLPL copolymer system disrupts the cell membrane of E. coli upon light irradiation, resulting in E. coli inactivation and showcasing an outstanding antibacterial performance. This study provides a simple molecular design strategy for the development of amorphous single-component exciplex-based OLPL materials, offering an important insight into the construction of highly stable OLPL materials and expansion of OLPL applications.
Data availability
All the necessary data to support the findings of this study can be found within the main text and ESI.†
Author contributions
H. L., H. D. and Y. T. conceived and designed the experiments. H. L., H. S. and S. Z. were primarily responsible for the experiments. H. L., C. T., C. C., H. L. and J. G. measured and analyzed the photophysical properties. X. Z., J. H. and G. X. performed the computational calculations. X. L. and H. D. performed the application of vitro antibacterial. H. L., H. D., R. C. and Y. T. wrote the manuscript and all authors contributed to the data analysis.
Conflicts of interest
There are no conflicts to declare.
Acknowledgements
This study was supported in part by the National Natural Science Foundation of China (22075149, 22322106, 62075102, 22305126, 22105104, 62288102 and 82301104), the Jiangsu Specially-Appointed Professor Plan, the China Postdoctoral Science Foundation (2023M731774), the Open Research Fund of Songshan Lake Materials Laboratory (2022SLABFN16), Natural Science Research Start-up Foundation of Recruiting Talents of Nanjing University of Posts and Telecommunications (grant no. NY222070), Project of State Key Laboratory of Organic Electronics and Information Displays, Nanjing University of Posts and Telecommunications (nos. GZR2023010014 and GDX2024010002), and the Hua Li Talents Program of Nanjing University of Posts and Telecommunications.
References
- R. Kabe and C. Adachi, Nature, 2017, 550, 384–387 CrossRef CAS PubMed.
- W. Zhao, Z. He and B. Z. Tang, Nat. Rev. Mater., 2020, 5, 869–885 CrossRef CAS.
- E. Hamzehpoor, C. Ruchlin, Y. Tao, C. Liu, H. M. Titi and D. F. Perepichka, Nat. Chem., 2022, 15, 83–90 CrossRef PubMed.
- H. Li, X. Xue, Y. Cao, H. Cheng, A. Luo, N. Guo, H. Li, G. Xie, Y. Tao, R. Chen and W. Huang, J. Am. Chem. Soc., 2023, 145, 7343–7351 CrossRef CAS PubMed.
- Y. Liang, C. Xu, H. Zhang, S. Wu, J. A. Li, Y. Yang, Z. Mao, S. Luo, C. Liu, G. Shi, F. Sun, Z. Chi and B. Xu, Angew. Chem., Int. Ed., 2023, 62, e202217616 CrossRef CAS PubMed.
- Y. Liang, M. Liu, T. Wang, J. Mao, L. Wang, D. Liu, T. Wang and W. Hu, Adv. Mater., 2023, 35, 2304820 CrossRef CAS PubMed.
- Y. Zhao, B. Ding, Z. Huang and X. Ma, Chem. Sci., 2022, 13, 8412–8416 RSC.
- W. Ye, H. Ma, H. Shi, H. Wang, A. Lv, L. Bian, M. Zhang, C. Ma, K. Ling, M. Gu, Y. Mao, X. Yao, C. Gao, K. Shen, W. Jia, J. Zhi, S. Cai, Z. Song, J. Li, Y. Zhang, S. Lu, K. Liu, C. Dong, Q. Wang, Y. Zhou, W. Yao, Y. Zhang, H. Zhang, Z. Zhang, X. Hang, Z. An, X. Liu and W. Huang, Nat. Mater., 2021, 20, 1539–1544 CrossRef CAS PubMed.
- Y. Zhang, L. Gao, X. Zheng, Z. Wang, C. Yang, H. Tang, L. Qu, Y. Li and Y. Zhao, Nat. Commun., 2021, 12, 2297 CrossRef CAS PubMed.
- Y. Miao, F. Lin, D. Guo, J. Chen, K. Zhang, T. Wu, H. Huang, Z. Chi and Z. Yang, Sci. Adv., 2024, 10, eadk3354 CrossRef CAS PubMed.
- C. Ji, L. Lai, P. Li, Z. Wu, W. Cheng and M. Yin, Aggregate, 2021, 2, e39 CrossRef CAS.
- B. Fang, L. Lai, M. Fan and M. Yin, J. Mater. Chem. C, 2021, 9, 11172–11179 RSC.
- Y. Tao, C. Liu, Y. Xiang, Z. Wang, X. Xue, P. Li, H. Li, G. Xie, W. Huang and R. Chen, J. Am. Chem. Soc., 2022, 144, 6946–6953 CrossRef CAS PubMed.
- H. Li, H. Li, J. Gu, F. He, H. Peng, Y. Tao, D. Tian, Q. Yang, P. Li, C. Zheng, W. Huang and R. Chen, Chem. Sci., 2021, 12, 3580–3586 RSC.
- Y. Wang, H. Gao, J. Yang, M. Fang, D. Ding, B. Z. Tang and Z. Li, Adv. Mater., 2021, 33, 2007811 CrossRef CAS PubMed.
- Y. Xia, C. Zhu, F. Cao, Y. Shen, M. Ouyang and Y. Zhang, Angew. Chem., Int. Ed., 2023, 62, e202217547 CrossRef CAS PubMed.
- F. Nie, K. Z. Wang and D. Yan, Nat. Commun., 2023, 14, 1654 CrossRef CAS PubMed.
- G. Yin, W. Lu, J. Huang, R. Li, D. Liu, L. Li, R. Zhou, G. Huo and T. Chen, Aggregate, 2023, 4, e344 CrossRef CAS.
- K. Jiang, Y. Wang, C. Lin, L. Zheng, J. Du, Y. Zhuang, R. Xie, Z. Li and H. Lin, Light Sci. Appl., 2022, 11, 80 CrossRef CAS PubMed.
- K. Jinnai, R. Kabe, Z. Lin and C. Adachi, Nat. Mater., 2022, 21, 338–344 CrossRef CAS PubMed.
- Z. Lin, R. Kabe, K. Wang and C. Adachi, Nat. Commun., 2020, 11, 191 CrossRef CAS PubMed.
- Y. Zhou, P. Zhang, Z. Liu, W. Yan, H. Gao, G. Liang and W. Qin, Adv. Mater., 2024, 2312439 CrossRef CAS PubMed.
- A. Cheng, H. Su, X. Gu, W. Zhang, B. Zhang, M. Zhou, J. Jiang, X. Zhang and G. Zhang, Angew. Chem., Int. Ed., 2023, 62, e202312627 CrossRef CAS PubMed.
- Y. Zhao, B. Ding, Z. Huang and X. Ma, Chem. Sci., 2022, 13, 8412–8416 RSC.
- W. Li, Z. Li, C. Si, M. Y. Wong, K. Jinnai, A. K. Gupta, R. Kabe, C. Adachi, W. Huang, E. Zysman-Colman and I. D. W. Samuel, Adv. Mater., 2020, 32, 2003911 CrossRef CAS PubMed.
- W. Qiu, X. Cai, M. Li, Z. Chen, L. Wang, W. Xie, K. Liu, M. Liu and S. Su, J. Phys. Chem. Lett., 2021, 12, 4600–4608 CrossRef CAS PubMed.
- P. Alam, T. S. Cheung, N. L. C. Leung, J. Zhang, J. Guo, L. Du, R. T. K. Kwok, J. W. Y. Lam, Z. Zeng, D. L. Phillips, H. H. Y. Sung, I. D. Williams and B. Z. Tang, J. Am. Chem. Soc., 2022, 144, 3050–3062 CrossRef CAS PubMed.
- S. Garain, S. M. Wagalgave, A. A. Kongasseri, B. C. Garain, S. N. Ansari, G. Sardar, D. Kabra, S. K. Pati and S. J. George, J. Am. Chem. Soc., 2022, 144, 10854–10861 CrossRef CAS PubMed.
- P. Alam, N. L. C. Leung, J. Liu, T. S. Cheung, X. Zhang, Z. He, R. T. K. Kwok, J. W. Y. Lam, H. H. Y. Sung, I. D. Williams, C. C. S. Chan, K. S. Wong, Q. Peng and B. Z. Tang, Adv. Mater., 2020, 32, 2001026 CrossRef CAS PubMed.
- S. Shao, J. Hu, X. Wang, L. Wang, X. Jing and F. Wang, J. Am. Chem. Soc., 2017, 139, 17739–17742 CrossRef CAS PubMed.
- J. Poisson, C. M. Tonge, N. R. Paisley, E. R. Sauvé, H. Mcmillan, S. V. Halldorson and Z. M. Hudson, Macromolecules, 2021, 54, 2466–2476 CrossRef CAS.
- C. M. Tonge and Z. M. Hudson, J. Am. Chem. Soc., 2019, 141, 13970–13976 CrossRef CAS PubMed.
- Y. Xin, Y. Zhu, R. Chi, C. Duan, P. Yan, C. Han and H. Xu, Adv. Mater., 2023, 35, 2304103 CrossRef CAS PubMed.
- J. Hu, Y. Wang, Q. Li, S. Shao, L. Wang, X. Jing and F. Wang, Chem. Sci., 2021, 12, 13083–13091 RSC.
- J. Hu, Q. Li, X. Wang, S. Shao, L. Wang, X. Jing and F. Wang, Angew. Chem., Int. Ed., 2019, 58, 8405–8409 CrossRef CAS PubMed.
- S. Ye, N. Meftahi, I. Lyskov, T. Tian, R. Whitfield, S. Kumar, A. J. Christofferson, D. A. Winkler, C. Shih, S. Russo, J. Leroux and Y. Bao, Chem, 2023, 9, 924–947 CAS.
- D. Zhong, X. Yang, X. Deng, X. Chen, Y. Sun, P. Tao, Z. Li, J. Zhang, G. Zhou and W. Wong, Chem. Eng. J., 2023, 452, 139480 CrossRef CAS.
- K. Jinnai, R. Kabe and C. Adachi, Adv. Mater., 2018, 30, 1800365 CrossRef PubMed.
- S. Kumar, L. G. Franca, K. Stavrou, E. Crovini, D. B. Cordes, A. M. Z. Slawin, A. P. Monkman and E. Zysman-Colman, J. Phys. Chem. Lett., 2021, 12, 2820–2830 CrossRef CAS PubMed.
- S. Yang, Y. Zhang, F. Kong, Y. Yu, H. Li, S. Zou, A. Khan, Z. Jiang and L. Liao, Chem. Eng. J., 2021, 418, 129366 CrossRef CAS.
- S. Tan, K. Jinnai, R. Kabe and C. Adachi, Adv. Mater., 2021, 33, 2008844 CrossRef CAS PubMed.
- Y. Shen, H. Liu, J. Cao, S. Zhang, W. Li and B. Yang, Phys. Chem. Chem. Phys., 2019, 21, 14511–14515 RSC.
- Z. Q. Feng, S. Y. Yang, F. C. Kong, Y. K. Qu, X. Y. Meng, Y. J. Yu, D. Y. Zhou, Z. Q. Jiang and L. S. Liao, Adv. Funct. Mater., 2023, 33, 2209708 CrossRef CAS.
- D. Barman, M. Annadhasan, A. P. Bidkar, P. Rajamalli, D. Barman, S. S. Ghosh, R. Chandrasekar and P. K. Iyer, Nat. Commun., 2023, 14, 6648 CrossRef CAS PubMed.
- X. Ban, T. Zhou, K. Zhang, Q. Cao, F. Ge, D. Zhang, P. Zhu, Z. Liu, Z. Li and W. Jiang, Chem. Eng. J., 2022, 441, 135898 CrossRef CAS.
- Y. Cai, W. Cheng, C. Ji, Z. Su and M. Yin, Dyes Pigm., 2021, 195, 109698 CrossRef CAS.
- X. Fu, X. Zhao, L. Chen, P. Ma, T. Liu and X. Yan, Biomater. Sci., 2023, 11, 5186–5194 RSC.
- L. Xu, K. Zhou, H. Ma, A. Lv, D. Pei, G. Li, Y. Zhang, Z. An, A. Li and G. He, ACS Appl. Mater. Interfaces, 2020, 12, 18385–18394 CrossRef CAS PubMed.
- Y. Miao, X. Zhang, J. Li, W. Yang, X. Huang and J. Lv, RSC Adv., 2022, 12, 20481–20491 RSC.
- C. Chao, L. Kang, W. Dai, C. Zhao, J. Shi, B. Tong, Z. Cai and Y. Dong, J. Mat. Chem. B, 2023, 11, 3106–3112 RSC.
|
This journal is © The Royal Society of Chemistry 2024 |
Click here to see how this site uses Cookies. View our privacy policy here.