DOI:
10.1039/D4SC03424D
(Edge Article)
Chem. Sci., 2024,
15, 17032-17040
Tumor oxygen microenvironment-tailored electron transfer-type photosensitizers for precise cancer therapy†
Received
25th May 2024
, Accepted 6th September 2024
First published on 7th September 2024
Abstract
The oxygen level in a tumor typically exhibits complex characteristics, ranging from mild hypoxia to moderate and even severe hypoxia. This poses significant challenges for the efficacy of photodynamic therapy, where oxygen is an essential element. Herein, we propose a novel therapeutic strategy and develop a series of lipid droplet-targeting photosensitive dyes (Ser-TPAs), i.e., in situ synergistic activation of two different electron transfer-type reactions. Based on this strategy, Ser-TPAs can synergistically generate O2˙− and nitrogen radicals regardless of the oxygen content, which results in a sustained high concentration of strongly oxidizing substances in the lipid droplets of cancer cells. As such, Ser-TPAs exhibited inhibitory activity against tumor growth in vivo, resulting in a significant reduction in tumor volume (Vexperimental group
:
Vcontrol group ≈ 0.07). This strategy offers a conceptual framework for the design of innovative photosensitive dyes that are suitable for cancer therapy in complex oxygen environments.
Introduction
Photodynamic therapy (PDT) has been recognized as a novel minimally invasive targeted approach for tumor treatment in clinical practice over recent years.1–3 PDT employs photosensitive dyes to initiate a series of photochemical reactions upon specific light irradiation,4,5 leading to the instantaneous formation of free radicals (O2˙−, ˙OH, etc.) or singlet oxygen (1O2) via electron transfer or energy transfer due to the different oxygen environments of the solid tumor lesion, respectively.6–8 Ultimately, this process induces the irreversible apoptosis of solid tumors. It is apparent that oxygen, light and photosensitive dyes are necessary elements for the PDT treatment of solid tumors. However, hypoxia is a common characteristic observed in solid tumors.9,10 Tumor lesions often exhibit mild (>2.5% O2) to moderate (1–2% O2) or even severe (<0.01% O2) hypoxia due to imbalances in solid tumor growth and the poorly organized vasculature.11 Moreover, the oxygen content in solid tumors is highly variable and can change significantly due to reduced internal oxygen supply with increasing tumor volume. Such hypoxia environments not only exacerbate the complexity of the tumor microenvironment but also seriously limit the therapeutic effect of PDT treatment strategies. Functioning as the core element of PDT, photosensitive dyes offer an effective approach toward addressing the challenges mentioned above, and developing photosensitive dyes that are capable of self-regulating different electron transfer-type reactions based on different levels of oxygen at tumor sites holds great promise for treating solid tumors with varying degrees of hypoxia.
In recent years, a range of targeting photosensitive dyes exhibiting excellent biocompatibility and metabolic capacity have been reported, some of which have been used for clinical photodynamic therapies,12–17 such as Photofrin, verteporfin, Foscan, etc. These dyes can generate singlet or free radical oxygen at tumor tissues upon irradiation with visible light (ca. 650 nm). However, despite their approval for cancer intervention, these photosensitive dyes still face significant limitations due to their dependence on oxygen. Consequently, researchers in this field are focusing on addressing the issue of oxygen dependency.18–31 A photosensitive dye (protoporphyrin IX, PPIX) coordinated with Cu(II) has been reported to enhance intersystem conversion from the singlet excited state to a triplet excited state via the stretching vibrations of N–H bonds.18 This enhancement effect successfully improves the production of 1O2 under mild hypoxia (>2.5% O2). Meanwhile, a mitochondria-targeting photosensitive dye (mitoaPS) with dual activation can generate 1O2 through combined classical PDT reactions (i.e. type-I and type-II), even under low oxygen conditions as low as 1% O2.19 However, these photosensitive dyes have limited efficacy in severe hypoxic tumors with oxygen content equal to or less than 0.01%. To overcome the oxygen dependence limitation of PDT, Peng and Kim developed a unimolecular photodynamic O2 economizer (PDOE) containing a mitochondria-targeted O2˙− generator (SORgenTAM). Therefore, using SORgenTAM, ensures the hypoxic environment can be overcome, enabling type-I PDT with significantly reduced dependence on oxygen levels (2%).20 Significantly, Peng and Fan reported the development of photosensitive dyes (NBEX) based on a new mechanism known as the type-III PDT reaction that does not require oxygen at all.21 When NBEX is excited by light, the excitation energy or electrons can be directly transferred to RNA to effectively destroy RNA and eliminate tumor cells even under hypoxic or anaerobic conditions. This research provides both conceptual insights and practical approaches for overcoming the limitations of phototherapeutics, while offering new design perspectives for photosensitive dyes under conditions of extremely low oxygen content (<0.01%). This previous research, which confirms that it is possible to ablate tumors using PDT even at low oxygen levels, provided us with the inspiration and motivation for the research described below.
Herein, we propose a novel strategy for a photosensitive dye (Fig. 1), that is, in situ synergistic activation of different electron transfer-type reactions based on the tumor oxygen microenvironments in the lesion area. Using this concept, a series of triphenylamine-based photosensitive dyes were designed and synthesized, including ((E)-4-(4-nitrostyryl)-N,N-diphenylaniline, NO2-TPA, (E)-4-(4-aminostyryl)-N,N-diphenylaniline, NH2-TPA, and (E)-N,N-diphenyl-4-styrylaniline, H-TPA), as depicted in Fig. 1. These photosensitive dyes should generate superoxide radical ions (O2˙−) until the oxygen concentration at the lesion sites decreases to < 0.01%, i.e. O2-type process. Subsequently, under severe hypoxic conditions (<0.01% O2), the excited singlet state of the photosensitive dye induces nitrogen radical formation through electron transfer facilitated by the tumor marker coenzyme upon light irradiation at the lesion sites (NAD+-type process). Consequently, these photosensitive dyes should exhibit remarkable efficacy against both hypoxic tumors and severely hypoxic tumors and display excellent performance in solid tumors with complex oxygen environments.
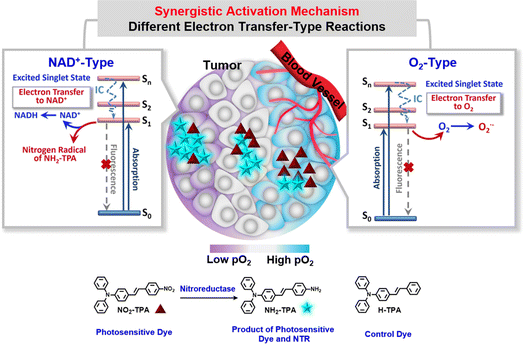 |
| Fig. 1 Chemical structures of compounds (NO2-TPA, NH2-TPA and H-TPA) and schematic diagram of the in situ synergistic activation mechanism for PDT activation. | |
Results
Molecular design and synthesis
To enhance photodynamic efficacy, this research provides an in situ synergistic activation strategy, i.e. the synergistic activation of two different electron transfer-type reactions according to different oxygen levels. One is an O2-type process and the other is a NAD+-type process (Fig. 1). Nitroreductase (NTR), a marker enzyme for hypoxic solid tumors, was selected as the initiator of the synergistic electron transfer-type reactions to enhance targeting of the photosensitive dye. Since NTR is overexpressed in hypoxic tumors and its expression is enhanced with an increase of the hypoxic degree,32,33 nitrobenzene, serving as the specific recognition group for NTR, was incorporated into the one end of the molecular skeleton (styryl) in the molecular design (see Fig. 1), while nicotinamide-adenine dinucleotide (NAD+), acting as a coenzyme facilitating electron transport of the photosensitive dye in living organisms,34 was chosen along with NTR to construct a complete system regulating photosensitive activity using two different electron transfer-type reactions. Importantly, the introduction of the nitrobenzene effectively inhibits the electron transfer from the photosensitive dye to biological substrates in non-tumor lesions under the influence of NAD+, which can significantly improve the targeting efficiency of photodynamic therapy. Furthermore, linking N,N-diphenylaniline to the other side of the styryl enhances the intramolecular charge-transfer (ICT) effect and provides potential for nitrogen radical formation under enzymatic regulation. A series of photosensitive dyes (Ser-TPAs, i.e. (E)-4-(4-nitrostyryl)-N,N-diphenylaniline, NO2-TPA, (E)-4-(4-aminostyryl)-N,N-diphenylaniline, NH2-TPA, and (E)-N,N-diphenyl-4-styrylaniline, H-TPA), were prepared and their molecular structures were confirmed by 1H NMR, 13C NMR, and Q-TOF-MS analytical data available in the ESI.†
Spectral behavior and mechanistic analysis in solution during the O2-type process
The spectral behavior was examined and analyzed using UV-vis absorption, two-photon absorption, fluorescence, phosphorescence, electron paramagnetic resonance (EPR) spectra and so on. Table S1† shows that the molar extinction coefficient (ε) of NO2-TPA is 2.66 × 104 M−1 cm−1 in H2O (λex = 456 nm). The two-photon absorption cross section of NO2-TPA was measured from 750 nm to 840 nm and exhibited a significant capacity for two-photon absorption within this range. A maximum absorption cross section of 394 GM was observed at 750 nm (1 GM = 10−50 cm4 s per photon, Fig. S1†), indicating its suitability for excitation by near-infrared light.35 And stability experiments indicate that NO2-TPA is suitable for use in physiological environments (Fig. S2†). These findings confirmed the fundamental molecular properties of the system for potential applications in PDT under long wavelength excitation.
In the free state, NO2-TPA (10 μM) exhibited a weak fluorescence quantum yield (Φflu = 0.27%, Table S1 and Fig. S3†) with an emission peak at 660 nm in H2O. Additionally, there was also negligible phosphorescence observed at 640 nm with extremely low intensity (Fig. S4†), which can be attributed to the quenching effect of –NO2. We calculated the electron hole separation of NO2-TPA and NH2-TPA, as shown in Fig. 2A and the electron and hole distance of NO2-TPA was 9.53 Å, which was much larger than the 1.51 Å of NH2-TPA. The greater the distance, the greater the energy loss for the NO2-TPA electron transition, and consequently the quenching of the fluorescence.36 The absence of intersystem crossing (ISC) from the excited singlet state (S1) to the excited triplet state (T1) was confirmed by theoretical calculations (ΔES1–T1 = 0.95 eV, Kisc (S1–T1) = 1.6 × 104 s−1 and Krisc (S1–T1) = 0.40 × 104 s−1, Table S2†). When 2′,7′-dichlorodihydrofluorescein (DCFH), a tracer agent for ROS, was used, no spectral changes were observed under light irradiation regardless of the presence or absence of oxygen (Fig. S5b†). Moreover, in the presence of NAD+, no signal enhancement of DCFH was observed in the NO2-TPA test system (Fig. S6†). EPR spectra also confirmed the absence of any signals corresponding to O2˙−, ˙OH, 1O2 and other reactive oxygen species (Fig. 2B, blue line and red line, Fig. S7†). These results indicate that NO2-TPA did not produce ROS including O2˙−, ˙OH, and 1O2, suggesting that traditional type-I or type-II PDT reactions do not occur, nor any new types of PDT reaction are initiated by NO2-TPA in the free state. This characteristic is expected to significantly enhance PDT targeting efficiency towards cancer cells.
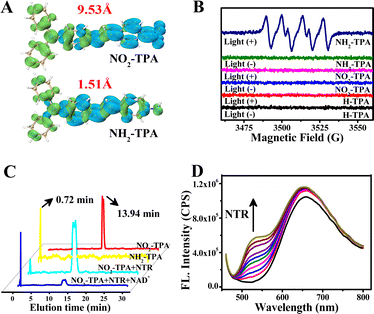 |
| Fig. 2 (A) Electron–hole distributions of NO2-TPA and NH2-TPA. The green regions represent electrons, and the blue regions represent holes. NO2-TPA has a larger distance dispersion of electrons and holes (9.53 Å) than NH2-TPA (1.51 Å), resulting in increased energy loss and lower fluorescence. (B) EPR spectra of NO2-TPA, NH2-TPA and H-TPA with or without light irradiation. The trapping agent was DMPO, and the test solution was methanol. Light (+): with light irradiation; light (−): without light irradiation. (C) NO2-TPA, NH2-TPA and the products of NO2-TPA reacting with NTR and NAD+ evaluated by HPLC. (D) Fluorescence response of NO2-TPA (10 μM) to NTR (0–4.67 μg mL−1) in H2O at 37 °C. | |
Upon encountering the tumor marker enzyme NTR,37,38NO2-TPA exhibits distinct and stable changes in its fluorescence spectra within 150 s (Fig. S8†). HPLC revealed that NO2-TPA (red line in Fig. 2C, 13.94 min) undergoes conversion to NH2-TPA (yellow line and blue line in Fig. 2C, 0.72 min) under the action of NTR, which can be triggered by a minimal overexpressed concentration of NTR (Fig. S9†). Furthermore, due to the changes in the molecular structure, the fluorescence intensity at 660 nm remains stable while the intensity at 525 nm gradually increases with increasing concentration of NTR (Fig. 2D). However, Φflu is still very low at 0.31% (see Table S1†). The phosphorescence is also observed to be extremely weak (Fig. S4†). We used EPR spectroscopy to further investigate the type of ROS generation during the O2-type process in the presence of oxygen. The EPR spectra exhibited a strong specific signal for O2˙− (Fig. 2B, navy blue line), but no signal for 1O2 (Fig. S7B†). The fluorescence spectra with different tracer agents for different kinds of ROS were used to detect this process (Fig. S5†). The above results confirmed that O2˙− is indeed generated by NH2-TPA, the product of NO2-TPA in an O2-type process, which occurs when the oxygen content is >0.01%.
To elucidate the mechanism of the O2-type process, transient absorption spectroscopy was used to determine the T1 state of NO2-TPA, NH2-TPA and the contrastive molecule H-TPA (Fig. S11†). The spectra reveal the absence of the T1 state for both NO2-TPA and H-TPA, while the T1 signal in NH2-TPA was found to be negligible. Thus, there is no ISC from S1 to T1 in NO2-TPA, NH2-TPA and H-TPA, which can be further supported by the theoretical calculations (see Table S2†). To further confirm the mechanism of O2˙− generation, we determined the electron transfer capacity of NO2-TPA and NH2-TPA in their respective S1 states as well as their oxidation potentials. Upon addition of 7,7,8,8-tetracyanoquinodimethane (TCNQ) at a concentration of 40 μM as an electron acceptor into each solution, respectively, a significant decrease in the fluorescence spectra of NH2-TPA was observed, but no such phenomenon was observed for the fluorescence spectra of NO2-TPA (Fig. S12†). In other words, only NH2-TPA can transfer electrons to O2 from the S1 state (O2-type process). The above processes are all completed with normal oxygen levels. However, the oxygen levels inside the tumors are complicated and it is necessary to investigate how the electron transfer reaction works at various oxygen levels.
Spectral behavior and mechanistic analysis in solution during the NAD+-type process
The intricate oxygen environment is a prominent feature in malignant tumors. The levels of NTR and NAD+ exhibit a significant increase when the content of O2 drops to as low as 0.01% (severe hypoxia, NAD+-type process). Notably, the electron transfer ability of NAD+ is greatly enhanced in situ.39–41 From Fig. 3A, a gradual decrease in fluorescence intensity at 656 nm is accompanied by an incremental enhancement in intensity at 540 nm with increasing concentrations of NTR and NAD+. However, despite this observation, the fluorescence intensity remains relatively low with a Φ value of only 0.52%, and no phosphorescence was detected (Table S1†). The presence of electron holes was detected by EPR spectroscopy (Fig. S7C and D†), which verified the ability of the NAD+-type process to generate nitrogen radical signals under hypoxic conditions. Subsequently, triphenylamine nitrogen centered radicals produced by the photochemical reaction of NH2-TPA and NAD+ were detected. The results are presented in Fig. 3B, and the EPR spectra displayed a three-line splitting pattern consistent with that of the triphenylamine nitrogen radical.42 All the above results confirmed that a novel reaction generating a nitrogen radical occurred under electron transfer mediated by NAD+ at extremely low oxygen levels (<0.01%).
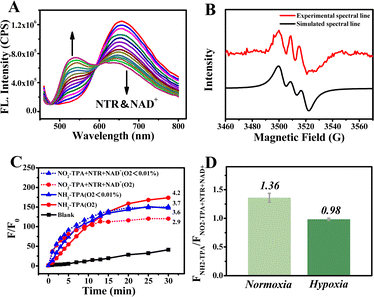 |
| Fig. 3 (A) Fluorescence response of NO2-TPA (10 μM) to both NTR (0–4.67 μg mL−1) and NAD+ (66.7 μM) in H2O. All tests were carried out at 37 °C. (B) EPR spectra of the nitrogen centered radical for the photochemical reaction between NH2-TPA and NAD+ (experimental: red line; simulated: black line). (C) Relative changes in the fluorescence intensity of DHR123 (40 μM) an indicator for O2˙− measured when NH2-TPA (10 μM), NO2-TPA (10 μM), NTR and NAD+ were subjected to light irradiation. Note: because the wavelength of the O2˙−-specific recognition probe DHE and the photosensitive dye involved in this paper interfere with each other, DHR123 was selected as an alternative probe to carry out the test. (D) Bar plots for the ratio of ROS generated by NH2-TPA relative to NO2-TPA reacting with NTR and NAD+ under normoxic and hypoxic conditions. | |
To characterize nitrogen radical production for the different oxygen levels, the fluorescence spectra were used during the NAD+-type process with dihydrorhodamine 123 (DHR123) as an indicator for free radicals.43 Due to varying NTR activity under different oxygen conditions, the amount of NH2-TPA produced is different, so there will be a different PDT effect. As such, Fig. 3C and D display signal enhancements with different oxygen levels and even at oxygen levels <0.01%. Fig. S5† also shows the same results using DCFH as an indicator for ROS including nitrogen radicals. These above results indicated that the NAD+-type process was initiated during the process of oxygen deprivation and could generate nitrogen-radical species for continuous photodynamic therapy.
To investigate the reaction mechanism in the second process (O2 <0.01%), the electron binding capacities of NO2-TPA, H-TPA and NH2-TPA were calculated by molecular docking and theoretical calculations (Fig. 4). The results are depicted in Fig. 4A and indicate significant changes in the predominant conformations of these molecules. After binding with NAD+, NH2-TPA exposes the single electron pair from N more extensively than before. The electron cloud density on the nitrogen atom of triphenylamine of NO2-TPA, H-TPA and NH2-TPA in the S1 state is −0.061, −0.093 and −0.083, respectively (Fig. 4B), indicating an increasing trend in the electron donating abilities: NO2-TPA < H-TPA < NH2-TPA. This observation is further supported by the REDOX potential of the S1 state (Table S3†). Consequently, NH2-TPA exhibits a higher propensity to form the nitrogen radical by donating electrons from its N atom compared to other compounds such as NO2-TPA, which does not exhibit this behavior in the S1 state. Therefore, the NAD+-type process mainly involves an electron transfer-type reaction between NH2-TPA and NAD+, leading to the generation of nitrogen radical signals specifically within severely hypoxic tumors (<1% O2).
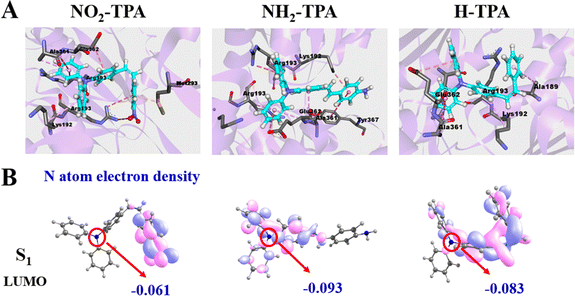 |
| Fig. 4 (A) Molecular docking diagrams of NO2-TPA, NH2-TPA and H-TPA with NAD+ respectively. (B) Molecular calculations and electron density on the nitrogen atom for optimal configurations of NO2-TPA, NH2-TPA, and H-TPA binding to NAD+. | |
Synergistic mechanism of two electron transfer-type reactions (O2-type and NAD+-type processes)
It is well known that organisms have extraordinarily complex microenvironments, notably at the location of lesions, where the concentrations of O2, NTR and NAD+ are highly variable. Activating either the O2-type process or NAD+-type process in this environment. Therefore, it is important to use both the O2-type and NAD+-type processes in concert in a biological environment. REDOX potentials were used to confirm the occurrence of these two processes in living organisms. The REDOX potentials of O2/O2˙− and NAD+/NADH are −0.33 V and −0.32 V, respectively, vs. standard electrode potential (Fig. S13†).44,45 Meanwhile, the excited singlet state of NH2-TPA had a REDOX potential of −2.60 V (Fig. S13†). So, NH2-TPA could react with O2 to form O2˙−via the O2-type process and with NAD+ to form nitrogen radicals via the NAD+-type process. Significantly, the two processes can occur in concert in living organisms. Throughout the synergistic process, O2 will be consumed until it is exhausted. Therefore, by using the synergistic effect of both the O2-type process and the NAD+-type process, the progression and metastasis of the tumor will be significantly reduced.
Application and analysis in living cells
Given the synergistic activation mechanism of such photosensitive dyes, the HepG-2 cell line was selected to explore possible applications and the mechanism in living cells. Firstly, the intracellular distribution was investigated. We calculated log
P values for the molecules NO2-TPA and NH2-TPA, which are 6.8 and 5.9, respectively. NO2-TPA is highly lipophilic based on quantitative structure–activity relationship analysis and was expected to accumulate in lipid droplets due to its membrane permeability.46 At the same time, Fig. S14† reveals that NO2-TPA was predominantly localized in a small organelle known as a lipid droplet (diameter as small as 40 nm), which plays crucial roles in lipid metabolism and storage, membrane transport, and protein degradation, and has become an important target for cancer therapy.47,48 These characteristics enable NO2-TPA to rapidly generate high concentrations of strong oxidizing substances (such as O2˙−, nitrogen radicals, etc.) in the intracellular region. Consequently, these two different electron transfer-type reaction processes can enhance efficacy toward cancer cells.49
Subsequently, different oxygen contents were modeled in HepG-2 cells to further validate the synergetic function of O2-type and NAD+-type reaction processes. DCFH-DA, DHR123 and Singlet Oxygen Sensor Green (SOSG, a fluorescent indicator for 1O2) were added into three cell models with different oxygen contents (i.e., 21%, 2.5% and <0.01%). Under a normal oxygen environment (21% oxygen content), an apparent fluorescence intensity was observed upon incubation of DHR123 (Fig. 5A) after 3 minutes of light irradiation in cancer cells expressing high levels of NTR. Moreover, the fluorescence intensity decreased as the oxygen content decreased (2.5% and <0.01%), and DCFH-DA exhibited a slight increase in fluorescence intensity for the different cell models with varying oxygen content (Fig. 5B). Simultaneously, no signal for 1O2 was detected regardless of the amount of O2 (Fig. 5C). These results obtained from both solution-based experiments and living cells collectively confirm that as the O2 content decreases, there is a concomitant decrease in the generation of O2˙−, while an increase in the generated amount of nitrogen radical occurs. This highlights an intelligent synergistic activation mechanism involving a gradual throttling of the O2-type reaction process (Fig. 1, O2-type) along with the activation of the NAD+-type reaction process (Fig. 1, NAD+-type) upon decreasing oxygen levels. A methyl thiazolyl tetrazolium (MTT) colorimetric assay was used to verify the targeted killing ability toward cancer cells. The results indicated that NO2-TPA (5 μM) exhibited significant cytotoxicity (21% O2: 59% death rate, 2.5% O2: 54% death rate and 0% O2: 41% death rate) specifically toward HepG-2 cells under irradiation. Compared with similar photosensitive dyes reported previously, NO2-TPA exhibited potent cell phototoxicity as well as good cancer cell targeting ability (Table S4†). Additionally, NO2-TPA effectively inhibited cell proliferation in a concentration-dependent manner as evidenced by its IC50 value for HepG-2 cells being determined to be 4.3 μM (Fig. S15†).
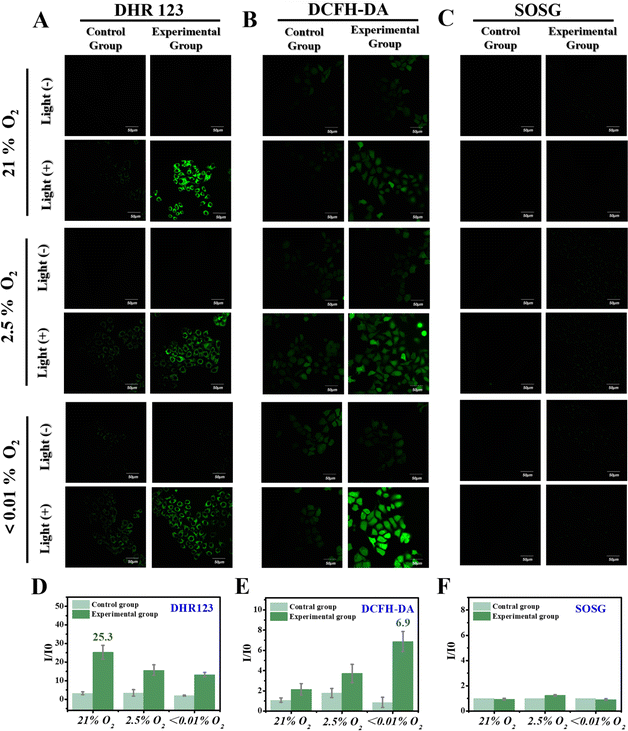 |
| Fig. 5 The O2˙− ((A) DHR 123, 6 μM, Ex: 488 nm, and green channel: 500–550 nm), ROS ((B) DCFH-DA, 6 μM, Ex: 488 nm, and green channel: 500–550 nm) and 1O2 ((C) SOSG, 5 μM, Ex: 488 nm, and green channel: 500–550 nm) generation ability of NO2-TPA (6 μM) in HepG-2 cells with different oxygen levels. The irradiation time was 3 min. Scale bars: 50 μm. (D–F) Fluorescence histogram of DHR123, DCFH-DA and SOSG at < 0.01%, 2.5% and 21% O2 concentrations. | |
To further elucidate the underlying cause of the elevated mortality rate, a mitochondrial membrane potential assay was performed with HepG-2 cells, since it represents an early event in the apoptosis cascade reaction.50 The imaging results (Fig. S16†) revealed a gradual decrease in fluorescence intensity in the red channel (580–620 nm), accompanied by a corresponding increase in fluorescence intensity in the green channel (500–550 nm) during 0–20 min of irradiation. These findings indicate a reduction in mitochondrial membrane potential and subsequent initiation of programmed cell death. High-throughput flow cytometric analysis confirmed a significant increase in apoptotic cell population upon incubation with NO2-TPA and NH2-TPA under irradiation (Fig. S17†).
In vivo applications
The feasibility of NO2-TPA for tumor treatment in mice was further investigated. Various levels of oxygen content in solid tumors (ranging from normal oxygen to mild, moderate, and severe hypoxia) were validated for their photodynamic therapeutic effect. Fig. S18† indicates that a wide range of fluorescence intensity changes were observed when the tumor tissue section was incubated with [Ru(dpp)3]Cl2 as an oxygen-indicating probe, confirming the highly intricate nature of O2 content in solid tumors.51 The in vivo performance of NO2-TPA was evaluated using tumor-bearing mice and a Night OWL II LB 983 imaging system when the solid tumors reached approximately 120 mm3. Fig. S19† illustrates the detection of distinct fluorescence at 530–570 nm following intratumoral injection of NO2-TPA. Over time, the fluorescence intensity gradually increased and peaked at 30 minutes post-injection, suggesting that this time point would be optimal for subsequent PDT.
For the PDT experiments, we chose NU/NU tumor-bearing mice. Tumors were treated with NO2-TPA/NH2-TPA by injection and light irradiation was performed on days 1, 2, 3, and 5, and the tumor was dissected after the tenth day of feeding. As shown in Fig. 6A, compared to the NO2-TPA/NH2-TPA group, the experimental group (NO2-TPA/NH2-TPA + light irradiation group) exhibited significant ablation. Encouraged by these results, in order to better verify the universality and effectiveness of the photosensitizer, we used normal strain KM mice to replace immunodeficient mice NU/NU, and extended the feeding time used for the subsequent experiments. The tumors were treated by injecting with NO2-TPA and then subjecting them to light irradiation on days 1, 4, 7, 10 and 13. Fig. 6B(III) indicates that the tumor volume ratio (V/V0) in the control group and blank group was 9.4 (control group), 11.7 (NO2-TPA group) and 9.7 (light irradiation group). Remarkably, the experimental group (NO2-TPA + light irradiation group, the treatment period = 22 days) exhibited a remarkable tumor regression effect with a Vexperimental group/Vcontrol group of only 0.07. The tumor suppression effects of the control groups, blank groups and experimental groups were further analyzed using hematoxylin–eosin staining (H&E). Fig. 6B(IV) indicates significant cell apoptosis in the experimental groups (NO2-TPA + light irradiation group), while extensive cell damage was not observed in the other groups. Furthermore, no abnormal changes in body weight were observed during treatment for any of the mice (Fig. 6B(II)). Additionally, there were no noticeable signs of cell necrosis or inflamed lesions in the liver and kidneys of the experimental group (Fig. 6B(V)), highlighting the biocompatibility and applicability of NO2-TPA toward in vivo systems.
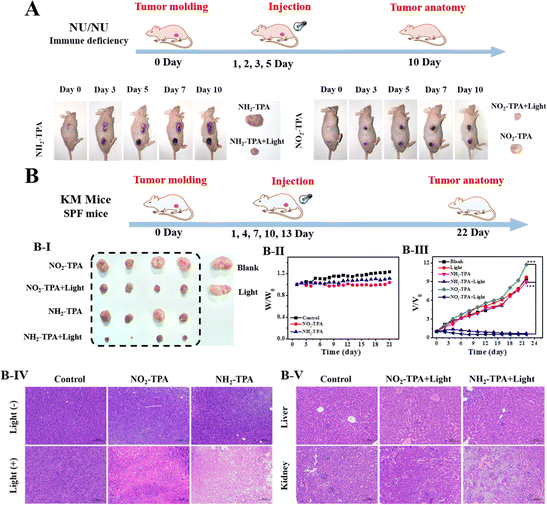 |
| Fig. 6 (A) Photodynamic therapeutic efficiency of NO2-TPA and NH2-TPA in S180 tumor-bearing NU/NU mice. On days 1, 4 and 7, NO2-TPA/NH2-TPA (1 mM, 20 μL) and light were delivered, and photographs were collected during the 10 days of monitoring. (B) Photodynamic therapeutic efficiency of NO2-TPA and NH2-TPA toward S180 tumor-bearing KM mice. NO2-TPA/NH2-TPA (1 mM, 20 μL) and light were applied on days 1, 4, 7, 10 and 13. Tumor volumes were measured one day apart and mice were weighed daily. Tumors were stripped after 22 days to obtain the photographs. (B(I)) Images of tumors harvested in mice from different groups after 22 days of treatment. (B(II)) Tumor volume changes in KM mice subjected to various treatments. (B(III)) Body weight changes of KM mice subjected to various treatments. ***(P < 0.001). (B(IV and V)) H&E staining analysis of tumor tissues, liver and kidneys following various therapies. The liver and kidney experimental groups were from mice treated by injection and illumination, and the control group was from mice without any treatment. Scale bars: 100 μm. | |
Conclusions
In summary, to overcome the limitations imposed on the efficacy of PDT by the complex oxygen environment in solid tumors, we have developed a series of novel styryl molecular skeleton-based photosensitive dyes (Ser-TPAs). The presence of specific recognition groups attached to ends of the molecular skeleton enables synergistic activation of two different electron transfer-type reactions at varying oxygen levels at tumor lesions. Under normoxic conditions, electron transfer between Ser-TPAs and oxygen molecules via an O2-type process generates O2˙−, while electron transfer between Ser-TPAs and NAD+ through a NAD+-type process results in the formation of a nitrogen radical. Consequently, as oxygen is depleted and extreme hypoxia prevails in the tumor lesion, there is a gradual decrease in the efficiency of the O2-type process mediated by Ser-TPAs, whereas the enhancement of the NAD+-type process takes place. Electron transfer occurs between Ser-TPA and NAD+, a biological enzyme, resulting in the generation of a nitrogen radical with strong oxidation capabilities, which increases with higher levels of hypoxia. The synergistic interaction between the nitrogen radicals and O2˙− allows for continuous production of high concentrations of potent oxidizing substances by Ser-TPAs in the tumor lesion, thereby maintaining an excellent photodynamic therapeutic effect and achieving effective tumor photoablation. Furthermore, Ser-TPAs exhibit remarkable tumor targeting ability as they are exclusively activated in cancer cells. These findings emphasize the potential of Ser-TPAs as a valuable tool for hypoxic tumor therapy while providing new perspectives for the design and development of novel photosensitive dyes to treat complex cancers using co-activation strategies involving both electron transfer-type reactions.
Data availability
The authors confirm that the data supporting the findings of this study are available within the article [and/or its ESI†].
Author contributions
Y. T. Y., J. Y. Y. and H. Z. proposed the concept and supervised the work; Y. T. Y. performed the synthesis of compounds and participated in all experimental processes. Y. F. W. and G. W. involved in mouse tumor model construction and in vivo testing. Y. L., K. W. and Y. G. Y. performed quantitative theoretical calculations. Y. T. Y., W. J., T. D. J., J. Y. Y. and H. Z. contributed to the discussion and provided suggestions. Y. T. Y. and H. Z. wrote the manuscript. All authors discussed the results and commented on the manuscript.
Conflicts of interest
The authors declare no competing interests.
Acknowledgements
This work was supported by the National Natural Science Foundation of China (U21A20314, 22107089, 11974103, and 22378100); T. D. J. wishes to thank the University of Bath and the Open Research Fund of the School of Chemistry and Chemical Engineering, Henan Normal University (2020ZD01) for support. T. D. J. has been appointed as an Outstanding Talent by Henan Normal University. The work was supported by the Program for Innovative Research Team in Science and Technology at the University of Henan Province (23IRTSTHN002). J. Y. and W. J. J. thank the National Research Foundation of Korea for the grant funded by the Korean government (MSIT) (RS-2023-00217701). The Henan Province Central Leading Local Science and Technology Development Fund Project (Z20231811083) and the Central Government of Henan Province Guides Local Projects are acknowledged. All authors express their gratitude to Dr C. C. Shen for his invaluable assistance in this study.
Notes and references
- L. Zhao, J. Li, Y. Su, L. Yang, L. Chen, L. Qiang, Y. Wang, H. Xiang, H. P. Tham, J. Peng and Y. Zhao, Science Advances, 2020, 6, eaaz0575 CrossRef
.
- C. Lu, Z. Li, N. Wu, D. Lu, X.-B. Zhang and G. Song, Chem, 2023, 9, 3185–3211 Search PubMed
.
- T. C. Pham, V. N. Nguyen, Y. Choi, S. Lee and J. Yoon, Chem. Rev., 2021, 121, 13454–13619 CrossRef PubMed
.
- J. A. Figueira and V. C. Veltrini, Photodiagn. Photodyn. Ther., 2017, 20, 125–129 CrossRef
.
- C. Hopper, Lancet Oncol., 2000, 1, 212–219 CrossRef
.
- L. O. Svaasand and R. Ellingsen, Photochem. Photobiol., 1983, 38, 293–299 CrossRef PubMed
.
- D. Mao, W. Wu, S. Ji, C. Chen, F. Hu, D. Kong, D. Ding and B. Liu, Chem, 2017, 3, 991–1007 Search PubMed
.
- G. O. Schenck, Ind. Eng. Chem., 1963, 55, 40–43 CrossRef
.
- J. M. Brown, Mol. Med. Today, 2000, 6, 157–162 CrossRef
.
- D. Hanahan and R. A. Weinberg, Cell, 2011, 144, 646–674 CrossRef PubMed
.
- E. Hammond, M. C. Asselin, D. Forster, J. O'Connor, J. M. Senra and K. J. Williams, Clin. Oncol., 2014, 26, 277–288 CrossRef PubMed
.
- M. R. Detty, S. L. Gibson and S. J. Wagner, J. Med. Chem., 2004, 47, 3897–3915 CrossRef PubMed
.
- A. Juarranz, P. Jaén, F. Sanz-Rodríguez, J. Cuevas and S. González, Clin. Transl. Oncol., 2008, 10, 148–154 CrossRef PubMed
.
- E. H. C. van Dijk, T. J. van Rijssen, Y. Subhi and C. J. F. Boon, Ophthalmol. Ther., 2020, 9, 329–342 CrossRef PubMed
.
- Y. Liu, L. Scrivano, J. D. Peterson, M. Fens, I. B. Hernández, B. Mesquita, J. S. Toraño, W. E. Hennink, C. F. van Nostrum and S. Oliveira, Mol. Pharm., 2020, 17, 1276–1292 CrossRef CAS PubMed
.
- K. Li, W. Dong, L. Qiu, Q. Liu, G. Lv, Y. Peng, M. Xie and J. Lin, Eur. J. Med. Chem., 2019, 181, 111582 CrossRef CAS PubMed
.
- H. Gu, W. Liu, W. Sun, J. Du, J. Fan and X. Peng, Chem. Sci., 2022, 13, 9719–9726 RSC
.
- J. M. Beames, A. J. Hudson, T. D. Vaden and J. P. Simons, Phys. Chem. Chem. Phys., 2010, 12, 14076–14081 RSC
.
- J. Sun, K. Du, J. Diao, X. Cai, F. Feng and S. Wang, Angew Chem. Int. Ed. Engl., 2020, 59, 12122–12128 CrossRef PubMed
.
- M. Li, Y. Shao, J. H. Kim, Z. Pu, X. Zhao, H. Huang, T. Xiong, Y. Kang, G. Li, K. Shao, J. Fan, J. W. Foley, J. S. Kim and X. Peng, J. Am. Chem. Soc., 2020, 142, 5380–5388 CrossRef PubMed
.
- Q. Yao, J. Fan, S. Long, X. Zhao, H. Li, J. Du, K. Shao and X. Peng, Chem, 2021, 8, 197–209 Search PubMed
.
- Y. Y. Zhao, L. Zhang, Z. Chen, B. Y. Zheng, M. Ke, X. Li and J. D. Huang, J. Am. Chem. Soc., 2021, 143, 13980–13989 CrossRef PubMed
.
- L. Li, C. Shao, T. Liu, Z. Chao, H. Chen, F. Xiao, H. He, Z. Wei, Y. Zhu, H. Wang, X. Zhang, Y. Wen, B. Yang, F. He and L. Tian, Adv. Mater., 2020, 32, e2003471 CrossRef PubMed
.
- L. Zuo, W. Nie, S. Yu, W. Zhuang, G. Wu, H. Liu, L. Huang, D. Shi, X. Sui, Y. Li and H. Y. Xie, Angew Chem. Int. Ed. Engl., 2021, 60, 25365–25371 CrossRef PubMed
.
- V. N. Nguyen, Y. Yan, J. Zhao and J. Yoon, Acc. Chem. Res., 2021, 54, 207–220 Search PubMed
.
- Y. Wang, S. Xu, L. Shi, C. Teh, G. Qi and B. Liu, Angew Chem. Int. Ed. Engl., 2021, 60, 14945–14953 CrossRef
.
- M. Wu, X. Liu, H. Chen, Y. Duan, J. Liu, Y. Pan and B. Liu, Angew Chem. Int. Ed. Engl., 2021, 60, 9093–9098 CrossRef
.
- P. Xiao, Z. Shen, D. Wang, Y. Pan, Y. Li, J. Gong, L. Wang, D. Wang and B. Z. Tang, Adv. Sci., 2022, 9, e2104079 CrossRef PubMed
.
- X. Zhao, J. Liu, J. Fan, H. Chao and X. Peng, Chem. Soc. Rev., 2021, 50, 4185–4219 RSC
.
- V. N. Nguyen, S. Qi, S. Kim, N. Kwon, G. Kim, Y. Yim, S. Park and J. Yoon, J. Am. Chem. Soc., 2019, 141, 16243–16248 CrossRef PubMed
.
- W. Zhou, S. Chen, Y. Ouyang, B. Huang, H. Zhang, W. Zhang and J. Tian, Chem. Sci., 2023, 14, 11481–11489 RSC
.
- A. Chevalier, Y. Zhang, O. M. Khdour, J. B. Kaye and S. M. Hecht, J. Am. Chem. Soc., 2016, 138, 12009–12012 CrossRef CAS PubMed
.
- Y. Li, Y. Sun, J. Li, Q. Su, W. Yuan, Y. Dai, C. Han, Q. Wang, W. Feng and F. Li, J. Am. Chem. Soc., 2015, 137, 6407–6416 CrossRef CAS PubMed
.
- A. Podder, V. P. Murali, S. Deepika, A. Dhamija, S. Biswas, K. K. Maiti and S. Bhuniya, Anal. Chem., 2020, 92, 12356–12362 CrossRef CAS
.
- B. K. Kundu, G. Han and Y. Sun, J. Am. Chem. Soc., 2023, 145, 3535–3542 CrossRef CAS PubMed
.
- N. Zhang, Y. Wang, S. Leng, S. Xu, L. Zhang, Q. Wang, Q. Zhang and H. Y. Hu, Talanta, 2019, 205, 120133 CrossRef CAS
.
- H. W. Liu, L. Chen, C. Xu, Z. Li, H. Zhang, X. B. Zhang and W. Tan, Chem. Soc. Rev., 2018, 47, 7140–7180 RSC
.
- X. Zhao, S. Long, M. Li, J. Cao, Y. Li, L. Guo, W. Sun, J. Du, J. Fan and X. Peng, J. Am. Chem. Soc., 2020, 142, 1510–1517 CrossRef CAS
.
- A. Garten, S. Schuster, M. Penke, T. Gorski, T. de Giorgis and W. Kiess, Nat. Rev. Endocrinol., 2015, 11, 535–546 CrossRef PubMed
.
- H. Lv, G. Lv, C. Chen, Q. Zong, G. Jiang, D. Ye, X. Cui, Y. He, W. Xiang, Q. Han, L. Tang, W. Yang and H. Wang, Cell Metab., 2021, 33, 110–127 CrossRef
.
- T. Nacarelli, L. Lau, T. Fukumoto, J. Zundell, N. Fatkhutdinov, S. Wu, K. M. Aird, O. Iwasaki, A. V. Kossenkov, D. Schultz, K. I. Noma, J. A. Baur, Z. Schug, H. Y. Tang, D. W. Speicher, G. David and R. Zhang, Nat. Cell Biol., 2019, 21, 397–407 CrossRef
.
- X. Li, Y. L. Wang, C. Chen and Y. F. Han, Chem.–Eur. J., 2023, 29, e202203242 CrossRef PubMed
.
- Q. Wan, R. Zhang, Z. Zhuang, Y. Li, Y. Huang, Z. Wang, W. Zhang, J. Hou and B. Tang, Adv. Funct. Mater., 2020, 30, 2002057 CrossRef
.
- Y. A. Ilan, G. Czapski and D. Meisel, Biochim. Biophys. Acta, 1976, 430, 209–224 CrossRef PubMed
.
-
M. Berlanga, Brock Biology of Microorganisms, Int. Microbiol., ed. M. T. Madigan and J. M. Martinko, 11th edn, Núm. 2, 2005, 2010, vol. 8, pp. 149–150 Search PubMed
.
- S. Uchinomiya, R. Horobin, E. Alvarado-Martínez, E. Peña-Cabrera and Y.-T. Chang, Comb. Chem. High Throughput Screening, 2016, 19, 378–383 CrossRef PubMed
.
- S. Pillai, I. Mahmud, R. Mahar, C. Griffith, M. Langsen, J. Nguyen, J. W. Wojtkowiak, P. Swietach, R. A. Gatenby, M. M. Bui, M. E. Merritt, P. McDonald, T. J. Garrett and R. J. Gillies, Cell Rep., 2022, 39, 110796 CrossRef PubMed
.
- A. R. Thiam and E. Ikonen, Trends Cell Biol., 2021, 31, 108–118 CrossRef PubMed
.
- T. Liang, D. Wen, G. Chen, A. Chan, Z. Chen, H. Li, Z. Wang, X. Han, L. Jiang, J. J. Zhu and Z. Gu, Adv. Mater., 2021, 33, e2100629 CrossRef PubMed
.
- B. R. Gastman, X. M. Yin, D. E. Johnson, E. Wieckowski, G. Q. Wang, S. C. Watkins and H. Rabinowich, Cancer Res., 2000, 60, 6811–6817 Search PubMed
.
- J. A. Bertout, S. A. Patel and M. C. Simon, Nat. Rev. Cancer, 2008, 8, 967–975 CrossRef PubMed
.
|
This journal is © The Royal Society of Chemistry 2024 |
Click here to see how this site uses Cookies. View our privacy policy here.