DOI:
10.1039/D4SC03428G
(Review Article)
Chem. Sci., 2024,
15, 15983-16005
2D compounds with heterolayered architecture for infrared photodetectors
Received
25th May 2024
, Accepted 7th September 2024
First published on 9th September 2024
Abstract
Compounds with heterolayered architecture, as a family of two-dimensional (2D) materials, are composed of alternating positive and negative layers. Their physical properties are determined not only by the charged constituents, but also by the interaction between the two layers. This kind of material has been widely used for superconductivity, thermoelectricity, energy storage, etc. In recent years, heterolayered compounds have been found as an encouraging choice for infrared photodetectors with high sensitivity, fast response, and remarkable reliability. In this review, we summarize the research progress of heterolayered materials for infrared photodetectors. A simple development history of the materials with three-dimensional (3D) or 2D structures, which are suitable for infrared photodetectors, is introduced firstly. Then, we compare the differences between van der Waals layered 2D materials and heterolayered 2D cousins and explain the advantages of heterolayered 2D compounds. Finally, we present our perspective on the future direction of heterolayered 2D materials as an emerging class of materials for infrared photodetectors.
1. Introduction
Infrared detectors, which are used to detect and measure infrared radiation, have been widely used for military, security, environmental monitoring and industrial applications.1–4 They can be divided into infrared thermal detectors and infrared photodetectors. Infrared thermal detectors are based on thermocouples, which are composed of two different semiconductors or conductors. Under infrared light irradiation, these two kinds of materials have different temperatures, which leads to the movement of electrons inside the thermocouple and the creation of an electric potential.5,6 For infrared photodetectors, the working mechanism is to convert the optical signals to the electrical ones based on the photovoltaic effect or photoconductive effect.7,8 Compared with infrared thermal detectors, infrared photodetectors have the advantages of higher sensitivity, faster response, broader wavelength range response and higher reliability.9 For infrared photodetectors, the core lies in the properties of the materials which basically have high sensitivity to infrared light.
Two-dimensional (2D) materials have been identified as an intriguing large family for infrared photodetectors, and the discovery of single-layer graphene in 2004 sparked interest in the application of 2D materials for infrared photodetectors.10 Compared with 3D materials, 2D materials generally exhibit higher photoelectric conversion efficiency and sensitivity, which allows 2D materials to respond more accurately and quickly when being used to detect infrared light signals. Due to the unique layered structure of 2D materials, electrons and holes move quickly in the 2D plane, and infrared photodetectors based on 2D materials usually have shorter response times and are able to detect changes in infrared light signals more quickly.11,12 Moreover, 2D materials often have a broad spectral response range, and some 2D materials even perform well in the ultraviolet (UV), visible (VIS), and terahertz (THz) bands, making them suitable for a broader range of applications. Black phosphorus has similar properties to graphene, with a broad absorption spectrum and high carrier mobility.13,14 In recent years, transition metal chalcogenides, such as MoS2 and WS2, have shown potential for photodetection due to their high light absorption coefficients and tunable bandgaps.15,16 However, one coin always has two sides. Different from their 3D counterparts, 2D materials also have some problems, such as high anisotropy and complex production processes.17–20
Heterolayered materials refer to 2D materials that are bonded by ionic or covalent interactions between the constituents.21 The crystal structures of these kinds of 2D compounds are composed of alternating positive and negative layers. Multiple choices of the positive or negative layers contribute to the large quantity of heterolayered compounds. Usually, either a positive or negative constituent determines the physical properties of the heterolayered compounds, in which the other layer stabilizes the whole structure. Besides, the bonding between the two layers also contributes to the properties of the compounds. Hereby, the unique heterolayered structures endow them with the properties of 3D bulk materials and the superiority of 2D geometric structures, marking them particularly suitable for the field of infrared photodetection. Up to now, several heterolayered 2D compounds have been reported for infrared photodetectors. The responsivity of the Bi2O2Te-based infrared detector is up to 104 A W−1 and the detectivity is up to 1014 jones, showing excellent infrared detection performance.22 Photodetectors based on EuMTe3 (M = Bi, Sb) can achieve a response of >1 A W−1 from the UV band to the THz band.23,24 The photodetector, which is based on the MXene (Mo2CTx) material, exhibits a responsivity of 9 A W−1 and a detectivity of 5 × 1011 jones under 660 nm laser irradiation. It shows a good photoresponse in the wavelength range of 325 nm to 1064 nm.25 So far, research on heterolayered materials is still in its infancy. More interest deserves to be devoted to this kind of compound.
Up to now, there have been several decent reviews about 2D materials for infrared photodetectors.26–29 However, there has been no special review about the heterolayered 2D compounds. As an emerging family of materials with superior performance for infrared photodetectors, their progress needs to be summarized. Here, we reviewed the reports of heterolayered materials used for infrared detectors. The crystal structures and properties of the infrared detectors are discussed in detail. Finally, we forecast the fields where the infrared detectors could be used.
2. Parameters of photodetectors
The diverse application scenarios are based on the parameters of photodetectors. The following parameters are generally used to evaluate the performance of a photodetector: responsivity, external quantum efficiency, noise equivalent power, detectivity, and response time. This chapter will introduce the parameters of photodetectors and discuss their effects on device performance.
2.1 Responsivity
The responsivity (R) is defined as the formula (1) and (2) which refers to the ratio of the output photocurrent (Iphotocurrent) or output voltage (Vphotovoltage) to the incident light power (p) under illumination. It indicates the ability of the detector to receive signals. The magnitude of responsivity directly affects the sensitivity and accuracy of photodetectors. The higher the responsivity, the more sensitive the photodetectors to the light signal. There are many factors that affect the responsivity of photodetectors. One of the important factors is the photoconductive gain (G). The gain can be alternatively expressed as the number of photogenerated electron–hole pairs collected by contact to generate a net photocurrent (Iph) divided by the number of photoexcited electron–hole pairs. Therefore, the responsivity can be improved by higher Iph generated by the increased gain.26 |  | (1) |
|  | (2) |
2.2 External quantum efficiency
External quantum efficiency (EQE) refers to the efficiency of converting photons into electrons when photodetectors receive optical signals. Generally speaking, it is the efficiency of photoelectric conversion. The EQE directly affects the sensitivity and signal-to-noise ratio of the detectors. The calculation formula of EQE is: |  | (3) |
where λ is the wavelength of the incident light, q is the electron charge, h is the Planck constant, c is the speed of light, and R is the responsivity. The EQE is usually tested in the visible light band or infrared band, and its numerical range is generally between 0 and 1.30–32 The higher the EQE, the higher the conversion efficiency of the detectors to the optical signal.
Improving the EQE of photodetectors is one of the important ways to improve their performance. In order to improve the EQE, we can start from the following aspects: (1) optimize the structure design of the detectors, including the material selection and thickness design of the photodetector conversion layer, so as to improve the interaction efficiency between photons and electrons; (2) optimize the preparation process of the detectors, improve the purity and uniformity of the material, and reduce the influence of defects and impurities on the photodetector conversion; (3) optimize the working environment of the detectors to ensure the stable input of the optical signal, reduce the noise interference and improve the signal-to-noise ratio. In short, the EQE, as an important performance index of photodetectors, directly affects the sensitivity and signal-to-noise ratio of the detectors. By optimizing the structural design, preparation process and working environment of the detectors, the EQE of the photodetectors can be improved, thereby improving their performance.33–35
2.3 Noise equivalent power
Noise equivalent power (NEP) refers to the influence of noise generated by various reasons in the infrared detection system. This kind of noise will reduce the signal-to-noise ratio of the system, thus affecting the detection performance of the system.36 The NEP directly affects the sensitivity and resolution of the system, so it has an important influence on the performance of the infrared detection system. Therefore, the noise equivalent power characterizes the minimum optical signal power that the detector can distinguish in noise. The definition is shown in formula (4). |  | (4) |
where in is the noise current corresponding to different powers, and R is the response rate.
In the infrared detection system, the noise equivalent power mainly comes from two aspects: (1) the thermal noise of the detector itself and (2) the interference noise of the external environment. The thermal noise of the detector itself is caused by the heat generated by the detector during operation, and this noise will increase with the increase of the detector temperature. The interference noise of the external environment is caused by electromagnetic interference, optical interference, and other factors in the surrounding environment, which will have a great impact on the detection performance of the system. By taking effective measures to reduce the influence of NEP, the detection performance and reliability of the infrared detection system can be improved, so as to meet the needs of various application fields better.37–39
2.4 Detectivity
The specific detectivity is also a performance index to measure the detector's ability to detect the minimum optical signal, which is defined as the reciprocal of the noise equivalent power. In order to compare the performance of different detectors in practical use, the specific detectivity is normalized, and the definition is shown in (5). | 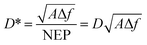 | (5) |
where A is the effective light-receiving area of the device, and Δf is the measurement bandwidth. At the same time, the main noise source of the photodetector is the shot noise from the dark current. Detectivity will be affected by the signal-to-noise ratio of the detector. The signal-to-noise ratio refers to the ratio of the detector between the signal and the noise. The higher the signal-to-noise ratio, the stronger the detector's ability to identify the target, and the higher the detection rate. In infrared photodetectors, the improvement of the signal-to-noise ratio can be achieved by optimizing the circuit design of the detector and reducing the thermal noise of the detector.26
2.5 Response time
The response time (τ) is the time interval between the photodetectors being stimulated by the optical signal and the output electrical signal. The response time is defined as the time occupied by 10% to 90% of the net photocurrent. The response speed of the detector when the signal changes is characterized. The response time is affected by many factors, such as carrier lifetime, bias voltage, and self-defect. The response time mainly depends on the lifetime of photogenerated carriers, so there is a trade-off between responsivity and response time. When the increase of carrier lifetime leads to the increase of photoconductive gain, the responsivity of the device can be improved. However, the response time of the device will increase accordingly.40
3. Mechanism of the photodetectors
There are many physical mechanisms for photodetectors to convert the absorbed optical signal into electrical ones. In this chapter, we focus on two mechanisms of photocurrent generation. The first is the excitation of free carriers caused by the optical effect, including the photovoltaic effect and photoconductivity effect. The second is the thermal effect, including the photothermoelectric effect and bolometric effect.41
3.1 Photovoltaic effect
The photovoltaic effect refers to the absorption of photons by the sample to generate photo-generated carriers when light irradiates the p–n junction. The built-in electric field separates the electrons in the p region from the holes in the n region, and the electrons and holes are stacked on both sides to produce a photoelectric field opposite to the built-in electric field (Fig. 1a).42
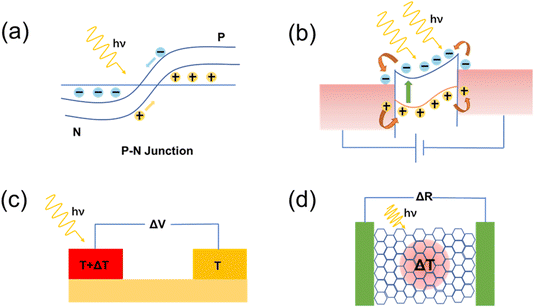 |
| Fig. 1 The diagrams of the mechanisms for (a) the photovoltaic effect, (b) the photoconductive effect, (c) the photothermoelectric effect, and (d) the bolometric effect. | |
3.2 Photoconductive effect
When the incident photon energy absorbed by the semiconductor material is greater than or equal to the band gap energy, electron–hole pairs will be generated, resulting in an increase in carrier concentration. The applied voltage will cause the electron–hole pair to move in the opposite direction and generate photocurrent (Fig. 1b). This phenomenon is called the photoconductive effect. Without light illumination, an external voltage is applied to drive a limited number of free carriers to generate dark current (Idark). Under illumination, the applied voltage drives the electron–hole pairs generated by the absorbed photon to produce a current (Iillumination) which is larger than the dark current. The photocurrent (Iph) can be expressed as Iph = Iillumination − Idark, which can reflect the increase of conductivity of the same semiconductor under different conditions.29
3.3 Photothermoelectric effect
The photothermoelectric effect involves photon absorption, heat conduction, and thermoelectric conversion. First of all, when the photon is irradiated to the surface of the material, the material absorbs the energy of the photon, and the electrons inside the material are excited to the high energy level. These high-level electrons will move inside the material and generate heat energy. Secondly, heat conduction is an important part of the photothermoelectric effect. As the surface temperature of the material increases, thermal energy will spread to the interior of the material through heat conduction. Finally, thermoelectric conversion is a key step in the photothermoelectric effect.41,43 When the surface temperature of the material increases, the temperature gradient inside the material causes electrons to move in the material (Fig. 1c). According to the principle of the thermoelectric effect, the temperature gradient will cause the drift and diffusion of electrons, resulting in uneven charge distribution and eventually forming a potential difference. An external circuit can capture this potential difference to generate electrical signals.44–46
3.4 Bolometric effect
When the incident photon generates heat, the conductance of the thermistor material changes (Fig. 1d). The photocurrent dominated by the radiative thermal effect increases linearly with the bias voltage. Unlike the photothermal effect, the photocurrent generated by the radiation thermal effect requires an external electric field.26
4. Development of materials for infrared photodetectors
Since Theodore W. Case prepared the first Tl2S infrared photodetector in 1917,47 many materials have been explored for infrared photodetectors (Fig. 2). Among them, several materials have been commercialized, such as silicon, HgCdTe, and InGaAs.48–50 The materials suitable for infrared photodetection basically require a narrow band gap (0–1.6 eV), high carrier mobility (>102 cm2 V−1 s−1), fast response time (<10−6 s) and so on (Fig. 3).5,51
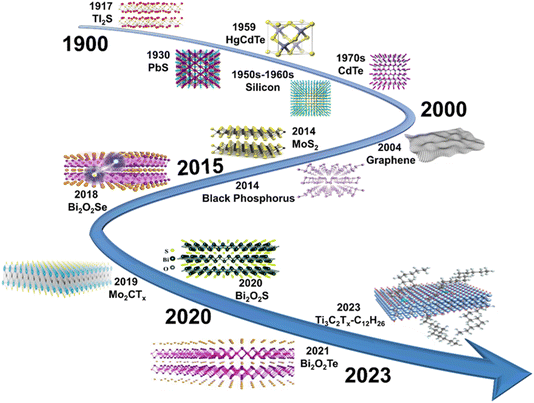 |
| Fig. 2 Timelines of the compounds developed for infrared photodetectors. | |
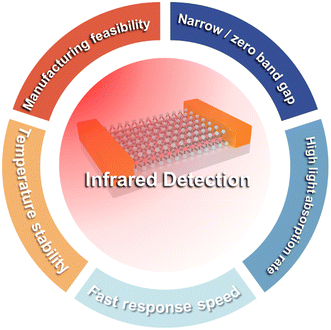 |
| Fig. 3 Requirements of the basic properties of materials that could potentially be used for infrared photodetectors. | |
4.1 3D compounds
4.1.1 Silicon.
Silicon is widely used in the field of infrared photodetection. The abundant silicon resources ensure the large-scale production of silicon and relatively low production costs. Silicon forms a diamond cubic crystal structure with a lattice spacing of 5.42 Å, which corresponds to a face-centered cubic Bravais lattice with a unit cell number containing 8 atoms at the vector position.52 Silicon has high carrier mobility, where the carrier mobility of electrons and holes is 1350 cm2 V−1 s−1 and 480 cm2 V−1 s−1, respectively.53 At 300 K, the carrier concentration of silicon is 1.08 × 1010 cm−3.54 Silicon provides an excellent platform for the implementation of microelectronics-based photodetector readout circuits.43 These merits make silicon promising for application in high-performance infrared photodetectors. Due to the inherent band gap energy of silicon (1.12 eV), the detectable spectral range is limited to wavelengths below 1100 nm. In order to improve the detectable spectral range based on silicon photodetectors, Nazirzadeh M. A. and co-workers used the Au nanoislands formed on the silicon surface by rapidly thermally annealing thin Au layers, so that the silicon photodetector achieved a light response of 2 mA W−1 at 1300 nm.55 (Fig. 4a) Huang and co-workers fabricated silicon photodetectors with a broadband spectral response by femtosecond laser irradiation and titanium hyperdoping. The device can respond to the incident light at 400 nm ∼1550 nm, with an average responsivity of 3.42 mA W−1 at 1550 nm.59 Although there are strategies to extend the detectable spectral range of silicon photodetectors beyond 1100 nm, the problem of the low responsivity still needs to be solved.
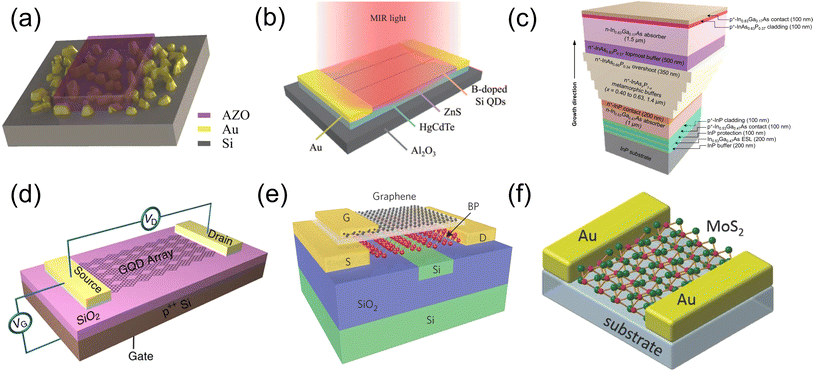 |
| Fig. 4 (a) Schematic diagram of the broad-band NIR Si Schottky photodetector.55 (b) Schematic illustration of the B-doped Si-QD/MCT device structure.56 (c) Structure diagram of the photodetector based on the InGaAs material.57 (d) Schematic diagram of the graphene-based photodetector.13 (e) Schematic diagram of the black phosphorus infrared photodetector.14 (f) Schematic diagram of the MoS2 infrared photodetector.58 | |
4.1.2 HgCdTe.
HgCdTe is one of the important materials used in the fabrication of infrared detectors. The crystal structure of HgCdTe is a face-centered cubic structure with Hg and Cd atoms arranged alternately (space group F
3m).49 The electron mobility and hole mobility of HgCdTe are 105 cm2 V−1 s−1 and 450 cm2 V−1 s−1, respectively.60 The low-temperature carrier concentration of HgCdTe can reach 2 × 1015 cm−3.61 The band gap (0–1.6 eV) of Hg1−xCdxTe is tunable.62,63 Cui and co-workers developed high-sensitivity boron-doped silicon quantum dot/mercury cadmium telluride mid-infrared (MIR) photodetectors (Fig. 4b). The detector has a detectivity of 1.6 × 109 jones at room temperature, a rise time of 224 ns, and a fall time of 580 ns.56 Wang and co-workers introduced multilayer graphene materials into HgCdTe photodetectors and designed and fabricated uncooled HgCdTe photodetectors with ultrafast and high sensitivity characteristics. The device has a fast response time of 13 ns and a room temperature detection rate of 2 × 1010 jones.64 In order to achieve high-performance infrared detection, HgCdTe-based infrared photodetectors usually require low-temperature cooling, which inevitably complicates the system and limits integration.65 High-performance room temperature operation is still a serious challenge for HgCdTe infrared photodetectors.
4.1.3 InGaAs.
InGaAs is a common material for commercial near-infrared photodiodes. The crystal structure and space group of InGaAs are the same as those of HgCdTe. InGaAs has a tunable band gap (from 0.36 to 1.425 eV), and its electron mobility and hole mobility are 1200 cm2 V−1 s−1 and 300 cm2 V−1 s−1, respectively.66 At room temperature, the carrier concentration of InGaAs is ∼1015 cm−3.67 Suho Park and co-workers have grown InGaAs thin films on InP substrates using the chemical vapor deposition approach and then fabricated InGaAs-based infrared photodetectors (Fig. 4c). The detection rate of the device can reach 4.1 × 1011 jones at room temperature.57 Li and co-workers fabricated a broadband flexible detector based on the InGaAs/InP PIN structure. The device has a maximum detectivity of 5.18 × 1011 jones at 640–1700 nm.68 Although InGaAs is competitive in the field of infrared detection, its relatively narrow detection range and large dark current limit its application potential in optical communication.
4.2 2D compounds (van der Waals)
Although the infrared photodetectors based on 3D compounds have been extensively developed, their performances have not been good enough. Hereby, extensive interest has been devoted to the 2D compounds.11,18,69,70 2D materials refer to materials in which the carriers can only move freely on the 2D planes.71 They have a high specific surface area, which can greatly increase the surface area of the interaction with light, thereby improving the detection sensitivity of the photodetector. Photodetectors based on 2D materials can achieve a broadband response (Fig. 5a). The band gap, absorptivity and photoelectric properties of 2D materials can be adjusted by changing the size, shape and composition of materials. For example, the band gap of black phosphorus can change significantly from 0.3 eV (bulk) to 2 eV (monolayer) depending on the number of stacking layers.72 The characteristics of the carrier motion in the plane of the 2D material make it have a fast response speed and high sensitivity. Under illumination, photons will excite carriers inside the material, which can move freely on the plane inside the materials to form a current. Because these carriers can move quickly in the plane of the 2D materials, they have high sensitivity and fast response speed in photodetectors. For instance, the rise time and decay time of photodetectors based on MoS2 are 70 μs and 110 μs, respectively.73Fig. 5b shows several 2D compounds that have been reported for infrared detection, such as graphene, transition metal sulfides, and black phosphorus. In summary, 2D materials are a large family of materials for infrared photodetectors.
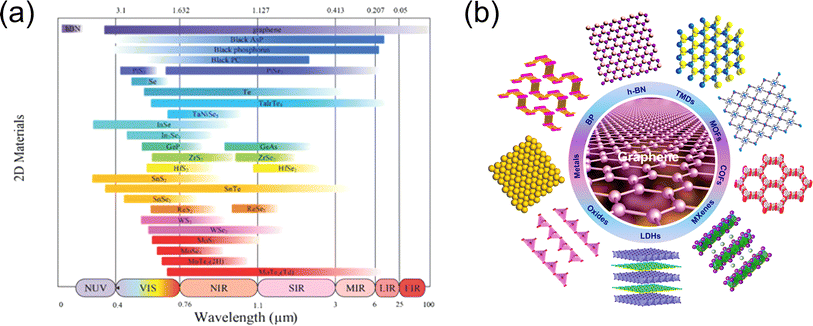 |
| Fig. 5 (a) Comparison of the response wavelength ranges of different 2D materials.41 (b) Diagram of the crystal structures of the well-known 2D compounds.71 | |
4.2.1 Graphene.
Graphene has been used for ultra-wideband photodetectors due to its exceptional crystal structure and physical properties. The crystal structure of graphene is a 2D crystal structure composed of sp2 hybrid orbitals of carbon atoms. Each carbon atom is connected to the other three carbon atoms by a σ bond, and the remaining π electrons form a delocalized large π bond with the π electrons of other carbon atoms. This structure allows electrons to move freely in this region, giving graphene ultrahigh electrical conductivity (2 × 103 S cm−1).74 Graphene exhibits ultrafast carrier dynamics, and its mobility can reach 200
000 cm2 V−1 s−1 at 300 K.75 Graphene is gapless, which makes its absorption spectrum cover the entire UV to THz spectral regimes.76 Zhang and co-workers reported a pure single-layer graphene broadband photodetector with a responsivity of 8.61 A W−1 (Fig. 4d).13 Konstantatos and co-workers spin-coated a layer of PbS quantum dots on the surface of graphene as a local grating layer to prepare a graphene-based photodetector.77 The responsivity of the device can reach 107 A W−1. Unfortunately, the small optical absorption of single-layer carbon atoms limits the responsivity of graphene-based photodetectors.78,79 The integration of colloidal quantum dots in the light absorption layer can improve the responsivity of the graphene photodetector to 107 A W−1, but the spectral range of the photodetector is reduced due to the light absorption occurs in the quantum dots.80
4.2.2 Black phosphorus.
The phosphorus atoms in the crystal structure of black phosphorus show a hexagonal arrangement. Each phosphorus atom is surrounded by three adjacent phosphorus atoms, forming a planar hexagonal structure. Covalent bonds connect the phosphorus six-membered rings in the crystal structure of black phosphorus to form a 2D layered structure. This layered structure is similar to graphite.81 The band gap of black phosphorus could be adjusted by the thickness of the layers. The band gap of monolayer black phosphorus is about 2 eV, while the band gap of bulk black phosphorus is about 0.3 eV.82 At room temperature, the carrier mobility of black phosphorus can reach 1000 cm2 V−1 s−1.83 A wide band gap adjustable range and excellent electrical properties make black phosphorus have great application potential in the field of photodetection.84 Li and co-workers reported a waveguide-integrated black phosphorus photodetector with a low dark current of 220 nA. At room temperature, the responsivity in the near-infrared region reaches up to 657 mA W−1 (Fig. 4e).14 Guo and co-workers reported a high-gain black phosphorus mid-infrared detector with a responsivity of up to 82 A W−1 at a wavelength of 3.39 μm.85 However, the problem of black phosphorus being unstable and degrading over time when exposed to the environment still needs to be solved.
4.2.3 Transition metal chalcogenides.
Transition metal dichalcogenides (TMDs) have unique structures and excellent physical properties. The chemical formula of TMD is MX2, where M is a transition element and X is a chalcogen element. MoS2 is a representative semiconductor belonging to the TMDs. Its crystal structure is that the Mo layer in the hexagonal array is sandwiched between the S layers. Strong covalent bonds characterize the Mo–S interaction, while the interaction between the S layers is a van der Waals interaction along the z-axis.86 MoS2 has a tunable band gap, which can be increased from 1.3 eV (bulk) to 1.8 eV (monolayer).87 The ultra-wideband multilayer MoS2 photodetector reported by Xie and co-workers has a responsivity of 50.7 mA W−1 in the wavelength range of 445–2717 nm (Fig. 4f).58 PtSe2 is also a typical TMD material. PtSe2 is a layered structure, in which each layer consists of Pt and Se atoms forming a hexagonal lattice. PtSe2 has a tunable band gap of 0 (bulk) ∼1.2 (monolayer) eV.88 The carrier mobility of PtSe2 can reach 4038 cm2 V−1 s−1.89 The field effect transistor based on PtSe2 prepared by Zhang and co-workers has a responsivity of 0.63 A W−1.90 In order to further improve the performance of PtSe2-based devices, Xu and co-workers fabricated photodetectors based on the PtSe2/SiO2/Si heterojunction. The PtSe2/SiO2/Si heterojunction greatly suppresses the dark current and improves the performance of the device. The dark current of the device is as low as 0.12 pA, and the responsivity is as high as 8.06 A W−1.91 WTe2 also belongs to the TMD material. Its crystal structure is orthogonal (space group Pmn21), and each layer of the structure is composed of two layers of tellurium atoms sandwiched between a layer of tungsten atoms.92 WTe2 exhibits semimetal properties at room temperature. It has an electron mobility of 3.8 × 104 cm2 V−1 s−1 and a hole mobility of 800 cm2 V−1 s−1.93 Xiao and co-workers used laser pulse deposition to deposit large-area WTe2 films. The responsivity and detectivity of the photodetector based on the WTe2 thin film are 1.4 mA W−1 and 2.3 × 106 jones, respectively.94 Although TMDs have shown considerable application potential in the infrared detection technology, the growth of large-scale and high-quality TMDs is still challenging.95
4.2.4 2D heterostructures.
Although photodetectors based on single 2D materials offer significant merits in the field of infrared detection, their performance still requires improvement. For instance, it is challenging for these photodetectors to achieve high responsivity and detectivity in infrared photodetection simultaneously, due to the fact that high responsivity is typically accompanied by large dark currents, thereby diminishing detectivity. By reasonably stacking two-dimensional materials with other materials, 2D/0D heterojunctions, 2D/2D heterojunctions and 2D/3D heterojunctions can be formed. The formation of heterostructures can reduce the shortcomings of a single material and improve the overall performance of the detector. Due to the lack of dangling bonds, two-dimensional materials can form vertical heterojunctions without considering any lattice matching conditions. 2D heterojunction-based photodetectors can combine the detection wavelength bands of two materials, thereby expanding the detection wavelength range. Additionally, by constructing a PN junction, the photovoltaic effect can be enhanced, leading to an improvement in response speed. For example, the response times of GeSe-based photodetectors, InSe-based photodetectors, and WS2-based photodetectors are 0.7 s, 5 s, and 37.2 ms,96–98 respectively, while the response times of graphene/InSe/GaSe/graphene-based photodetectors and GaSe/WS2 heterojunction-based photodetectors can reach 2 μs and 37 μs,99,100 respectively, which are much faster than those of single-material-based photodetectors. Furthermore, BP heterojunction-based photodetectors can also be used for polarization detection at 1550 nm.101 Although 2D heterostructures have many merits in the field of infrared photodetection, there are still obstacles to overcome in practical applications. For instance, when transferring 2D materials to build heterostructures, contaminants and defects are inevitably introduced into the material or interface, resulting in degraded device performance.29
5. 2D compounds with heterolayers for photodetection
The crystal structures of typical 2D materials, heterolayered materials, and 3D materials are shown in Fig. 6a. Fig. 6b summarizes the responsivity of photodetectors based on typical 2D, heterolayered, and 3D compounds from the UV to MIR region. Compared with other materials, photodetectors based on heterolayered compounds have obvious advantages in the responsivity of this band. The properties of heterolayered materials can be modulated by adjusting the thickness or by introducing different ionic layers. Through the combination of different ion layers, heterolayered compounds can attain high responsivity, high sensitivity, and low power consumption. This kind of versatility is hard to achieve in 2D and 3D materials, providing photodetectors based on heterolayered compounds with significant competitiveness in detecting fields demanding high performance. Heterolayered compounds are formed by the alternating stacking of two or more layers with distinct chemical properties and thereby possess unique traits. It is found that their physical properties are determined by the structures of the constituents and interactions between the layers. Each layer is composed of several thickness of atoms, which enables the 2D feature. Different from the conventional 2D materials (such as graphene, MoS2, etc.), the strong chemical bonding leads to the carriers transfer between the layers, weakening the anisotropic properties and enhancing the 3D properties.21,102 Examples include NaCu4Se4 with non-saturating, large, and linear magnetoresistance, the charge density wave material KLaM1−xTe4 (M = Mn and Zn), [Pb3.1Sb0.9S4][AuxTe2−x] with anomalous, transverse non-saturating negative magnetoresistance, and RbEuFe4As4 with superconductivity and ferromagnetism.103–106 Proper doping in one layer will have a large impact on the other one.
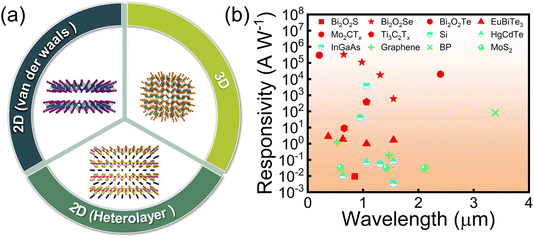 |
| Fig. 6 (a) Comparison of the crystal structures of typical 2D, heterolayered, and 3D compounds. (b) The reported responsivities of photodetectors of typical 2D, heterolayered, and 3D compounds in the UV to MIR region. | |
Bi2O2Se is a representative semiconductor as a heterolayered material. As shown in Fig. 7, the crystal structure of Bi2O2Se consists of alternating positive and negative layers. The positive layer is [Bi2O2]n2n+ and the negative layer is [Se]n2n−, with carriers transfer between the layers.21 In addition, the negative layer can be replaced by elements of the same main group to form other heterolayered materials (Bi2O2S, Bi2O2Te), thereby expanding the family of heterolayered materials. In the present era, a limited number of heterolayered materials have been used for infrared photodetectors, such as Bi2O2X (X = S, Se, Te), EuMTe3 (M = Sb, Bi), and MXene.25,107–109 Heterolayered materials have attracted the attention of many researchers in the field of infrared photodetection.108,110–116Table 1 lists the detailed parameters of the performances of infrared photodetectors based on the typical 2D, heterolayered, and 3D materials. Currently, the detection range of photodetectors based on heterolayered materials is from visible to near-infrared wavelengths. The responsivity of the photodetector based on Bi2O2Se in the near-infrared range is 1.1 × 105 A W−1,123 which exceeds that of most near-infrared photodetectors.127–130 Additionally, Ti3C2Tx-based and Mo2CTx-based photodetectors exhibit commendable performance in the near-infrared range.25,131 Due to the relatively large band gaps, it is difficult for photodetectors based on Bi2O2X (X = S, Se), Ti3C2Tx, and Mo2CTx to achieve mid-far infrared photodetection. Surprisingly, those heterolayered materials (Bi2O2Te, EuSbTe3, and EuBiTe3) with narrow band gaps (<0.4 eV) have also not been explored for mid-far infrared photodetection. In the near future, photodetectors based on heterolayered materials for mid-far infrared photodetection need to be explored.
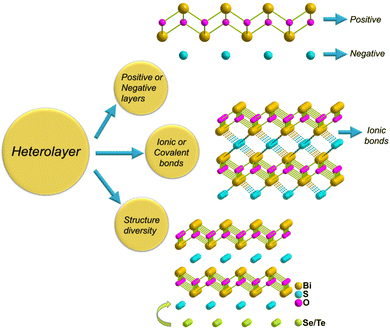 |
| Fig. 7 Scheme of the construction of Bi2O2X (X = S, Se, Te). | |
Table 1 A summary of the performances of the typical 2D materials, heterolayered materials, and 3D materials used for infrared photodetectorsa
Materials |
Dimension |
E
g (eV) |
R (A W−1) |
D* (jones) |
Res./rec. time |
Wavelength (nm) |
Ref. |
E
g: band gap, R: responsivity, D*: detectivity, res./rec.: response/recovery.
|
Si |
3D |
1.12 |
40.59 |
3.61 × 1012 |
— |
950–1550 |
59
|
HgCdTe |
0–1.6 |
2.5 |
2 × 1010 |
13 ns |
520–4252 |
64
|
InGaAs |
0.36–1.425 |
3700 |
1.01 × 1011 |
30/180 ms |
1064 |
117
|
Graphene |
2D |
0 |
0.2 |
— |
— |
532–10 310 |
13
|
BP |
0.3 |
82 |
— |
— |
532–3390 |
85
|
MoS2 |
0.26 |
3.25 × 10−2 |
1 × 109 |
— |
445–2717 |
58
|
PtSe2 |
0.3 |
0.63 |
1.95 × 109 |
— |
405–808 |
90
|
WTe2 |
0 |
1.4 × 10−3 |
2.3 × 106 |
— |
1064 |
94
|
Bi2O2S |
Heterolayered |
1.16 |
5.9 × 10−2 |
6.77 × 109 |
81.9 ms |
532 |
118
|
Bi2O2S |
1.5 |
2.31 × 10−3 |
— |
0.08 s |
250–550 |
119
|
Bi2O2S |
0.67 |
9.48 × 10−3 |
9.96 × 1010 |
227.7 ms |
850 |
120
|
Bi2O2Se |
0.8 |
65 |
3.0 × 109 |
1 ps |
300–1700 |
121
|
Bi2O2Se |
0.8 |
300 |
— |
5.42/2 ms |
940–1550 |
122
|
Bi2O2Se |
0.8 |
3.2 × 105 |
3.28 × 1013 |
2.1 μs |
650, 1500 |
123
|
Bi2O2Se |
0.8 |
3.712 × 103 |
3.3 × 1010 |
1.77/1.45 ms |
300–900 |
124
|
Bi2O2Te |
0.3 |
3 × 105 |
4 × 1015 |
77/126 ms |
210–2400 |
22
|
EuBiTe3 |
0.3 |
1 |
— |
40 ms |
370–1550 |
24
|
EuSbTe3 |
0.1 |
1 |
2.4 × 1014 |
8 ms |
325–1550 |
23
|
Ti3C2Tx |
0.49–2.15 |
1.33 × 10−4 |
1.46 × 108 |
— |
650 |
125
|
Ti3C2Tx |
1.53 |
8.50 × 102 |
3.69 × 1011 |
— |
1060 |
126
|
Mo2CTx |
— |
9 |
5 × 1011 |
500 ms |
400–800 |
25
|
5.1 Layered bismuth oxychalcogenides
In recent years, Bi2O2X (X = S, Se, Te), as an emerging 2D material, has received much attention due to its applications in thermoelectric and photoelectric field applications.110,118,123,132–136 The Bi2O2X (X = S, Se, Te) materials have two different crystal structures, Bi2O2S belongs to a primitive orthorhombic lattice with a space group Pnnm, whereas Bi2O2Se and Bi2O2Te have a body-centered tetragonal lattice with space group I4/mmm.110–113 Bi2O2S has both ferroelasticity and antiferroelectricity, Bi2O2Se exhibits ferroelasticity and piezoelectricity under in-plane strain and Bi2O2Te has intrinsic out-of-plane ferroelectricity.137–139 As shown in Fig. 8a, Bi2O2X is connected by ionic bonds, where the [Bi2O2]n2n+ positive layer and the [X]n2n− negative layer are alternately stacked. Bi2O2X also has the characteristics of conventional 2D materials, and the band gap value can be changed by adjusting the number of layers.140 Its unique properties have attracted the attention of many researchers. ‘Wei et al. proposed a model called the ‘zipper model’, which explains the structure of 2D Bi2O2X well, as shown in Fig. 8b. Each layer contains a [Bi2O2]n2n+ positive layer and the top [X]n2n− negative layer. The 50% X on the lower surface of the layer and the 50% X on the upper surface of the bottom [X]n2n− anion layer are connected by electrostatic force, which is similar to zipper.107
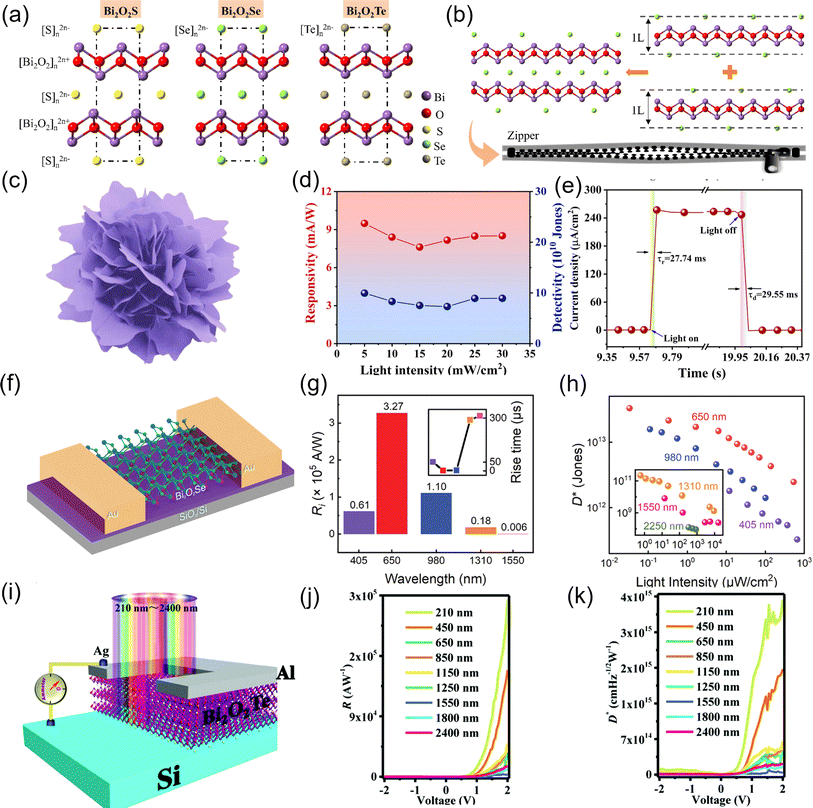 |
| Fig. 8 (a) Crystal structures of Bi2O2S, Bi2O2Se and Bi2O2Te.140 (b) Scheme of a bilayer Bi2O2Se formed by two monolayers and a zipper model.140 (c) Bi2O2S nanoflower diagram.120 (d) A single magnified photoresponse curve under infrared light illumination with a light intensity of 30 mW cm−2.120 (e) Responsivity and detectivity as a function of the light intensity.120 (f) The schematic illustration of the photodetector.122 (g) The responsivities in wavelengths ranging from 405 to 1550 nm.123 (h) Variation in detectivity as a function of the light intensity at wavelengths ranging from 405 to 1550 nm.123 (i) Schematic diagram showing the structure of the photodetector, composed of 2D Bi2O2Te and the n-Si substrate, with an active area of 4 mm2 and responding to a broad wavelength between 210 nm and 2.4 μm.22 (j and k) Responsivity and detectivity against voltage curves at different wavelengths.22 | |
5.1.1 Bi2O2S.
Bi2O2S is a layered bismuth oxysulfide family material with a zipper structure. It is stacked by [Bi2O2]n2n+ cation layers and [S]n2n− anion layers.141 Bi2O2S has a tunable band gap (from 0.67 to 1.15 eV). It is loosely structured with a crystal stacking factor of 0.66,119 which leads to the high carrier mobility of Bi2O2S. The electron mobility of monolayer hydrogen passivated Bi2O2S at 300 K is 16
447–266
99 cm2 V−1 s−1.142
In 2020, Li et al. reported the Bi2O2S-based photodetector for the first time.118 Bi2O2S nanosheets were synthesized by a one-step hydrothermal method, and Bi2O2S thin film photodetectors were prepared by thermal spraying. The response time of the device is 81.9 ms under 532 nm light irradiation. The highest responsivity is 0.059 A W−1, and the highest detectivity is 6.77 × 109 jones.118 Wang et al. synthesized Bi2O2S nanosheets and constructed a photoelectrochemical photodetector based on Bi2O2S nanosheets. The device has self-healing properties under alkaline conditions, which can prolong the lifetime of photogenerated carriers. Therefore, it has a longer working time. The response time can reach 0.08 s, the response rate can reach 2.31 mA W−1, and it has long-term stability at 0.6 V bias voltage.119 Rong et al. prepared Bi2O2S nanoflowers with hierarchical structure by a hydrothermal method (Fig. 8c). The responsivity, detectivity and response time of the infrared photodetector based on Bi2O2S nanoflowers are shown in Fig. 8d and e. Under 850 nm infrared light, the responsivity of the Bi2O2S nanoflower photodetector is 9.48 mA W−1, the detectivity is 9.96 × 1010 jones, and the response time is 27.74 m.120
5.1.2 Bi2O2Se.
Bi2O2Se is the most studied member of the bismuth sulfide oxide semiconductor family by researchers.143,144 Bi2O2Se has a tetragonal crystal structure. The crystal structure of Bi2O2Se is composed of alternating stacking of [Bi2O2]n2n+ positive layers and [Se]n2n− negative layers.145,146 Bi2O2Se has a narrow bandgap (0.8 eV). The Hall mobility of Bi2O2Se is 29
000 cm2 V−1 s−1 at 1.9 K and 450 cm2 V−1 s−1 at room temperature.110–121 In addition, it also has air stability at room temperature.144 These properties make Bi2O2Se show outstanding application potential in the field of infrared detection.147–153 Peng et al. synthesized Bi2O2Se nanosheets on mica substrates by chemical vapor deposition. The infrared photodetector based on Bi2O2Se nanosheets has high sensitivity in the extremely wide spectral range of 300–1700 nm, high responsivity of 65 A W−1 at 1200 nm, and ultrafast light response of 1 ps at room temperature.121 Chen et al. synthesized high-quality, large-area Bi2O2Se nanosheets by the CVD method, which realized broadband detection from infrared to THz. Under 940, 1060, 1310 and 1550 nm laser irradiation, the maximum responsivity reached 300, 121, 53 and 58 A W−1, respectively.122 Wei et al. synthesized Bi2O2Se nanobelts on fluorophlogopite substrates by chemical vapor deposition. A photodetector exhibiting both fast response and low noise was developed by establishing a Schottky barrier between the Bi2O2Se nanobelts and the Au electrode (Fig. 8f). The responsivity of the device at 650 nm is 3.2 × 105 A W−1 (Fig. 8g). At 650 nm and 1550 nm, the response time is 2.1 μs and 313 μs, and the detectivities are 3.28 × 1013 and 8.07 × 109 jones, respectively (Fig. 8h).123 Zou et al. studied the heat-treated Bi2O2Se nanoplates and found that they can also have extremely high air stability in harsh environments. The infrared photodetector based on the heat-treated Bi2O2Se nanosheets also has high sensitivity and can work stably at 250 °C. The detectivity reaches 3.3 × 1010 jones and has an ultra-high responsivity of 3712 A W−1.124 Nevertheless, Bi2O2Se is an indirect bandgap semiconductor with weak light absorption, which results in a significant decline in its photoresponse performance at a wavelength of 1550 nm.121
5.1.3 Bi2O2Te.
As a new member of the bismuth oxysulfide family, Bi2O2Te inherits comparable optoelectronic properties to Bi2O2Se. Compared with Bi2O2Se, Bi2O2Te has a lower thermal conductivity, narrower band gap and higher carrier mobility.154,155 Bi2O2Te crystallizes in a space group of I4/mmm and the crystal structure of Bi2O2Te consists of [Bi2O2]2+ layers and [Te]2− square net layers alternately stacked along the c-axis.156 The ultra-thin Bi2O2Te crystals synthesized by the chemical vapor deposition method by Ai et al. have ultra-low temperature Hall mobility (>20
000 cm2 V−1 s−1).157
Tian et al. successfully prepared 2D Bi2O2Te films by the magnetron sputtering and rapid annealing approach. Based on Bi2O2Te thin films, an ultra-sensitive photodetector for weak light detection was fabricated (Fig. 8i). The device has a wide spectral detection range of 210–2400 nm. The responsivities to deep UV and short-wave infrared are 3 × 105 and 2 × 104 A W−1, respectively (Fig. 8j). The detectivities are 4 × 1015 and 2 × 1014 jones, respectively (Fig. 8k). Due to the saturated absorption characteristics of the surface state of the 2D material, the device exhibits better performance under weak light irradiation than under strong light irradiation.22 Yang et al. synthesized Bi2Te3 nanosheets by the solvothermal method and then prepared 2D Bi2O2Te nanosheets by low-temperature oxidation. The researchers prepared a quasi-solid-state photoelectrochemical-type (PEC-type) photodetector based on 2D Bi2O2Te nanosheets and studied its performance. The PEC-type Bi2O2Te photodetector exhibits significant photoresponsive properties in the spectral range from 365 to 850 nm. Its responsivity at 365 nm is up to 20.5 mA W−1.154
5.2 EuMTe3 (M = Bi, Sb)
EuMTe3 is a layered bimetallic telluride with a low band gap value. EuMTe3 crystallizes in a layered structure in the Pmmn space group. As shown in Fig. 9a, it consists of a stacked Eu–Te–M slab and a Te atomic layer, where the Te atomic layer is sandwiched between the Eu–Te–M slab. Inside the Eu–Te–M plate, M–Te and Eu–Te zigzag network structures are tightly bonded.108 In 2015, Niu et al. synthesized ternary tellurides EuMTe3 (M = Bi, Sb) by the low-temperature flux method and studied their properties. The optical band gaps of EuSbTe3 and EuBiTe3 are 0.1 eV and 0.3 eV, respectively.108
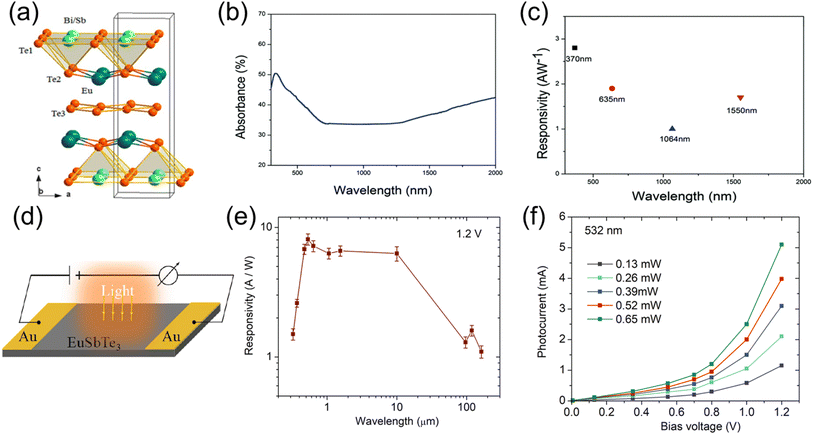 |
| Fig. 9 (a) The schematic illustration of the crystal structure of EuMTe3 (M = Bi, Sb).108 (b) RT ab-plane optical absorbance spectrum of EuBiTe3 crystals.24 (c) The responsivity for our EuBiTe3 detectors under illumination with wavelengths from UV to near-infrared (NIR) based on the same device.24 (d) The schematic diagram of the EuSbTe3 photodetector.23 (e) For applied bias 1.2 V, the available ultra-broadband photoresponsivities from UV to THz at various illuminations at 325 nm, 370 nm, 473 nm, 532 nm, 635 nm, 1064 nm, 1550 nm, 10.6 μm, 96.5 μm, 118.8 μm and 163 μm, respectively.23 (f) The photocurrent as a function of applied bias voltage under 532 nm illumination.23 | |
5.2.1 EuBiTe3.
Niu et al. prepared EuBiTe3 by the flux method, and obtained EuBiTe3 flakes from EuBiTe3 crystals by the transparent tape method, and deposited Au electrodes on the surface of EuBiTe3. Based on EuBiTe3 flakes, photodetectors were fabricated on EuBiTe3 flakes. Fig. 9b and c show that the device has a broadband response at 370–1550 nm with a responsivity greater than 1 A W−1, and the performance does not change significantly after exposure to air at room temperature for three months.24
5.2.2 EuSbTe3.
Niu et al. further prepared EuSbTe3 single crystals by the flux method and then peeled out EuSbTe3 flakes using the transparent tape method, and fabricated photodetectors based on EuSbTe3 flakes. The structure of the EuSbTe3 photodetector is shown in Fig. 9d. The device has excellent direct photosensitive performance in the UV-THz wavelength range. Fig. 9e and f show the photoresponsivity of the device is greater than 1 A W−1 in the THz wavelength range, under the illumination of 532 nm, the photocurrent increases rapidly and nonlinearly with the increase of bias voltage. And it can maintain stable performance after three months at room temperature in air.23
5.3 MXenes
MXene is a new type of 2D crystal compound with a hexagonal structure and novel properties.158 The general formula of MXene is Mn+1Xn or Mn+1XnTx (n = 1, 2, 3), where M is a transition metal (M = Sc, Ti, Ta, Hf, Zr, V, Nb, Cr, Mo), X is carbon or nitrogen, and Tx represents functional groups, such as –O, –OH, –F and –Cl. MXenes have excellent light transmittance, and the light transmittance of many MXenes can reach more than 90%.159–161 It also has extremely conductivity, and its composite with graphene exceeds the conductivity of commercial indium tin oxide films. By compositing with a small amount of graphene, MXene/graphene composites with a conductivity of 9.5 × 104 S cm−1 can be obtained.162 These unique properties have made them highly sought after in the field of photodetection.109,163–171 However, the extremely narrow band gap (0–0.1 eV) of MXene containing –F, –OH and –O terminal groups limits its application in the field of photodetectors.172
As a representative material of the MXene family, Ti3C2Tx has high electrical conductivity, and the conductivity of Ti3C2Tx thin films reaches 8000–25
000 S cm−1.158,173 Furthermore, the material exhibits high transparency, and a single Ti3C2Tx layer can transmit about 97% of visible light.174 The structure of Ti3C2Tx is shown in Fig. 10a.114,115 Hu et al. prepared semiconducting Ti3C2Tx MXene by grafting dodecyl (–C12H26) groups on Ti3C2Tx MXene materials. The band gap (from 0.49 to 2.15 eV) can be changed by adjusting the number of layers of the semiconductor Ti3C2Tx MXene. Fig. 10b shows the I–V curves of Ti3C2Tx-based infrared photodetectors under laser irradiation at 1064 nm with different optical power densities. Under the irradiation of a 1064 nm laser, the responsivity and detectivity can reach 1.33 × 10−4 A W−1 and 1.46 × 108 jones, respectively (Fig. 10c).125 Liu also found that Ti3C2Tx-based infrared photodetectors generate polarized photocurrent at zero bias under the irradiation of a 1064 nm laser.175 In a separate study, Hu et al. prepared the semiconductor Ti3C2Tx MXene (S-Ti3C2Tx) with a band gap of 1.53 eV using a benzenesulfonic acid group (–phSO3H) as a surface functional group. The phenylsulfonic acid group modified Ti3C2Tx MXene (S-Ti3C2Tx)-based flexible photodetector has a maximum responsivity of 8.50 × 102 A W−1 and a detectivity of 3.69 × 1011 jones under 1064 nm laser irradiation.126
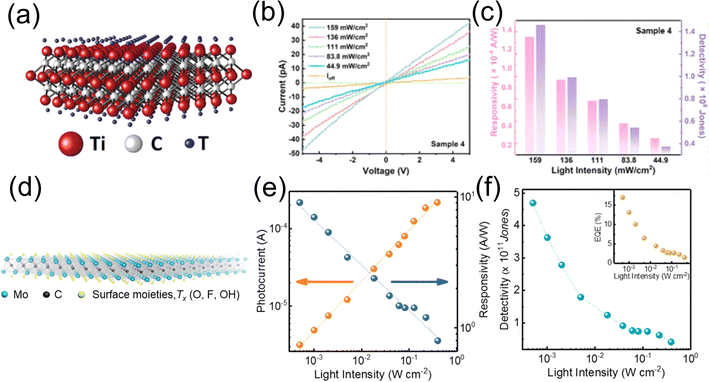 |
| Fig. 10 (a) Ti3C2Tx structure diagram.109 (b) I–V curves under laser irradiation of 1064 nm with different optical power densities.125 (c) The detectivity and responsivity under different optical power densities at 1064 nm.125 (d) Structure diagram of Mo2CTx.25 (e) Responsivity of the photodetector as a function of different light intensities.25 (f) The detectivity of the photodetector as a function of different light intensities.25 | |
As another member of the MXene family, Mo2CTx has high electrical conductivity and optical properties and can achieve a broadband response in the UV and near-infrared bands.116 The structure of Mo2CTx is shown in Fig. 10d. The Mo2CTx material can achieve high sensitivity detection of infrared light, and the detection sensitivity is even higher than that of HgCdTe and InGaAs. The photodetector based on the Mo2CTx material obtains a weak optical response in the range of 325–1064 nm, the responsivity is 9 A W−1 and the detectivity is 5 × 1011 jones under 660 nm laser irradiation (Fig. 10e and f).25 The above studies have proved the feasibility of Mo2CTx materials for infrared photodetection. At present, the most studied is still the infrared photodetector based on Ti3C2Tx. There are relatively few studies on other members of the MXene family applied to infrared photodetectors.
6. Application of infrared photodetectors
6.1 Cell detection
So far, most cancer patients have died of metastatic tumors (CTC). Therefore, the development of high-performance sensing systems for CTC measurement is very important for early diagnosis, prognosis and accurate treatment of cancer patients.176 Photoelectrochemical (PEC) immunosensing technology is a new type of CTC detection technology. Compared with traditional CTC detection techniques (chromatography-mass spectrometry, enzyme-linked immunosorbent assay, and fluorescence spectroscopy),177–179 the PEC immunosensing system is more sensitive and stable for the detection of CTCs.180 The core of the PEC immunosensor is the photosensitive material.181 Xu and co-workers used Bi2O2S nanoflowers as photosensitive elements to construct a near-infrared photoelectrochemical (PEC) biosensing interface that can be excited with an 808 nm laser. The mechanism of the near-infrared PEC biosensor interface for detecting tumor cells is shown in Fig. 11a. The aptamer was immobilized on the surface of AuNPs/Bi2O2S/ITO, which can selectively capture tumor cells. When the tumor cells were captured on the AuNPs/Bi2O2S/ITO surface, the photocurrent of the PEC biosensor interface decreased accordingly. The change of current achieves the quantitative detection of tumor cells.182
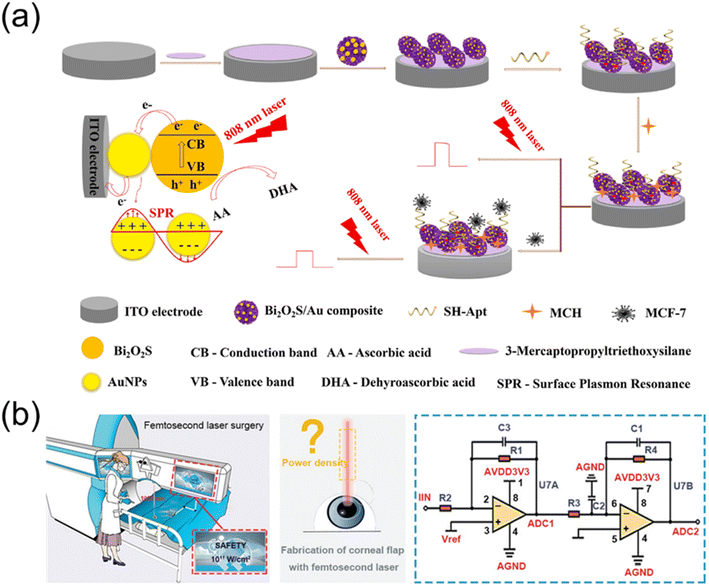 |
| Fig. 11 (a) The fabrication of the PEC aptamer sensor and the detection mechanism of tumor cells.182 (b) Ti3C2Tx-RAN photodetector monitors laser density display and laser density monitoring system circuit diagram during femtosecond laser surgery.183 | |
6.2 Monitoring
In laser operation, the monitoring of optical power density is particularly important. For example, the femtosecond laser is needed for myopia recovery surgery. The femtosecond laser has the advantages of high instantaneous power, short pulse time and accurate focusing range.184 Hu and co-workers used Ti3C2Tx MXene as the conductive electrode and RAN films as the active material to design an infrared photodetector based on the Ti3C2Tx-RAN heterostructure. During surgery, the monitor platform is adjusted to the same height as the eye. When the laser is turned on, the power density monitoring system composed of Ti3C2Tx-RAN converts the optical signal into a current signal. After being amplified by the I–V circuit, the current signal is collected by the microprocessor for processing and conversion. Finally, the optical power density of the laser is displayed on the screen. The device can monitor the optical power density of the laser during myopia surgery to prevent excessive laser power from causing damage to the eyes (Fig. 11b).183
6.3 Optical communication
Flexible infrared photodetectors have great advantages in optical communication. By combining MXene (Ti3C2Tx) and photosensitive lead sulfide quantum dots, skin-like photodetectors and arrays with simple bilayer configurations are fabricated. The separation of photocarriers is highly promoted at the interface of the bilayer, and efficient transmission is achieved between the spatial separation layers. A double-layer photodetector based on MXene/PbS was obtained by spin-coating MXene and lead sulfide quantum dots on quartz and PI substrates, and then evaporating silver electrodes (Fig. 12a). Fig. 12b is a schematic diagram of a two-layer photodetector array based on MXene/PbS. The flexible wireless NFC system is integrated with a MXene/PbS-based double-layer photodetector array (Fig. 12c). The NFC system can store and wirelessly transmit distance-containing information by NIR-II light reflected from fingers or other objects. When the finger is closer to the sensor, more light will be reflected on the sensor and produce higher photocurrent. When the hand leaves, the current decreases due to less light irradiated on the sensor. The transmission of information is realized by encoding and analyzing the change of current (Fig. 12d).185
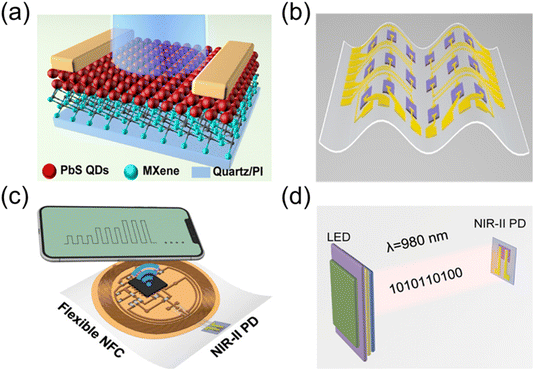 |
| Fig. 12 (a) MXene/PbS infrared photodetector schematic diagram.185 (b) A skin-like bilayer photodetector array was prepared on the PI substrate.185 (c) A schematic diagram of a wireless photodetection system using flexible NFC and NIR-II photodetectors.185 (d) The schematic diagram of optical communication through NIR-II light.185 | |
7. Outlook of heterolayered materials for infrared detectors
Heterolayered materials have great research potential in the field of optoelectronics. In fact, there is a large number of heterolayered compounds in the inorganic crystal structure database (ICSD) such as RETe2, RETe3 (RE = Y, La, Ce, Pr, Nd, Sm, Gd, Tb, Dy, Ho, Er and Tm), MX2 (M = Eu, Gd; X = Ge, Si) and so on.186–188 RETe3 has a heterolayered structure with a unit cell consisting of two RE-Te plates separated by two Te sheets.187 RETe3 has a small effective mass and very high carrier mobility, and GdTe3 has the highest mobility among all known 2D layered magnetic materials. The electron mobility is beyond 60
000 cm2 V−1 s−1.189 Coupled with silicene and germanene, Eu and Gd formed a new 2D RE compounds MX2 (M = Eu, Gd; X = Ge, Si). The stoichiometric MX2 has layered structure. In MX2, Eu or Gd forms the triangular lattice, while silicene or germanene forms the 2D honeycomb network.188 EuGe2 is a bipolar magnetic semiconductor with a band gap of 0.55 eV, and its carrier mobility can be up to 104 cm2 V−1 s−1.190,191 EuSi2 is a semiconductor with an indirect surface band gap of 0.45 eV.192 GdGe2 is a ferromagnetic half-semiconductor and an indirect band gap of 0.55 eV.193 The narrow bandgaps of MX2 allow them to be excited by infrared light, and they also have high enough carrier mobility. It is foreseeable that the research of heterolayered compounds in the field of infrared photodetection will definitely have vigorous development in the near future.
8. Conclusions
In summary, we begin by outlining the evolution of materials with 3D and 2D structures that are apt for infrared photodetectors. Then we introduced the research progress of infrared photodetectors based on heterolayered materials. Photodetectors based on heterolayered materials have been reported to have broadband detection, THz detection, polarization-sensitive light detection, etc. Bi2O2S has self-healing properties under alkaline conditions to extend the lifetime of photogenerated carriers. Bi2O2Se-based photodetectors have a broadband response from UV to THz, with high responsivity and detectivity. However, due to its indirect bandgap semiconductor and weak light absorption, its optical response at 1550 nm is significantly reduced. Bi2O2Te has polarization-sensitive photodetection properties, but its synthesis process still needs to be optimized. Although EuMTe3 (M = Sb, Bi) has extremely high stability at room temperature and can maintain its performance after being placed in air at room temperature for 3 months, there are still few studies on them. MXene can achieve a fast response and has high chemical stability and thermal stability. By introducing surface functional groups to regulate the band gap of Ti3C2Tx, its response in the infrared band is realized. Moreover, it has high transparency and flexibility, which provides conditions for the preparation of transparent and flexible/stretchable electronic devices. However, except for Ti3C2Tx, other members of the MXene family have been studied less in the field of infrared photodetection.
Although heterolayered materials have been extensively studied, there is still considerable research value and scope for improvement in the field of infrared photodetection.
(1) Firstly, many heterolayered materials with suitable band gaps are identified in the inorganic crystal structure database, such as RETe2, RETe3 (RE = Y, La, Ce, Pr, Nd, Sm, Gd, Tb, Dy, Ho, Er, and Tm), MX2 (M = Eu, Gd; X = Ge, Si), etc. Investigations of these compounds for detectors are worthy to be conducted. Secondly, the construction of different 2D constituents could be designed to form new compounds. The functional layers need to have comparable lattice parameters and strong bonds between the outermost ions, leading to new materials with promising properties. For instance, the combination of superconducting layers and magnetic layers lead to the formation of new magnetic superconductors, like RbEuFe4As4 with a superconducting [FeAs] layer and a ferromagnetic Eu layer.105
(2) Current research on infrared photodetectors made of heterolayered materials mainly focuses on the NIR wavelength band, while studies on the MIR and far-infrared (FIR) wavelength bands have lagged behind. The performance of the heterolayered material photodetector can be optimized by adjusting the physical properties of the semiconductors. Considering the doping elements, different elements can introduce new energy levels in the forbidden band, facilitating the generation and separation of photogenerated carriers and improving photoelectric performance. As for the doping concentration, a too low concentration may not fully introduce the needed energy levels, leading to insufficient generation and separation efficiency of photogenerated carriers, affecting light absorption and photoelectric conversion efficiency of the material. However, an overly high concentration may cause lattice distortion, reduce carrier mobility, and impede charge transport, adversely affecting the photoelectric performance. An appropriate doping concentration can optimize the energy band structure of heterolayered materials, enhance charge separation and transport efficiency, boost light absorption, and significantly enhance photoelectric performance. As for doping sites, doping at specific lattice sites can introduce new energy levels, altering the positions and distributions of the valence band maximum and conduction band minimum, directly influencing the material's light absorption and charge transport properties. Meanwhile, for the heterolayered materials, when the doping site is close to the interface, it may change the band bending and charge distribution at the interface, influencing the charge transfer and separation efficiency at the interface and thereby affecting the photoelectric performance.
(3) The response speed of infrared photodetectors based on heterolayered materials is frequently sluggish. A fast response can be attained by optimizing the carrier mobility of the material, enhancing the electrodes of the device, and establishing a heterojunction structure. Regarding carrier mobility, optimizing the growth process can improve crystal quality and minimize the presence of impurities and defects. This is because impurities and defects scatter the carriers, impeding their free movement and thereby reducing their mobility. Optimizing the electrode by selecting an electrode material with high conductivity, such as a metal with excellent conductivity, can lower the electrode resistance, accelerate the transport of carriers within the electrode, and thus shorten the response time. Appropriately reducing the thickness of the electrode can decrease the resistance and capacitance of the electrode and mitigate the delay of carrier transmission. The utilization of a rational electrode structure can increase the contact area between the electrode and the detection material, enhancing the carrier collection efficiency, and decreasing the response time. Appropriate electrode spacing and arrangement also contribute to reducing the effects of capacitance and resistance. For heterojunction structures, choosing two materials with matching band structures, such as combining a material with a wide bandgap and high carrier mobility with a material with a narrow bandgap and a high optical absorption coefficient, can simultaneously achieve high responsivity and fast response time. Precise regulation of the thickness of each layer within the heterojunction can optimize the distribution and intensity of the built-in electric field and expedite the separation and transport of carriers. On the other hand, the response time of the photodetector is restricted by the bandwidth. When the bandwidth is increased by narrowing the width of the intrinsic region and downsizing the device, the light absorption of the material is affected, leading to a decrease in responsivity. The light propagation direction of the waveguide-coupled photodetector is perpendicular to the transmission direction of the carrier, reducing the mutual constraint between the response time and the responsivity. High responsivity and fast response speed of photodetectors can be optimized by preparing waveguide-coupled photodetectors.
All in all, heterolayered materials have great research potential in the field of infrared photodetection. While strides have been made in the development of infrared photodetectors using 2D materials, several challenges persist: (1) existing infrared photodetectors, despite their high sensitivity, encounter issues of signal interference and excessive background noise in certain environments. This compromises the accuracy and stability of these detectors. (2) The production of two-dimensional material infrared photodetectors presents its own set of challenges. The complex manufacturing process and high production costs hinder their large-scale application. (3) The requirements for infrared photodetectors vary across different fields, such as military, security, medical, and environmental protection. This necessitates the development of detectors with broad adaptability.
Data availability
No primary research results have been included and no new data were generated or analysed as part of this review.
Author contributions
Hao Gu and Tianshuo Zhang wrote the review and contributed equally. Yunluo Wang and Tianrui Zhou revised the manuscript. Haijie Chen developed the concept and edited this review.
Conflicts of interest
There are no conflicts to declare.
Acknowledgements
This work was supported by the National Natural Science Foundation of China (22101045).
References
- J. Cao, B. Jiang, H. Jiao, X. Niu, J. Zhang, Z. Zhang, X. Cheng and Z. Wang, A Dichroic Beamsplitter for the Laser Protection of Infrared Detectors, Coatings, 2022, 12, 1861 CrossRef CAS.
- T. Zhang, C. Ling, X. Wang, B. Feng, M. Cao, X. Xue, Q. Xue, J. Zhang, L. Zhu and C. Wang, Six-arm Stellat Dendritic-PbS Flexible Infrared Photodetector for Intelligent Healthcare Monitoring, Adv. Mater. Technol., 2022, 7, 2200250 CrossRef CAS.
- S. Kim, Y. T. Lim, E. G. Soltesz, A. M. De Grand, J. Lee, A. Nakayama, J. A. Parker, T. Mihaljevic, R. G. Laurence and D. M. Dor, Near-infrared fluorescent type II quantum dots for sentinel lymph node mapping, Nat. Biotechnol., 2004, 22, 93–97 CrossRef CAS PubMed.
- N. Ding, Y. Wu, W. Xu, J. Lyu, Y. Wang, L. Zi, L. Shao, R. Sun, N. Wang and S. Liu, A novel approach for designing efficient broadband photodetectors expanding from deep ultraviolet to near infrared, Light: Sci. Appl., 2022, 11, 91 CrossRef CAS PubMed.
- A. Rogalski, History of infrared detectors, Opto-Electron. Rev., 2012, 20, 279–308 Search PubMed.
-
E. Putley, Thermal detectors, Optical and Infrared Detectors, 2005, pp. 71–100 Search PubMed.
- W. Ma, Y. Gao, L. Shang, W. Zhou, N. Yao, L. Jiang, Q. Qiu, J. Li, Y. Shi, Z. Hu and Z. Huang, Ultrabroadband Tellurium Photoelectric Detector from Visible to Millimeter Wave, Adv. Sci., 2022, 9, 2103873 CrossRef CAS.
- L. Wang, C. Liu, X. Chen, J. Zhou, W. Hu, X. Wang, J. Li, W. Tang, A. Yu, S.-W. Wang and W. Lu, Toward Sensitive Room-Temperature Broadband Detection from Infrared to Terahertz with Antenna-Integrated Black Phosphorus Photoconductor, Adv. Funct. Mater., 2017, 27, 1604414 CrossRef.
- S. Bianconi and H. Mohseni, Recent advances in infrared imagers: toward thermodynamic and quantum limits of photon sensitivity, Rep. Prog. Phys., 2020, 83, 044101 CrossRef CAS PubMed.
- G. Rao, X. Wang, Y. Wang, P. Wangyang, C. Yan, J. Chu, L. Xue, C. Gong, J. Huang, J. Xiong and Y. Li, Two-dimensional heterostructure promoted infrared photodetection devices, InfoMat, 2019, 1, 272–288 CrossRef CAS.
- J. Yao and G. Yang, 2D material broadband photodetectors, Nanoscale, 2020, 12, 454–476 RSC.
- L. Zhang, N. Wang and Y. Li, Design, synthesis, and application of some two-dimensional materials, Chem. Sci., 2023, 14, 5266–5290 RSC.
- B. Y. Zhang, T. Liu, B. Meng, X. Li, G. Liang, X. Hu and Q. J. Wang, Broadband high photoresponse from pure monolayer graphene photodetector, Nat. Commun., 2013, 4, 1811 CrossRef.
- N. Youngblood, C. Chen, S. J. Koester and M. Li, Waveguide-integrated black phosphorus photodetector with high responsivity and low dark current, Nat. Photonics, 2015, 9, 247–252 CrossRef CAS.
- H. Wang, C. Zhang, W. Chan, S. Tiwari and F. Rana, Ultrafast response of monolayer molybdenum disulfide photodetectors, Nat. Commun., 2015, 6, 8831 CrossRef CAS PubMed.
- D. H. Kang, M. S. Kim, J. Shim, J. Jeon, H. Y. Park, W. S. Jung, H. Y. Yu, C. H. Pang, S. Lee and J. H. Park, High-performance transition metal dichalcogenide photodetectors enhanced by self-assembled monolayer doping, Adv. Funct. Mater., 2015, 25, 4219–4227 CrossRef CAS.
- L. Li, G. J. Ye, V. Tran, R. Fei, G. Chen, H. Wang, J. Wang, K. Watanabe, T. Taniguchi and L. Yang, Quantum oscillations in a two-dimensional electron gas in black phosphorus thin films, Nat. Nanotechnol., 2015, 10, 608–613 CrossRef.
- X. Chen, X. Lu, B. Deng, O. Sinai, Y. Shao, C. Li, S. Yuan, V. Tran, K. Watanabe, T. Taniguchi, D. Naveh, L. Yang and F. Xia, Widely tunable black phosphorus mid-infrared photodetector, Nat. Commun., 2017, 8, 1672 CrossRef PubMed.
- C. O. Kim, S. Kim, D. H. Shin, S. S. Kang, J. M. Kim, C. W. Jang, S. S. Joo, J. S. Lee, J. H. Kim, S.-H. Choi and E. Hwang, High photoresponsivity in an all-graphene p–n vertical junction photodetector, Nat. Commun., 2014, 5, 3249 CrossRef.
- C. K. Kanade, H. Seok, V. K. Kanade, K. Aydin, H.-U. Kim, S. B. Mitta, W. J. Yoo and T. Kim, Low-temperature and large-scale production of a transition metal sulfide vertical heterostructure and its application for photodetectors, ACS Appl. Mater. Interfaces, 2021, 13, 8710–8717 CrossRef CAS.
- A. P. Balan, A. B. Puthirath, S. Roy, G. Costin, E. F. Oliveira, M. A. S. R. Saadi, V. Sreepal, R. Friedrich, P. Serles, A. Biswas, S. A. Iyengar, N. Chakingal, S. Bhattacharyya, S. K. Saju, S. C. Pardo, L. M. Sassi, T. Filleter, A. Krasheninnikov, D. S. Galvao, R. Vajtai, R. R. Nair and P. M. Ajayan, Non-van der Waals quasi-2D materials; recent advances in synthesis, emergent properties and applications, Mater. Today, 2022, 58, 164–200 CrossRef CAS.
- P. Tian, H. Wu, L. Tang, J. Xiang, R. Ji, S. P. Lau, K. S. Teng, W. Guo, Y. Yao and L.-J. Li, Ultrasensitive broadband photodetectors based on two-dimensional Bi2O2Te films, J. Mater. Chem. C, 2021, 9, 13713–13721 RSC.
- Y. Y. Niu, D. Wu, Y. Q. Su, H. Zhu, B. Wang, Y. X. Wang, Z. R. Zhao, P. Zheng, J. S. Niu, H. B. Zhou, J. Wei and N. L. Wang, Uncooled EuSbTe3 photodetector highly sensitive from ultraviolet to terahertz frequencies, 2D Mater., 2017, 5, 011008 CrossRef.
- Y. Niu, B. Wang, J. Chen and D. Wu, Ultra-broadband and highly responsive photodetectors based on a novel EuBiTe3 flake material at room temperature, J. Mater. Chem. C, 2018, 6, 713–716 RSC.
- D. B. Velusamy, J. K. El-Demellawi, A. M. El-Zohry, A. Giugni, S. Lopatin, M. N. Hedhili, A. E. Mansour, E. D. Fabrizio, O. F. Mohammed and H. N. Alshareef, MXenes for Plasmonic Photodetection, Adv. Mater., 2019, 31, 1807658 CrossRef PubMed.
- Q. Qiu and Z. Huang, Photodetectors of 2D Materials from Ultraviolet to Terahertz Waves, Adv. Mater., 2021, 33, 2008126 CrossRef CAS PubMed.
- Z. Cheng, T. Zhao and H. Zeng, 2D Material-Based Photodetectors for Infrared Imaging, Small Sci., 2022, 2, 2100051 CrossRef CAS.
- J. Zha, M. Luo, M. Ye, T. Ahmed, X. Yu, D. H. Lien, Q. He, D. Lei, J. C. Ho and J. Bullock, Infrared photodetectors based on 2D materials and nanophotonics, Adv. Funct. Mater., 2022, 32, 2111970 CrossRef CAS.
- X. Guan, X. Yu, D. Periyanagounder, M. R. Benzigar, J. K. Huang, C. H. Lin, J. Kim, S. Singh, L. Hu and G. Liu, Recent progress in short-to long-wave infrared photodetection using 2D materials and heterostructures, Adv. Opt. Mater., 2021, 9, 2001708 CrossRef CAS.
- Y. Liu, X. Yi, N. J. Bailey, Z. Zhou, T. B. O. Rockett, L. W. Lim, C. H. Tan, R. D. Richards and J. P. R. David, Valence band engineering of GaAsBi for low noise avalanche photodiodes, Nat. Commun., 2021, 12, 4784 CrossRef CAS PubMed.
- J. Chen, J. Chen, X. Li, J. He, L. Yang, J. Wang, F. Yu, Z. Zhao, C. Shen, H. Guo, G. Li, X. Chen and W. Lu, High-performance HgCdTe avalanche photodetector enabled with suppression of band-to-band tunneling effect in mid-wavelength infrared, npj Quantum Mater., 2021, 6, 103 CrossRef CAS.
- J. Li, A. Dehzangi, G. Brown and M. Razeghi, Mid-wavelength infrared avalanche photodetector with AlAsSb/GaSb superlattice, Sci. Rep., 2021, 11, 7104 CrossRef CAS PubMed.
- G. Wu, R. Fu, J. Chen, W. Yang, J. Ren, X. Guo, Z. Ni, X. Pi, C. Z. Li and H. Li, Perovskite/Organic Bulk-Heterojunction Integrated Ultrasensitive Broadband Photodetectors with High Near-Infrared External Quantum Efficiency over 70%, Small, 2018, 14, 1802349 CrossRef.
- Q. Zhang, D. Liu, Z. Wang, P. Dang, H. Lian, G. Li and J. Lin, LaMgGa11O19: Cr3+, Ni2+ as blue-light excitable near-infrared luminescent materials with ultra-wide emission and high external quantum efficiency, Adv. Opt. Mater., 2023, 11, 2202478 CrossRef CAS.
- W. Yin, J. Yang, K. Zhao, A. Cui, J. Zhou, W. Tian, W. Li, Z. Hu and J. Chu, High responsivity and external quantum efficiency photodetectors based on solution-processed Ni-doped CuO films, ACS Appl. Mater. Interfaces, 2020, 12, 11797–11805 CrossRef CAS.
-
V. Mackowiak, J. Peupelmann, Y. Ma and A. Gorges, NEP – Noise Equivalent Power, Thorlabs, Inc, 2015, p. 56 Search PubMed.
- L. Huang, W. C. Tan, L. Wang, B. Dong, C. Lee and K.-W. Ang, Infrared black phosphorus phototransistor with tunable responsivity and low noise equivalent power, ACS Appl. Mater. Interfaces, 2017, 9, 36130–36136 CrossRef CAS.
- R. Tauk, F. Teppe, S. Boubanga, D. Coquillat, W. Knap, Y. Meziani, C. Gallon, F. Boeuf, T. Skotnicki and C. Fenouillet-Beranger, Plasma wave detection of terahertz radiation by silicon field effects transistors: Responsivity and noise equivalent power, Appl. Phys. Lett., 2006, 89, 253511 CrossRef.
- G. H. Shin, J. Park, K. J. Lee, G.-B. Lee, H. B. Jeon, Y.-K. Choi, K. Yu and S.-Y. Choi, Si–MoS2 vertical heterojunction for a photodetector with high responsivity and low noise equivalent power, ACS Appl. Mater. Interfaces, 2019, 11, 7626–7634 CrossRef CAS PubMed.
- T. Dong, J. Simões and Z. Yang, Flexible photodetector based on 2D materials: processing, architectures, and applications, Adv. Mater. Interfaces, 2020, 7, 1901657 CrossRef CAS.
- M. Long, P. Wang, H. Fang and W. Hu, Progress, Challenges, and Opportunities for 2D Material Based Photodetectors, Adv. Funct. Mater., 2019, 29, 1803807 CrossRef.
- T. Patel and A. W. Tsen, Stress testing the bulk photovoltaic effect, Nat. Nanotechnol., 2023, 18, 3–4 CrossRef CAS.
- C. Liu, J. Guo, L. Yu, J. Li, M. Zhang, H. Li, Y. Shi and D. Dai, Silicon/2D-material photodetectors: from near-infrared to mid-infrared, Light: Sci. Appl., 2021, 10, 123 CrossRef CAS PubMed.
- X. Lu, L. Sun, P. Jiang and X. Bao, Progress of Photodetectors Based on the Photothermoelectric Effect, Adv. Mater., 2019, 31, 1902044 CrossRef CAS PubMed.
- D. Basko, A photothermoelectric effect in graphene, Science, 2011, 334, 610–611 CrossRef CAS.
- X. Lu, P. Jiang and X. Bao, Phonon-enhanced photothermoelectric effect in SrTiO3 ultra-broadband photodetector, Nat. Commun., 2019, 10, 138 CrossRef PubMed.
- T. Case, Notes on the change of resistance of certain substances in light, Phys. Rev., 1917, 9, 305 CrossRef CAS.
- M. Casalino, G. Coppola, M. Iodice, I. Rendina and L. Sirleto, Near-infrared sub-bandgap all-silicon photodetectors: state of the art and perspectives, Sensors, 2010, 10, 10571–10600 CrossRef PubMed.
- W. Lei, J. Antoszewski and L. Faraone, Progress, challenges, and opportunities for HgCdTe infrared materials and detectors, Appl. Phys. Rev., 2015, 2, 041303 Search PubMed.
-
H. Yuan, J. Zhang, J. Kim, D. Bond, J. Laquindanum, J. Kimchi and M. G. DeForest, Recent progress in extended wavelength InGaAs photodetectors and comparison with SWIR HgCdTe photodetectors, Infrared Sensors, Devices, and Applications IX, 2019, vol. 11129, pp. 97–106 Search PubMed.
- P. Wang, H. Xia, Q. Li, F. Wang, L. Zhang, T. Li, P. Martyniuk, A. Rogalski and W. Hu, Sensing infrared photons at room temperature: from bulk materials to atomic layers, Small, 2019, 15, 1904396 CrossRef CAS.
- D. Gray, A. McCaughan and B. Mookerji, Crystal structure of graphite, graphene and silicon, Physics for Solid State Applications, 2009, 6, 730 Search PubMed.
- W. Yang, J. Chen, Y. Zhang, Y. Zhang, J. H. He and X. Fang, Silicon-compatible photodetectors: trends to monolithically integrate photosensors with chip technology, Adv. Funct. Mater., 2019, 29, 1808182 CrossRef.
- M. A. Green, Intrinsic concentration, effective densities of states, and effective mass in silicon, J. Appl. Phys., 1990, 67, 2944–2954 CrossRef CAS.
- M. A. Nazirzadeh, F. B. Atar, B. B. Turgut and A. K. Okyay, Random sized plasmonic nanoantennas on Silicon for low-cost broad-band near-infrared photodetection, Sci. Rep., 2014, 4, 7103 CrossRef PubMed.
- Y. Cui, Z. Tong, X. Zhang, W. Wang, W. Zhao, Y. Yu, X. Pi, J. Zhang and Z. Ni, Mid-infrared plasmonic silicon quantum dot/HgCdTe photodetector with ultrahigh specific detectivity, Sci. China Inf. Sci., 2023, 66, 142404 CrossRef.
- S. Park, J. Jeon, V. M. More, R. S. Lee, Y. Seo, M. Kim, P. D. Nguyen, M. Kim, J. S. Kim and Y. Kim, Monolithic two-color short-wavelength InGaAs infrared photodetectors using InAsP metamorphic buffers, Appl. Surf. Sci., 2022, 581, 152421 CrossRef CAS.
- Y. Xie, B. Zhang, S. Wang, D. Wang, A. Wang, Z. Wang, H. Yu, H. Zhang, Y. Chen and M. Zhao, Ultrabroadband MoS2 photodetector
with spectral response from 445 to 2717 nm, Adv. Mater., 2017, 29, 1605972 CrossRef.
- S. Huang, J. Cao, G. Song, J. Cao, Y. Lu, Q. Wu, W. Gao and J. Xu, Broadband-Spectral-Responsivity of black silicon photodetector with high gain and sub-bandgap sensitivity by titanium hyperdoping, Opt Laser. Technol., 2024, 171, 110399 CrossRef CAS.
- A. Rogalski, HgCdTe infrared detector material: history, status and outlook, Rep. Prog. Phys., 2005, 68, 2267 CrossRef CAS.
- I. B. Bhat, H. Ehsani and S. K. Ghandhi, The growth and characterization of HgTe and HgCdTe using methylalylltelluride, J. Vac. Sci. Technol., A, 1990, 8, 1054–1058 CrossRef CAS.
- X. Wang, M. Wang, Y. Liao, H. Zhang, B. Zhang, T. Wen, J. Yi and L. Qiao, Molecular-beam epitaxy-grown HgCdTe infrared detector: Material physics, structure design, and device fabrication, Sci. China: Phys., Mech. Astron., 2023, 66, 237302 CAS.
- J. Schmit, Growth, properties and applications of HgCdTe, J. Cryst. Growth, 1983, 65, 249–261 CrossRef CAS.
- Y. Wang, Y. Gu, A. Cui, Q. Li, T. He, K. Zhang, Z. Wang, Z. Li, Z. Zhang and P. Wu, Fast uncooled mid-wavelength infrared photodetectors with heterostructures of van der Waals on epitaxial HgCdTe, Adv. Mater., 2022, 34, 2107772 CrossRef CAS.
- H. Jiao, X. Wang, Y. Chen, S. Guo, S. Wu, C. Song, S. Huang, X. Huang, X. Tai and T. Lin, HgCdTe/black phosphorus van der Waals heterojunction for high-performance polarization-sensitive midwave infrared photodetector, Sci. Adv., 2022, 8, eabn1811 CrossRef CAS.
- S. An, H. Park and M. Kim, Recent advances in single crystal narrow band-gap semiconductor nanomembranes and their flexible optoelectronic device applications: Ge, GeSn, InGaAs, and 2D materials, J. Mater. Chem. C, 2023, 11, 2430–2448 RSC.
- W. Lee and C. G. Fonstad, The growth of high mobility InGaAs and InAlAs layers by molecular beam epitaxy, J. Vac. Sci. Technol., B: Microelectron. Process. Phenom., 1986, 4, 536–538 CrossRef CAS.
- X. Li, J. Zhang, C. Yue, X. Tang, Z. Gao, Y. Jiang, C. Du, Z. Deng, H. Jia and W. Wang, High performance visible-SWIR flexible photodetector based on large-area InGaAs/InP PIN structure, Sci. Rep., 2022, 12, 7681 CrossRef CAS.
- D. Kwak, D. K. Polyushkin and T. Mueller, In-sensor computing using a MoS2 photodetector with programmable spectral responsivity, Nat. Commun., 2023, 14, 4264 CrossRef CAS PubMed.
- Z. Xu, M. He, Q. Wu, C. Wu, X. Li, B. Liu, M. C. Tang, J. Yao and G. Wei, Ultrafast charge transfer 2D MoS2/organic heterojunction for sensitive photodetector, Adv. Sci., 2023, 10, 2207743 CrossRef CAS.
- H. Zhang, Ultrathin Two-Dimensional Nanomaterials, ACS Nano, 2015, 9, 9451–9469 CrossRef CAS PubMed.
- V. Tran, R. Soklaski, Y. Liang and L. Yang, Layer-controlled band gap and anisotropic excitons in few-layer black phosphorus, Phys. Rev. B: Condens. Matter Mater. Phys., 2014, 89, 235319 CrossRef.
- D.-S. Tsai, K.-K. Liu, D.-H. Lien, M.-L. Tsai, C.-F. Kang, C.-A. Lin, L.-J. Li and J.-H. He, Few-layer MoS2 with high broadband photogain and fast optical switching for use in harsh environments, ACS Nano, 2013, 7, 3905–3911 CrossRef CAS PubMed.
- Z.-S. Wu, W. Ren, L. Gao, J. Zhao, Z. Chen, B. Liu, D. Tang, B. Yu, C. Jiang and H.-M. Cheng, Synthesis of Graphene Sheets with High Electrical Conductivity and Good
Thermal Stability by Hydrogen Arc Discharge Exfoliation, ACS Nano, 2009, 3, 411–417 CrossRef CAS PubMed.
- A. K. Geim and K. S. Novoselov, The rise of graphene, Nat. Mater., 2007, 6, 183–191 CrossRef CAS PubMed.
- F. H. L. Koppens, T. Mueller, P. Avouris, A. C. Ferrari, M. S. Vitiello and M. Polini, Photodetectors based on graphene, other two-dimensional materials and hybrid systems, Nat. Nanotechnol., 2014, 9, 780–793 CrossRef CAS PubMed.
- G. Konstantatos, M. Badioli, L. Gaudreau, J. Osmond, M. Bernechea, F. Pelayo Garcia de Arquer, F. Gatti and F. H. L. Koppens, Hybrid graphene–quantum dot phototransistors with ultrahigh gain, Nat. Nanotechnol., 2021, 7, 363–368 CrossRef.
- J. Park, Y. Ahn and C. Ruiz-Vargas, Imaging of photocurrent generation and collection in single-layer graphene, Nano Lett., 2009, 9, 1742–1746 CrossRef CAS.
- F. Xia, T. Mueller, R. Golizadeh-Mojarad, M. Freitag, Y.-m. Lin, J. Tsang, V. Perebeinos and P. Avouris, Photocurrent imaging and efficient photon detection in a graphene transistor, Nano Lett., 2009, 9, 1039–1044 CrossRef CAS PubMed.
- C.-H. Liu, Y.-C. Chang, T. B. Norris and Z. Zhong, Graphene photodetectors with ultra-broadband and high responsivity at room temperature, Nat. Nanotechnol., 2014, 9, 273–278 CrossRef CAS PubMed.
- D. Zahn, P.-N. Hildebrandt, T. Vasileiadis, Y. W. Windsor, Y. Qi, H. L. N. Seiler and R. Ernstorfer, Anisotropic nonequilibrium lattice dynamics of black phosphorus, Nano Lett., 2020, 20, 3728–3733 CrossRef CAS.
- M. Buscema, D. J. Groenendijk, S. I. Blanter, G. A. Steele, H. S. van der Zant and A. Castellanos-Gomez, Fast and broadband photoresponse of few-layer black phosphorus field-effect transistors, Nano Lett., 2014, 14, 3347–3352 CrossRef CAS PubMed.
- R. Gusmao, Z. Sofer and M. Pumera, Black phosphorus rediscovered: from bulk material to monolayers, Angew. Chem., Int. Ed., 2017, 56, 8052–8072 CrossRef CAS PubMed.
- Z. Hu, T. Niu, R. Guo, J. Zhang, M. Lai, J. He, L. Wang and W. Chen, Two-dimensional black phosphorus: its fabrication, functionalization and applications, Nanoscale, 2018, 10, 21575–21603 RSC.
- Q. Guo, A. Pospischil, M. Bhuiyan, H. Jiang, H. Tian, D. Farmer, B. Deng, C. Li, S.-J. Han and H. Wang, Black phosphorus mid-infrared photodetectors with high gain, Nano Lett., 2016, 16, 4648–4655 CrossRef CAS PubMed.
- D. Kong, H. Wang, J. J. Cha, M. Pasta, K. J. Koski, J. Yao and Y. Cui, Synthesis of MoS2 and MoSe2 films with vertically aligned layers, Nano Lett., 2013, 13, 1341–1347 CrossRef CAS PubMed.
- H. Wang, C. Li, P. Fang, Z. Zhang and J. Z. Zhang, Synthesis, properties, and optoelectronic applications of two-dimensional MoS 2 and MoS 2-based heterostructures, Chem. Soc. Rev., 2018, 47, 6101–6127 RSC.
- B. Cao, Z. Ye, L. Yang, L. Gou and Z. Wang, Recent progress in van der Waals 2D PtSe2, Nanotechnology, 2021, 32, 412001 CrossRef CAS PubMed.
- S. Liu and Z. Liu, Hybridization induced metallic and magnetic edge states in noble transition-metal-dichalcogenides of PtX 2 (X= S, Se) nanoribbons, Phys. Chem. Chem. Phys., 2018, 20, 21441–21446 RSC.
- H. Zhang, H. Li, F. Wang, X. Song, Z. Xu, D. Wei, J. Zhang, Z. Dai, Y. Ren and Y. Ye, PtSe2 field-effect phototransistor with positive and negative photoconductivity, ACS Appl. Electron. Mater., 2022, 4, 5177–5183 CrossRef CAS.
- P. Ye, H. Xiao, Q. Zhu, Y. Kong, Y. Tang and M. Xu, Si-CMOS-compatible 2D PtSe2-based self-driven photodetector with ultrahigh responsivity and specific detectivity, Sci. China Mater., 2023, 66, 193–201 CrossRef CAS.
- J. Deng, W. Zhang, X. Dai, Y. Yu, Z. Li, W. Wang, L. Wang, J. Zhou and X. Chen, Nonmonotonic wavelength dependence of the polarization-sensitive infrared photoresponse of an anisotropic semimetal, Nanoscale, 2022, 14, 7314–7321 RSC.
- Y. Yi, C. Wu, H. Wang, H. Liu, H. Li, H. Zhang, H. He and J. Wang, Thickness dependent magneto transport properties of WTe2 thin films, Solid State Commun., 2017, 260, 45–49 CrossRef CAS.
- Y. Xiao, K. Luo, Q. Kao, Y. Fu, W. Jiang and L. Cao, Photoelectric properties of large area WTe2 thin films prepared by pulsed laser deposition, Surf. Interfaces, 2024, 44, 103670 CrossRef CAS.
- F. Wang, Y. Zhang, Y. Gao, P. Luo, J. Su, W. Han, K. Liu, H. Li and T. Zhai, 2D metal chalcogenides for IR photodetection, Small, 2019, 15, 1901347 CrossRef PubMed.
- S. Sorifi, M. Moun, S. Kaushik and R. Singh, High-temperature performance of a GaSe nanosheet-based broadband photodetector, ACS Appl. Electron. Mater., 2020, 2, 670–676 CrossRef CAS.
- Z. Li, H. Qiao, Z. Guo, X. Ren, Z. Huang, X. Qi, S. C. Dhanabalan, J. S. Ponraj, D. Zhang and J. Li, High-performance photo-electrochemical photodetector based on liquid-exfoliated few-layered InSe nanosheets with enhanced stability, Adv. Funct. Mater., 2018, 28, 1705237 CrossRef.
- W. Gao, S. Zhang, F. Zhang, P. Wen, L. Zhang, Y. Sun, H. Chen, Z. Zheng, M. Yang and D. Luo, 2D WS2 based asymmetric Schottky photodetector with high performance, Adv. Electron. Mater., 2021, 7, 2000964 CrossRef CAS.
- F. Yan, L. Zhao, A. Patanè, P. Hu, X. Wei, W. Luo, D. Zhang, Q. Lv, Q. Feng and C. Shen, Fast, multicolor photodetection with graphene-contacted p-GaSe/n-InSe van der Waals heterostructures, Nanotechnology, 2017, 28, 27LT01 CrossRef PubMed.
- Q. Lv, F. Yan, X. Wei and K. Wang, High-performance, self-driven photodetector based on graphene sandwiched GaSe/WS2 heterojunction, Adv. Opt. Mater., 2018, 6, 1700490 CrossRef.
- W. Zhu, X. Wei, F. Yan, Q. Lv, C. Hu and K. Wang, Broadband polarized photodetector based on p-BP/n-ReS2 heterojunction, J. Semicond., 2019, 40, 092001 CrossRef CAS.
- I. Tantis, S. Talande, V. Tzitzios, G. Basina, V. Shrivastav, A. Bakandritsos and R. Zboril, Non-van der Waals 2D Materials for Electrochemical Energy Storage, Adv. Funct. Mater., 2023, 33, 2209360 CrossRef CAS.
- H. Chen, J. N. B. Rodrigues, A. J. E. Rettie, T.-B. Song, D. G. Chica, X. Su, J.-K. Bao, D. Y. Chung, W.-K. Kwok, L. K. Wagner and M. G. Kanatzidis, High Hole Mobility and Nonsaturating Giant Magnetoresistance in the New 2D Metal NaCu4Se4 Synthesized by a Unique Pathway, J. Am. Chem. Soc., 2019, 141, 635–642 CrossRef CAS PubMed.
- J.-K. Bao, C. D. Malliakas, C. Zhang, S. Cai, H. Chen, A. J. E. Rettie, B. L. Fisher, D. Y. Chung, V. P. Dravid and M. G. Kanatzidis, Quasi-Two-Dimensional Heterostructures (KM1 – xTe)(LaTe3) (M = Mn and Zn) with Charge Density Waves, Chem. Mater., 2021, 33, 2155–2164 CrossRef CAS.
- H. Chen, J. He, C. D. Malliakas, C. C. Stoumpos, A. J. E. Rettie, J.-K. Bao, D. Y. Chung, W.-K. Kwok, C. Wolverton and M. G. Kanatzidis, A Natural 2D Heterostructure [Pb3.1Sb0.9S4][AuxTe2–x] with Large Transverse Nonsaturating Negative Magnetoresistance and High Electron Mobility, J. Am. Chem. Soc., 2019, 141, 7544–7553 CrossRef CAS PubMed.
- M. P. Smylie, K. Willa, J. K. Bao, K. Ryan, Z. Islam, H. Claus, Y. Simsek, Z. Diao, A. Rydh, A. E. Koshelev, W. K. Kwok, D. Y. Chung, M. G. Kanatzidis and U. Welp, Anisotropic superconductivity and magnetism in single-crystals RbEuFe4As4, Phys. Rev. B, 2018, 98, 104503 CrossRef CAS.
- Q. Wei, R. Li, C. Lin, A. Han, A. Nie, Y. Li, L.-J. Li, Y. Cheng and W. Huang, Quasi-Two-Dimensional Se-Terminated Bismuth Oxychalcogenide (Bi2O2Se), ACS Nano, 2019, 13, 13439–13444 CrossRef CAS PubMed.
- Y. Y. Niu, D. Wu, L. Shen and B. Wang, A layered antiferromagnetic semiconductor EuMTe3 (M = Bi, Sb), Phys. Status Solidi RRL, 2015, 9, 735–739 CrossRef CAS.
- H. Xu, A. Ren, J. Wu and Z. Wang, Recent Advances in 2D MXenes for Photodetection, Adv. Funct. Mater., 2020, 30, 2000907 CrossRef CAS.
- J. Wu, H. Yuan, M. Meng, C. Chen, Y. Sun, Z. Chen, W. Dang, C. Tan, Y. Liu, J. Yin, Y. Zhou, S. Huang, H. Q. Xu, Y. Cui, H. Y. Hwang, Z. Liu, Y. Chen, B. Yan and H. Peng, High electron mobility and quantum oscillations in non-encapsulated ultrathin semiconducting Bi2O2Se, Nat. Nanotechnol., 2017, 12, 530–534 CrossRef CAS PubMed.
- T. Tu, Y. Zhang, T. Li, J. Yu, L. Liu, J. Wu, C. Tan, J. Tang, Y. Liang, C. Zhang, Y. Dai, Y. Han, K. Lai and H. Peng, Uniform High-k Amorphous Native Oxide Synthesized by Oxygen Plasma for Top-Gated Transistors, Nano Lett., 2020, 20, 7469–7475 CrossRef CAS.
- X. Ma, D. Chang, C. Zhao, R. Li, X. Huang, Z. Zeng, X. Huang and Y. Jia, Geometric structures and electronic properties of the Bi2X2Y (X, Y = O, S, Se, and Te) ternary compound family: a systematic DFT study, J. Mater. Chem. C, 2018, 6, 13241–13249 RSC.
- Y.-D. Xu, C. Wang, Y.-Y. Lv, Y. B. Chen, S.-H. Yao and J. Zhou, Infrared and Raman spectra of Bi2O2X and Bi2OX2 (X = S, Se, and Te) studied from first principles calculations, RSC Adv., 2019, 9, 18042–18049 RSC.
- H. Kim and H. N. Alshareef, MXetronics: MXene-enabled electronic and photonic devices, ACS Mater. Lett., 2019, 2, 55–70 CrossRef.
- Z. Guo, Z. Xu, S. Teo, C. Zhang, Y. Kamata, S. Hayase, T. Ma and L. Gao, High Electrical Conductivity 2D MXene Serves as Additive of Perovskite for Efficient Solar Cells, Small, 2018, 14, 1802738 CrossRef PubMed.
- N. M. Abbasi, Y. Xiao, L. Peng, Y. Duo, L. Wang, L. Zhang, B. Wang and H. Zhang, Recent advancement for the synthesis of MXene derivatives and their sensing protocol, Adv. Mater. Technol., 2021, 6, 2001197 CrossRef CAS.
- L. Shen, H. Qian, Y. Yang, Y. Ma, J. Deng and Y. Zhang, Photoresponse Improvement of InGaAs Nanowire Near-Infrared Photodetectors with Self-Assembled Monolayers, J. Phys. Chem. C, 2023, 127, 11328–11337 CrossRef CAS.
- M.-Q. Li, L.-Y. Dang, G.-G. Wang, F. Li, M. Han, Z.-P. Wu, G.-Z. Li, Z. Liu and J.-C. Han, Bismuth Oxychalcogenide Nanosheet: Facile Synthesis, Characterization, and Photodetector Application, Adv. Mater. Technol., 2020, 5, 2000180 CrossRef CAS.
- K. Wang, H. Qiao, J. Li and X. Qi, A robust photoelectrochemical photodetectors based on the self-healing properties of Bi2O2S nanoplates, Appl. Surf. Sci., 2021, 565, 150444 CrossRef CAS.
- P. Rong, S. Gao, M. Zhang, S. Ren, H. Lu, J. Jia, S. Jiao, Y. Zhang and J. Wang, Large-area hierarchical Bi2O2S flowers composed of 2D ultrathin nanosheets for high performance self-powered IR photodetector, J. Alloys Compd., 2022, 928, 167128 CrossRef CAS.
- J. Yin, Z. Tan, H. Hong, J. Wu, H. Yuan, Y. Liu, C. Chen, C. Tan, F. Yao, T. Li, Y. Chen, Z. Liu, K. Liu and H. Peng, Ultrafast and highly sensitive infrared photodetectors based on two-dimensional oxyselenide crystals, Nat. Commun., 2018, 9, 3311 CrossRef.
- Y. Chen, W. Ma, C. Tan, M. Luo, W. Zhou, N. Yao, H. Wang, L. Zhang, T. Xu, T. Tong, Y. Zhou, Y. Xu, C. Yu, C. Shan, H. Peng, F. Yue, P. Wang, Z. Huang and W. Hu, Broadband Bi2O2Se Photodetectors from Infrared to Terahertz, Adv. Funct. Mater., 2021, 31, 2009554 CrossRef CAS.
- Y. Wei, C. Chen, C. Tan, L. He, Z. Ren, C. Zhang, S. Peng, J. Han, H. Zhou and J. Wang, High-Performance Visible to Near-Infrared Broadband Bi2O2Se Nanoribbon Photodetectors, Adv. Opt. Mater., 2022, 10, 2201396 CrossRef CAS.
- X. Zou, R. Wang, Y. Sun and C. Wang, Two-dimensional Bi2O2Se nanosheets for sensitive and fast-response high-temperature photodetectors, J. Materiomics, 2023, 9, 1024–1031 CrossRef.
- C. Hu, Z. Du, Z. Wei, L. Li and G. Shen, Functionalized Ti3C2Tx MXene with layer-dependent band gap for flexible NIR photodetectors, Appl. Phys. Rev., 2023, 10, 021402 CAS.
- C. Hu, Z. Wei, L. Li and G. Shen, Strategy Toward Semiconducting Ti3C2Tx-MXene: Phenylsulfonic Acid Groups Modified Ti3C2Tx as Photosensitive Material for Flexible Visual Sensory-Neuromorphic System, Adv. Funct. Mater., 2023, 33, 2302188 CrossRef CAS.
- W. Yu, S. Li, Y. Zhang, W. Ma, T. Sun, J. Yuan, K. Fu and Q. Bao, Near-infrared photodetectors based on MoTe2/graphene heterostructure with high responsivity and flexibility, Small, 2017, 13, 1700268 CrossRef PubMed.
- F. Luo, M. Zhu, H. Sun, W. Luo, G. Peng, Z. Zhu, X.-A. Zhang and S. Qin, High responsivity graphene photodetectors from visible to near-infrared by photogating effect, AIP Adv., 2018, 8, 115106 CrossRef.
- S. N. S. Yadav, P. L. Chen, C. H. Liu and T. J. Yen, Plasmonic Metasurface Integrated Black Phosphorus-Based Mid-Infrared Photodetector with High Responsivity and Speed, Adv. Mater. Interfaces, 2023, 10, 2202403 CrossRef CAS.
- C. Wang, Q. Wu, Y. Ding, X. Zhang, W. Wang, X. Guo, Z. Ni, L. Lin, Z. Cai and X. Gu, High-responsivity and broadband MoS2 photodetector using interfacial engineering, ACS Appl. Mater. Interfaces, 2023, 15, 46236–46246 CrossRef CAS PubMed.
- C. Hu, Z. Wei, L. Li and G. Shen, Strategy Toward Semiconducting Ti3C2Tx-MXene: Phenylsulfonic Acid Groups Modified Ti3C2Tx as Photosensitive Material for Flexible Visual Sensory-Neuromorphic System, Adv. Funct. Mater., 2023, 33, 2302188 CrossRef CAS.
- S. D. N. Luu and P. Vaqueiro, Synthesis, characterisation and thermoelectric properties of the oxytelluride Bi2O2Te, J. Solid State Chem., 2015, 226, 219–223 CrossRef CAS.
- Q. Lin, Z. Yu, L. Lu, X. Huang, Q. Wei and D. Tang, Smartphone-based photoelectrochemical immunoassay of prostate-specific antigen based on Co-doped Bi2O2S nanosheets, Biosens. Bioelectron., 2023, 230, 115260 CrossRef CAS PubMed.
- C. Huang, H. Yu, J. Chen, J. Zhang, Z. Wu and C. Hou, Improved performance of polymer solar cells by doping with Bi2O2S nanocrystals, Sol. Energy Mater. Sol. Cells, 2019, 200, 110030 CrossRef CAS.
- X. Zou, H. Liang, Y. Li, Y. Zou, F. Tian, Y. Sun and C. Wang, 2D Bi2O2Te Semiconductor with Single-Crystal Native Oxide Layer, Adv. Funct. Mater., 2023, 33, 2213807 CrossRef CAS.
- X. Zhang, Y. Liu, G. Zhang, Y. Wang, H. Zhang and F. Huang, Thermal Decomposition of Bismuth Oxysulfide from Photoelectric Bi2O2S to Superconducting Bi4O4S3, ACS Appl. Mater. Interfaces, 2015, 7, 4442–4448 CrossRef CAS PubMed.
- M. Wu and X. C. Zeng, Bismuth Oxychalcogenides: A New Class of Ferroelectric/Ferroelastic Materials with Ultra High Mobility, Nano Lett., 2017, 17, 6309–6314 CrossRef CAS PubMed.
- T. Ghosh, M. Samanta, A. Vasdev, K. Dolui, J. Ghatak, T. Das, G. Sheet and K. Biswas, Ultrathin Free-Standing Nanosheets of Bi2O2Se: Room Temperature Ferroelectricity in Self-Assembled Charged Layered Heterostructure, Nano Lett., 2019, 19, 5703–5709 CrossRef CAS PubMed.
- W. Wang, Y. Meng, Y. Zhang, Z. Zhang, W. Wang, Z. Lai, P. Xie, D. Li, D. Chen, Q. Quan, D. Yin, C. Liu, Z. Yang, S. Yip and J. C. Ho, Electrically Switchable Polarization in Bi2O2Se Ferroelectric Semiconductors, Adv. Mater., 2023, 35, 2210854 CrossRef CAS.
- F. Wang, S. Yang, J. Wu, X. Hu, Y. Li, H. Li, X. Liu, J. Luo and T. Zhai, Emerging two-dimensional bismuth oxychalcogenides for electronics and optoelectronics, InfoMat, 2021, 3, 1251–1271 CrossRef CAS.
- J. Dong, L. Zhang, K. Lau, Y. Shu, S. Wang, Z. Fu, Z. Wu, X. Liu, B. Sa, J. Pei, J. Zheng, H. Zhan and Q. Wang, Tailoring Broadband Nonlinear Optical Characteristics and Ultrafast Photocarrier Dynamics of Bi2O2S Nanosheets by Defect Engineering, Small, 2024, 20, 2309595 CrossRef CAS PubMed.
- L. Xu, S. Liu, H. Zhang, X. Zhang, J. Li, J. Yan, B. Shi, J. Yang, C. Yang, L. Xu, X. Sun and J. Lu, First-principles simulation of monolayer hydrogen passivated Bi2O2S2–metal interfaces, Phys. Chem. Chem. Phys., 2020, 22, 7853–7863 RSC.
- Y. Sun, J. Zhang, S. Ye, J. Song and J. Qu, Progress Report on Property, Preparation, and Application of Bi2O2Se, Adv. Funct. Mater., 2020, 30, 2004480 CrossRef CAS.
- S. Liu, C. Tan, D. He, Y. Wang, H. Peng and H. Zhao, Optical Properties and Photocarrier Dynamics of Bi2O2Se Monolayer and Nanoplates, Adv. Opt. Mater., 2020, 8, 1901567 CrossRef CAS.
- C. Chen, M. Wang, J. Wu, H. Fu, H. Yang, Z. Tian, T. Tu, H. Peng, Y. Sun, X. Xu, J. Jiang, N. B. M. Schröter, Y. Li, D. Pei, S. Liu, S. A. Ekahana, H. Yuan, J. Xue, G. Li, J. Jia, Z. Liu, B. Yan, H. Peng and Y. Chen, Electronic structures and unusually robust bandgap in an ultrahigh-mobility layered oxide semiconductor, Bi2O2Se, Sci. Adv., 2018, 4, eaat8355 CrossRef PubMed.
- T. Tong, M. Zhang, Y. Chen, Y. Li, L. Chen, J. Zhang, F. Song, X. Wang, W. Zou, Y. Xu and R. Zhang, Ultrahigh Hall mobility and suppressed backward scattering in layered semiconductor Bi2O2Se, Appl. Phys. Lett., 2018, 113, 072106 CrossRef.
- G. Wang, F. Liu, R. Chen, M. Wang, Y. Yin, J. Zhang, Z. Sa, P. Li, J. Wan, L. Sun, Z. Lv, Y. Tan, F. Chen and Z.-x. Yang, Tunable Contacts of Bi2O2Se Nanosheets MSM Photodetectors by Metal-Assisted Transfer Approach for Self-Powered Near-Infrared Photodetection, Small, 2024, 20, 2306363 CrossRef CAS PubMed.
- Z. Zhang, L. Han, Z. Dan, H. Li, M. Yang, Y. Sun, Z. Zheng, N. Huo, D. Luo, W. Gao and J. Li, Type II Homo-Type Bi2O2Se Nanosheet/InSe Nanoflake Heterostructures for Self-Driven Broadband Visible–Near-Infrared Photodetectors, ACS Appl. Nano Mater., 2023, 6, 4573–4583 CrossRef CAS.
- L. Zhang, Y. He, X. Dong, J. Guo, Z. Gao, Z. Liu, J. Chen, Y. Zhao, Z. Zhou, J. Yin, X. Fu, F. Luo, H. Fu and J. Wu, Controlled Synthesis of a High-Mobility Bi3O2.5Se2 Semiconductor by Oxidation of Bi2Se3 for Fast and Highly Sensitive Photodetectors, Laser Photonics Rev., 2024, 18, 2300854 CrossRef CAS.
- P. Luo, F. Wang, J. Qu, K. Liu, X. Hu, K. Liu and T. Zhai, Self-Driven WSe2/Bi2O2Se Van der Waals Heterostructure Photodetectors with High Light On/Off Ratio and Fast Response, Adv. Funct. Mater., 2021, 31, 2008351 CrossRef CAS.
- J. Li, Z. Wang, Y. Wen, J. Chu, L. Yin, R. Cheng, L. Lei, P. He, C. Jiang, L. Feng and J. He, High-Performance Near-Infrared Photodetector Based on Ultrathin Bi2O2Se Nanosheets, Adv. Funct. Mater., 2018, 28, 1706437 CrossRef.
- P. Luo, F. Zhuge, F. Wang, L. Lian, K. Liu, J. Zhang and T. Zhai, PbSe Quantum Dots Sensitized High-Mobility Bi2O2Se Nanosheets for High-Performance and Broadband Photodetection Beyond 2 μm, ACS Nano, 2019, 13, 9028–9037 CrossRef CAS PubMed.
- C. Tan, S. Xu, Z. Tan, L. Sun, J. Wu, T. Li and H. Peng, Exploitation of Bi2O2Se/graphene van der Waals heterojunction for creating efficient photodetectors and short-channel field-effect transistors, InfoMat, 2019, 1, 390–395 CrossRef CAS.
- S. Yang, S. Jiao, Y. Nie, Y. Zhao, S. Gao, D. Wang and J. Wang, A high-performance and self-powered polarization-sensitive photoelectrochemical-type Bi2O2Te photodetector based on a quasi-solid-state gel electrolyte, Mater. Horiz., 2024, 11, 1710–1718 RSC.
- Z. Hui, X. Bu, Y. Wang, D. Han, J. Gong, L. Li, X. Li and S. Yan, Bi2O2Te Nanosheets Saturable Absorber-Based Passive Mode-Locked Fiber Laser: From Soliton Molecules to Harmonic Soliton, Adv. Opt. Mater., 2022, 10, 2201812 CrossRef CAS.
- J.-X. Chen, X.-G. Zhao, X.-X. Dong, Z.-L. Lv and H.-L. Cui, Density Functional Study of the Electronic, Elastic and Optical Properties of Bi2O2Te, Z. Naturforsch. A, 2020, 75, 73–80 CrossRef CAS.
- W. Ai, J. Chen, X. Dong, Z. Gao, Y. He, Z. Liu, H. Fu, F. Luo and J. Wu, High Mobility and Quantum Oscillations in Semiconducting Bi2O2Te Nanosheets Grown by Chemical Vapor Deposition, Nano Lett., 2022, 22, 7659–7666 CrossRef CAS PubMed.
- M. Han, D. Zhang, C. E. Shuck, B. McBride, T. Zhang, R. Wang, K. Shevchuk and Y. Gogotsi, Electrochemically modulated interaction of MXenes with microwaves, Nat. Nanotechnol., 2023, 18, 373–379 CrossRef CAS PubMed.
- C. Zhang and V. Nicolosi, Graphene and MXene-based transparent conductive electrodes and supercapacitors, Energy Storage Mater., 2019, 16, 102–125 CrossRef.
- C. Zhang, B. Anasori, A. Seral-Ascaso, S.-H. Park, N. McEvoy, A. Shmeliov, G. S. Duesberg, J. N. Coleman, Y. Gogotsi and V. Nicolosi, Transparent, Flexible, and Conductive 2D Titanium Carbide (MXene) Films with High Volumetric Capacitance, Adv. Mater., 2017, 29, 1702678 CrossRef PubMed.
- K. Hantanasirisakul, M.-Q. Zhao, P. Urbankowski, J. Halim, B. Anasori, S. Kota, C. E. Ren, M. W. Barsoum and Y. Gogotsi, Fabrication of Ti3C2Tx MXene Transparent Thin Films with Tunable Optoelectronic Properties, Adv. Electron. Mater., 2016, 2, 1600050 CrossRef.
- B. Aïssa, A. Ali, K. A. Mahmoud, T. Haddad and M. Nedil, Transport properties of a highly conductive 2D Ti3C2Tx MXene/graphene composite, Appl. Phys. Lett., 2016, 109, 043109 CrossRef.
- K. Montazeri, M. Currie, L. Verger, P. Dianat, M. W. Barsoum and B. Nabet, Beyond Gold: Spin-Coated Ti3C2-Based MXene Photodetectors, Adv. Mater., 2019, 31, 1903271 CrossRef CAS PubMed.
- K. Montazeri, M. Currie, M. W. Barsoum and B. Nabet, Ultra-High Speed, High-Sensitivity Spin-Cast MXene-Semiconductor-MXene Photodetectors, Adv. Funct. Mater., 2022, 32, 2206942 CrossRef CAS.
- W. Song, Q. Liu, J. Chen, Z. Chen, X. He, Q. Zeng, S. Li, L. He, Z. Chen and X. Fang, Interface Engineering Ti3C2 MXene/Silicon Self-Powered Photodetectors with High Responsivity and Detectivity for Weak Light Applications, Small, 2021, 17, 2100439 CrossRef CAS PubMed.
- L. Luo, Y. Huang, K. Cheng, A. Alhassan, M. Alqahtani, L. Tang, Z. Wang and J. Wu, MXene-GaN van der Waals metal-semiconductor junctions for high performance multiple quantum well photodetectors, Light: Sci. Appl., 2021, 10, 177 CrossRef CAS PubMed.
- L. Gao, Y. Zhao, X. Chang, J. Zhang, Y. Li, S. Wageh, O. A. Al-Hartomy, A. G. Al-Sehemi, H. Zhang and H. Ågren, Emerging applications of MXenes for photodetection: Recent advances and future challenges, Mater. Today, 2022, 61, 169–190 CrossRef CAS.
- B. Li, Q.-B. Zhu, C. Cui, C. Liu, Z.-H. Wang, S. Feng, Y. Sun, H.-L. Zhu, X. Su, Y.-M. Zhao, H.-W. Zhang, J. Yao, S. Qiu, Q.-W. Li, X.-M. Wang, X.-H. Wang, H.-M. Cheng and D.-M. Sun, Patterning of Wafer-Scale MXene Films for High-Performance Image Sensor Arrays, Adv. Mater., 2022, 34, 2201298 CrossRef CAS PubMed.
- M. Naguib, M. W. Barsoum and Y. Gogotsi, Ten years of progress in the synthesis and development of MXenes, Adv. Mater., 2021, 33, 2103393 CrossRef CAS PubMed.
- D. H. Ho, Y. Y. Choi, S. B. Jo, J. M. Myoung and J. H. Cho, Sensing with MXenes: progress and prospects, Adv. Mater., 2021, 33, 2005846 CrossRef CAS PubMed.
- B. Anasori, M. R. Lukatskaya and Y. Gogotsi, 2D metal carbides and nitrides (MXenes) for energy storage, Nat. Rev. Mater., 2017, 2, 16098 CrossRef CAS.
- M. Naguib, M. Kurtoglu, V. Presser, J. Lu, J. Niu, M. Heon, L. Hultman, Y. Gogotsi and M. W. Barsoum, Two-Dimensional Nanocrystals Produced by Exfoliation of Ti3AlC2, Adv. Mater., 2011, 23, 4248–4253 CrossRef CAS PubMed.
- Y. Di, K. Ba, Y. Chen, X. Wang, M. Zhang, X. Huang, Y. Long, M. Liu, S. Zhang, W. Tang, Z. Huang, T. Lin, H. Shen, X. Meng, M. Han, Q. Liu and J. Wang, Interface Engineering to Drive High-Performance MXene/PbS Quantum Dot NIR Photodiode, Adv. Sci., 2024, 11, 2307169 CrossRef CAS PubMed.
- A. D. Dillon, M. J. Ghidiu, A. L. Krick, J. Griggs, S. J. May, Y. Gogotsi, M. W. Barsoum and A. T. Fafarman, Highly Conductive Optical Quality Solution-Processed Films of 2D Titanium Carbide, Adv. Funct. Mater., 2016, 26, 4162–4168 CrossRef CAS.
- B. Liu, L. Qian, Y. Zhao, Y. Zhang, F. Liu, Y. Zhang, Y. Xie and W. Shi, A polarization-sensitive, self-powered, broadband and fast Ti3C2T x MXene photodetector from visible to near-infrared driven by photogalvanic effects, Front. Phys., 2022, 17, 53501 CrossRef.
- M. Yousefi, P. Ghaffari, R. Nosrati, S. Dehghani, A. Salmaninejad, Y. J. Abarghan and S. H. Ghaffari, Prognostic and therapeutic significance of circulating tumor cells in patients with lung cancer, Cell. Oncol., 2020, 43, 31–49 CrossRef PubMed.
- S. Sun, S. Yang, X. Hu, C. Zheng, H. Song, L. Wang, Z. Shen and Z.-S. Wu, Combination of immunomagnetic separation with aptamer-mediated double rolling circle amplification for highly sensitive circulating tumor cell detection, ACS Sens., 2020, 5, 3870–3878 CrossRef CAS PubMed.
- P. Gopinathan, N.-J. Chiang, C.-H. Wang, A. Sinha, Y.-C. Tsai, H.-C. Tu, S.-C. Hung, P.-H. Hsu, Y.-S. Shan and G.-B. Lee, Aptamer probed isolation of circulating tumor cells in cholangiocarcinoma patients, Sens. Actuators, B, 2020, 322, 128569 CrossRef CAS.
- M. Zhang, C. Wang, C. Yang, H. Wu, H. Xu and G. Liang, Using fluorescence on/off to trace tandem nanofiber assembly/disassembly in living cells, Anal. Chem., 2021, 93, 5665–5669 CrossRef CAS.
- C. Ding, C. Zhang, X. Yin, X. Cao, M. Cai and Y. Xian, Near-infrared fluorescent Ag2S nanodot-based signal amplification for efficient detection of circulating tumor cells, Anal. Chem., 2018, 90, 6702–6709 CrossRef CAS PubMed.
- B. Zhang, H. Wang, J. Xi, F. Zhao and B. Zeng, A novel Z-scheme ZnIn2S4/WO3 photocatalyst based photoelectrochemical immunosensor for the sensitive detection of prostate specific antigen, Sens. Actuators, B, 2019, 298, 126835 CrossRef CAS.
- X. Xu, Z. Ding, X. Zhang, R. Zha, W. Li, L. Xu, D. Sun, X. Cai, T. Liang and Y. Wang, A near-infrared photoelectrochemical aptasensing system based on Bi2O2S nanoflowers and gold nanoparticles for high-performance determination of MCF-7ácells, Anal. Chim. Acta, 2023, 1251, 340982 CrossRef CAS PubMed.
- C. Hu, H. Chen, L. Li, H. Huang and G. Shen, Ti3C2Tx MXene-RAN van der Waals Heterostructure-Based Flexible Transparent NIR Photodetector Array for 1024 Pixel Image Sensing Application, Adv. Mater. Technol., 2022, 7, 2101639 CrossRef CAS.
- D. Palanker, Femtosecond lasers for ophthalmic surgery enabled by chirped-pulse amplification, N. Engl. J. Med., 2018, 379, 2267–2269 CrossRef PubMed.
- Y. Zhu, C. Geng, L. Hu, L. Liu, Y. Zhu, Y. Yao, C. Li, Y. Ma, G. Li and Y. Chen, Skin-Like Near-Infrared II Photodetector with High Performance for Optical Communication, Imaging, and Proximity Sensing, Chem. Mater., 2023, 35, 2114–2124 CrossRef CAS.
- J. Gjerde and R. A. Jishi, Hyperbolic Behavior and Antiferromagnetic Order in Rare-Earth Tellurides, Crystals, 2022, 12, 1839 CrossRef CAS.
- K. Yumigeta, Y. Qin, H. Li, M. Blei, Y. Attarde, C. Kopas and S. Tongay, Advances in Rare-Earth Tritelluride Quantum Materials: Structure, Properties, and Synthesis, Adv. Sci., 2021, 8, 2004762 CrossRef CAS PubMed.
- A. M. Tokmachev, D. V. Averyanov, A. N. Taldenkov, O. E. Parfenov, I. A. Karateev, I. S. Sokolov and V. G. Storchak, Lanthanide f7 metalloxenes – a class of intrinsic 2D ferromagnets, Mater. Horiz., 2019, 6, 1488–1496 RSC.
- S. Lei, J. Lin, Y. Jia, M. Gray, A. Topp, G. Farahi, S. Klemenz, T. Gao, F. Rodolakis, J. L. McChesney, C. R. Ast, A. Yazdani, K. S. Burch, S. Wu, N. P. Ong and L. M. Schoop, High mobility in a van der Waals layered antiferromagnetic metal, Sci. Adv., 2020, 6, eaay6407 CrossRef CAS.
- Z. Gao, Y. Wang, J. Gao, Z. Cui, X. Zhang, J. Shi and X. Fan, Bipolar ferromagnetic semiconductor with large magnetic moment: EuGe2 monolayer, Comput. Mater. Sci., 2022, 213, 111611 CrossRef CAS.
- O. E. Parfenov, D. V. Averyanov, A. M. Tokmachev, I. S. Sokolov, I. A. Karateev, A. N. Taldenkov and V. G. Storchak, High-Mobility Carriers in Germanene Derivatives, Adv. Funct. Mater., 2020, 30, 1910643 CrossRef CAS.
- G. Yang, J.-S. Chai, K. Bu, L.-F. Xu and J.-T. Wang, Structural, magnetic, and electronic properties of EuSi2 thin films on the Si(111) surface, Phys. Chem. Chem. Phys., 2022, 24, 6782–6787 RSC.
- Y. Wang, Z. Cui, H. Zeng, Z. Wang, X. Zhang, J. Shi, T. Cao and X. Fan, Tunable magnetic order in two-dimensional layered GdGe2, J. Mater. Chem. C, 2022, 10, 1259–1269 RSC.
|
This journal is © The Royal Society of Chemistry 2024 |
Click here to see how this site uses Cookies. View our privacy policy here.