DOI:
10.1039/D4SC04243C
(Review Article)
Chem. Sci., 2024,
15, 16006-16014
Cyclic polymers from alkynes: a review
Received
26th June 2024
, Accepted 2nd September 2024
First published on 4th September 2024
Abstract
Cyclic polymers have applications across various fields, including material science, biomedicine, and inorganic chemistry. Cyclic polymers derived from alkyne monomers have expanded the application scope to include electronic materials and polyolefins. This review highlights recent advancements in the synthesis of cyclic polymers from both mono- and disubstituted alkynes. The aim is to provide a comprehensive overview of the synthetic methodologies and the application of cyclic polymers derived from alkynes. Additionally, this review will facilitate a comparative analysis of the advantages and limitations of various synthetic methods and describe opportunities for future development of novel catalytic systems to synthesize cyclic polymers from alkynes.
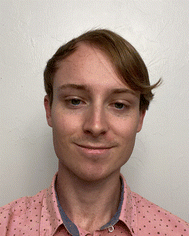 Parker T. Boeck | Parker T. Boeck received his B.A. in Chemistry from the University of North Carolina at Chapel Hill in 2020 while developing novel controlled polymerizations under the guidance of Prof. You. He is now a PhD student in the Veige group at the University of Florida. His doctoral research focuses on the synthesis of cyclic polyacetylenes. |
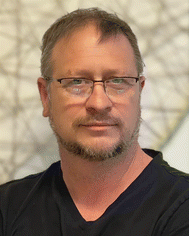 Adam S. Veige | Dr Adam Veige is the V. T. and Louise Jackson Professor of Chemistry at the University of Florida, where he also serves as the Director for the Center for Catalysis. He received his Hons. BSc degree in Chemistry in 1997 from the University of Western Ontario, Canada, and earned his PhD in 2003 at Cornell University. Since joining the University of Florida in 2004, Prof. Veige has led a multidisciplinary research group that explores a broad range of topics within transition metal catalysis and polymer chemistry. |
1. Introduction
The increasing need for flexible electronic components has prompted extensive exploration into the synthesis of conjugated polymers.1 The unique combination of flexibility, electronic conductivity, and tuneable electrochemical properties make conjugated polymers ideal for advanced electronic applications.2 Poly(3-hexylthiophene) is an example of a conjugated polymer used in organic sensors3 and organic solar cells.4 Substituted polyacetylenes are also conjugated and exhibit electronic conductivity,5–9 electrochromism,10–13 and luminescence.14–19 Leveraging these properties, researchers use polyacetylenes for sensing cyanide,17 photoresists,20 and light emitting diodes (LEDs).21 Some polyacetylenes adopt a helical secondary structure,22 enabling applications in enantiomer resolution,23 chirality sensing,24 and circularly polarized luminescence.16 Most recently, and the subject of this review, conjugated polyacetylenes with a cyclic topology are now available, thus opening new opportunities to explore topological effects on all the aforementioned properties and applications. For example, discovered in our lab, cyclic polyacetylene (cPA) synthesized at any temperature has a predominantly trans configuration, whereas the linear forms initially with cis bonds. Additionally, films of cPA are more stretchable leading to a significant increase in conductivity compared to linear polyacetylene (lPA).
Cyclic and linear polymers with the same repeat unit and molar mass exhibit different thermal,25 rheological,26 crystallization,27 and degradation28 properties. For example, cyclic polyesters degrade slower than linear versions of identical molar mass,28 a feature particularly advantageous for cyclic polyacetylenes given their tendency to degrade oxidatively.29 Nature also takes advantage of topology; cyclic DNA30 and peptides31,32 exhibit increased thermal, chemical, and enzymatic stability.
The solid-state physical properties of cyclic and linear polymers are different. For example, the reduced free volume33 created by a lack of chain ends in cyclic polymers result in higher glass transition temperatures (Tg) than linear analogues of similar molar mass.25,34,35 Also, a lack of chain ends for cyclic polymers results in fewer entanglements leading to lower melt viscosities.36 The higher Tg and lower melt viscosities of cyclic polymers suggest their promising application as thermoplastics with improved processing capability.
Amphiphilic block copolymers undergo self-assembly in both the solution and solid-state and given the decreased size of cyclic polymers their assemblies are smaller. Indeed, theoretical studies predict the spacing between cyclic block copolymers assemblies is 0.63 smaller.37 Subsequent early experimental studies validated the theory revealing solid-state domain spacing 0.70–0.75 times smaller for assembled cyclic block copolymers compared to linear analogues,38,39 a result crucial to advancing block copolymer nanolithography. Recent studies suggest a cyclic topology has more impact on the self-assembly properties of polymers than previously thought.40
Despite the many studies illustrating the physical differences between linear and cyclic polymers many properties remain underexplored, for example conductivity in conjugated polymers41–43 with a cyclic topology. The synthesis of cyclic polymers in reasonable quantities and purity has always been the limiting factor to exploring the full suite of cyclic polymer properties. Ring closing of a linear polymeric precursor and ring expansion are the two most common methods for synthesizing cyclic polymers. Historically, catalysts used to polymerize alkynes do not provide control over the polymer end groups, thus preventing the synthesis of cyclic polyacetylenes via ring closure. Overcoming this synthetic limitation, catalysts can now directly synthesize conjugated cyclic polyacetylenes from alkynes according to Fig. 1. In this review, we highlight recent key developments in the synthesis of cyclic polyacetylenes from alkynes, their emerging applications, and the remaining challenges starting from the synthesis a pincer supported tungsten alkylidyne complex.
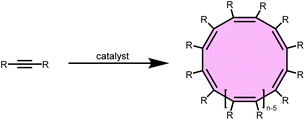 |
| Fig. 1 Synthesis of cyclic polymers from alkynes. | |
2. Cyclic polymers from monosubstituted alkynes
A breakthrough in cyclic polymer synthesis came in 2012 when we reported alkylidyne complex 1 initiates the polymerization of phenylacetylene.44 At the time, we were not aware of the cyclic topology of the resulting polyacetylenes. However, the clues to the hidden topology were there originally, including the isolation of tethered alkylidene intermediates 2 and 3 (ref. 44) and ring expanded metallacyclopentadienes 4 and 5 (Fig. 2).45 Eventually, in 2016 we reported the tethered alkylidene tungsten catalyst 6 initiates the polymerization of phenyl acetylene (1
:
10
000) to give cyclic poly(phenylacetylene) (cPPA)46 in high yield (>94%) (Table 1, entry 1). Cyclic polyacetylenes from most alkyne monomers are now accessible using 6 including, aliphatic alkynes, gaseous alkynes (propyne and acetylene), and both electron poor and rich phenylacetylene derivatives.34,35,47–49 The assignment of a cyclic topology for the polymers produced from 6 and alkynes comes from a variety of techniques; such as, atomic force microscopy (AFM),47,50 differential scanning calorimetry (DSC),25 gel permeation chromatography (GPC),48,51 and rheology.34,35 One of the exciting features of initiator 6 is its extreme reactivity toward alkynes, with measured activities of 180
000
000 g molcat−1 h−1.34 The cyclic polyacetylenes produced by 6 typically exhibit a predominantly cis (>90%) configuration with the notable exception of cyclic polyacetylene.
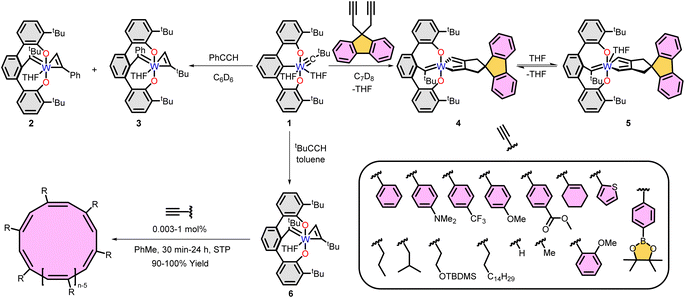 |
| Fig. 2 Synthesis of ring expanded metallacyclopentadienes (top left), tethered alkylidene intermediates (top right), polymerization of alkynes and monomer scope (bottom). | |
Table 1 Polymerization of phenylacetylene
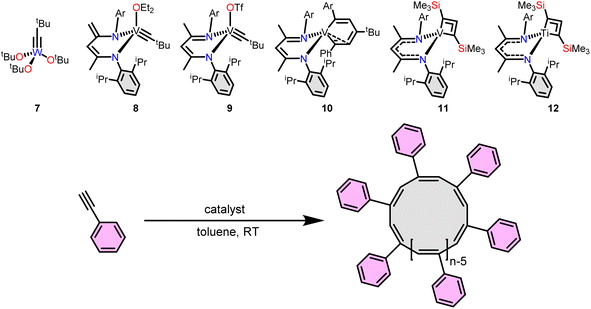
|
Entry |
Monomer |
Catalyst |
[PhCCH]: [Cat] |
Time |
Yield (%) |
M
n
(kDa) |
Đ
|
1 |
PA |
6
|
10 000 |
15 min |
94 |
30.8 |
2.2 |
2 |
PA |
6
|
5000 |
15 min |
96 |
69 |
1.8 |
3 |
PA |
8
|
1000 |
12 h |
94 |
62.9 |
1.6 |
4 |
PA |
8
|
100 |
2 h |
94 |
272 |
1.3 |
5 |
PA |
10
|
1000 |
12 h |
37 |
108 |
1.5 |
6 |
PA |
10
|
1000 |
12 h |
19 |
115 |
1.5 |
7 |
PA |
11
|
500 |
12 h |
59 |
48 |
4.1 |
8 |
PA |
11
|
100 |
12 h |
21 |
30 |
3.4 |
9 |
PA |
12
|
50 |
12 h |
16 |
32 |
3.6 |
10 |
PA |
12
|
250 |
12 h |
7 |
38 |
3.5 |
The mechanism of cyclic polymer formation remains unclear. Isolation or in situ detection of several metallacyclopentadienes45,52,53 lead to our initial proposal of a straightforward ring expansion polymerization (REP) where subsequent insertions of alkyne grow the cyclic polymer on the metal center. The role, if any, of the tethered alkylidene remains elusive.
Alkylidynes polymerize acetylenes to give linear polyacetylenes.54 However, recent results by Mindiola and a patent suggest the possibility that cyclic polymers are also accessible from alkynes initiated by alkylidynes. In the patent, Exxon reports that (tBuO)3W
CtBu initiates the polymerization of phenylacetylene to give cyclic poly(phenylacetylene).55 Evidence for the cyclic topology comes from DSC, GPC, and NMR data. Mindiola et al., in 2024, reported the vanadium-alkylidyne 8 initiates the polymerization of phenylacetylene to yield cyclic poly(phenylacetylene) (with some linear impurities).56 In earlier work,57 they noted in passing that polymer forms when 9 was exposed to phenylacetylene but its topology was not interrogated. Revisiting those initial results revealed the cyclic topology of PPA formed by 8. Very good yields of polymer form within 12 h with a 1
:
1000 catalyst: monomer loading of 8 (Table 1, entry 3). Increasing the loading to 1
:
100 results in a corresponding faster reaction providing 94% in 2 h (Table 1, entry 4). Initial inquiries into the mechanism of polymerization revealed several key intermediates. The interplay between the non-innocent dBDI2− (ArNC(CH3)CHC(CH2)Nar, Ar = 2,6-iPr2C6H3) ligand is a key feature to access a cyclic topology. For example, intermediate 10, bearing the protonated form of the dBDI2− ligand and a metallacyclohextriene was isolated and is active for the synthesis of cPPA. Although, the yield was low (19–37%) the cPPA was topologically pure (Table 1, entries 5 & 6).
Installing metal–carbon triple bonds within alkylidynes can be synthetically challenging. Circumventing the need to access an alkylidyne, Mindiola recently reported that treatment of Ti or V dihalide precursors with 1,3-dilithioallene yields metallacyclobuta-(2,3)-diene species capable of polymerizing phenylacetylene to yield cPPA.58 While the complexes are simple to prepare the yields of cyclic polyphenylacetylene are modest when using complexes 11 (21–59%) or 12 (7–16%), with relatively higher dispersity (3.5–4.1) compared to 8 and 10 (1.3–1.6) (Table 1, entries 7–10). The cis/trans content of cyclic poly(phenylacetylene) produced from catalysts 7–12 has not been evaluated.
3. Cyclic polymers from disubstituted alkynes
3.1 Polymerization of disubstituted alkynes
The polymerization of disubstituted alkynes was thoroughly studied.59–63 However, the polymerization of disubstituted alkynes for cyclic polymer synthesis is receiving renewed attention. In 2021, Maeda et al. reported the polymerization of diphenylacetylenes at 80 °C with TaCl5 pretreated with nBu4Sn in toluene to yield cyclic polymers (Fig. 3) with high cis-content.64 The exciting discovery is the easy access to cyclic polyacetylenes from commercial reagents.
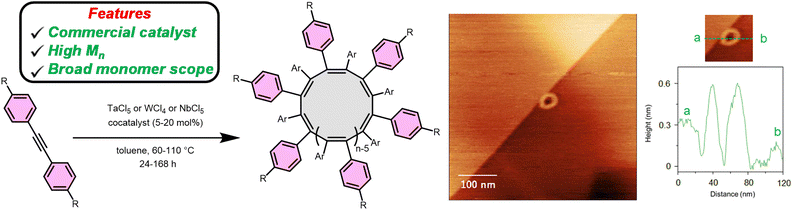 |
| Fig. 3 Polymerization of disubstituted acetylenes using commercially available catalysts and AFM image of cyclic polydiphenylacetylene on HOPG. Reprinted with permission from Macromolecules. 2023, 56, 5873–5880. Copyright 2023 American Chemical Society. | |
In subsequent work Maeda and Taniguchi et al. reveal NbCl5 pretreated with PhSiH3 also affords cyclic polydiphenylacetylenes from diphenylacetylenes substituted in either the meta or para positions in high yield and high molar mass.65 Additionally, the NbCl5/PhSiH3 catalysts system produces predominately cis-double bonds within the backbone. Moreover, using NbCl5/PhSiH3 the authors synthesized the first helical cyclic polyacetylenes by polymerizing monomers containing chiral side groups. These polymers form predominantly one-handed helices capable of circularly polarized luminescence. The high molar mass of the polymers appeared to drastically increase the induced circular dichroism intensities compared to previous reports.65
Expanding the monomer scope Maeda et al. reported a WCl4 initiated polymerization of electron poor, rich, and neutral diphenylacetylenes.66 WCl4 pretreated with Ph4Sn and PPh3 at 110 °C will polymerize diphenylacetylenes to produce cyclic polydiphenylacetylenes. AFM provided conclusive evidence for the cyclic topology of the polymers (Fig. 3).
3.2 Polymerization of cyclic alkynes
In 2016 Fisher et al. reported the ring closing of a linear polymer to give cyclic polyphenylene ethynylene during ring opening alkyne metathesis polymerization (ROAMP) as depicted in Fig. 4.67 In that system the choice of catalyst dictates whether a linear or cyclic polymer forms. Specifically, the authors found that polymerizing 5,6,11,12-tetradehydrobenzo[a,e][8]annulene with alkylidyne 13 selective (for the chain end) backbiting led to the formation of cyclic phenylene ethynylenes (with minor linear impurities) as confirmed by NMR and mass spectrometry (Fig. 4).
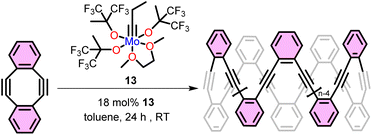 |
| Fig. 4 Synthesis of cyclic poly(o-phenylene ethylene) using ROAMP catalyst 13. | |
A common method to avoid linear impurities formed during ring closing of a linear polymer is to perform ring expansion of an already cyclic initiator. To this end just a few years later the first ring expansion alkyne metathesis polymerization (REAMP) of cyclic alkynes to yield cyclic polyalkynes was reported by Veige et al. Treating 3,8-didodecyloxy-5,6-dihydro-11,12-dihydrodibenzo[a,e]-[8]annulene (14), with the tethered alkylidyne initiator 15 in toluene produces cyclic poly(o-phenylene ethynylene) (cPoPE). Notably, the polymerization proceeds quickly (5 min) producing high yields (70%) of cPoPE with high molar mass (Mn > 100 kg mol−1) but high dispersity (Đ = 4.2–9) (Fig. 5).
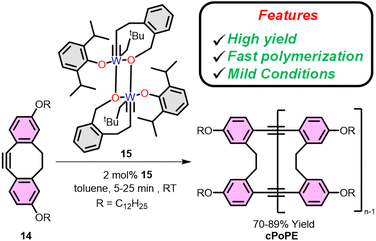 |
| Fig. 5 Polymerization of 14via REAMP using tethered alkylidyne 15. | |
Using the methods described in this review, cyclic polymers are now accessible from almost any alkyne. Overall, the recent advances across all cyclic polymers derived from alkynes through catalyst design, scalability, and monomer scope are opening new applications for these interesting materials that exploit their lack of chain ends.
4. Applications of cyclic polyacetylenes
4.1 Precursor to cyclic polyolefins
The scalable synthesis of cyclic analogues of commercially relevant linear polymers is a longstanding challenge. Given the high activity of initiator 6 commercially relevant cyclic polymers are now accessible at scale. In pursuit of this goal Veige and Sumerlin et al. reported the synthesis of cyclic poly(propylene). Complex 6 initiates the polymerization of propyne gas to form the unsaturated cyclic poly(propyne) and subsequent hydrogenation yields atactic cyclic poly(propylene) (cPP) (Fig. 6). cPP prepared with 6 has a higher glass transition temperature than previously reported linear atactic polypropylene. Investigation of the bulk rheological properties of cPP reveals a lack of a plateau modulus and a zero-shear viscosity ten times lower than linear poly(propylene) (lPP). Both properties suggest interesting potential of cyclic polyolefins as additives in commercial applications.
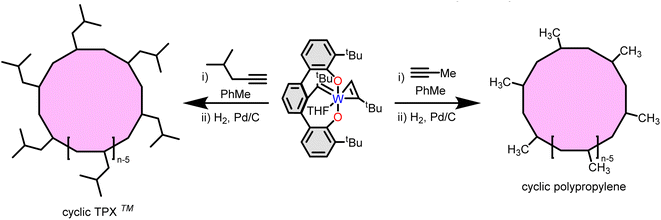 |
| Fig. 6 Synthesis of cyclic poly(4-methyl-pentene) (also known as TPX™) and cyclic polypropylene using tethered tungsten alkylidene 6. | |
Poly(4-methyl-1-pentene) (or TPX™) is a transparent thermoplastic used primarily in packaging and membranes. Treating 4-methyl-1-pentyne (4-MP) with 6 yields unsaturated cyclic poly(4-methyl-1-pentyne) and hydrogenation over Pd/C in cyclohexane yields atactic cyclic poly(4-methyl-1-pentyne) (cPMP).34 Again, cPMP exhibits a higher Tg (39 °C vs. 29 °C) with no plateau modulus and a zero-shear viscosity fifteen times less than a linear poly(4-methyl-1-pentyne) (lPMP) sample. Importantly, catalyst 6 initiates pentyne polymerization with an activity of 180
000
000 gpolymer molcat−1 h−1. Given its high activity initiator 6 has the potential to produce cyclic polyolefins at an industrially relevant scale.
4.2 Synthesis of cyclic brushes
Another application of cyclic polyacetylenes is as a precursor to ultra-high molar mass cyclic bottlebrush polymers via post-polymerization modification.50 Adding bromine to cyclic polyphenylacetylene brominates the backbone yielding a benzylic bromine capable of initiating atom transfer radical polymerization (ATRP) to grow cyclic brush polymers (Fig. 7). Butyl acrylate and styrene side chains grow from the macrocyclic initiator with 52–72% grafting efficiencies. Atomic force microscopy (AFM) interrogation of the bottlebrush polymers dispersed on a surface reveal the topology of the polymers produced from alkynes with 6.
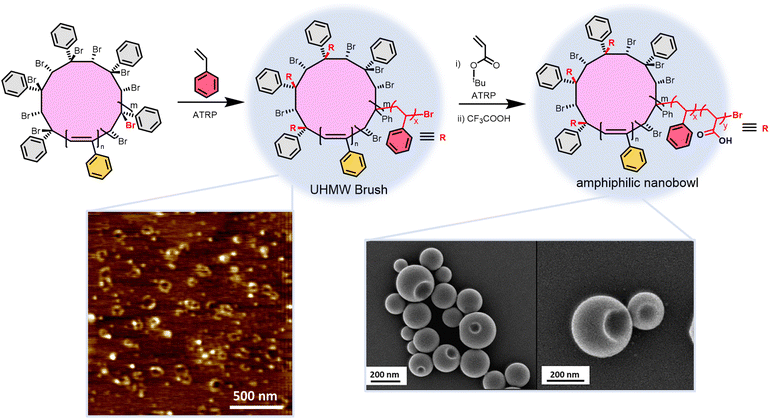 |
| Fig. 7 Synthesis of ultra-high molecular weight bottle brushes via ATRP. AFM (bottom left) and SEM images (bottom right) of ultra-high molecular weight bottle brushes. Reprinted with permission from Macromolecules, 2020, 53, 9717–9724 (bottom left) and Macromolecules, 2022, 55, 7446–7453 (bottom right). Copyright 2020 and 2022 American Chemical Society. | |
Leveraging the broad monomer scope of grafting from via ATRP enables the synthesis of amphiphilic cyclic bottlebrushes from alkynes capable of self-assembling into porous spheres and nanobowls.68 Grafting from using styrene followed by chain extension using tert-butylacrylate provides the bottlebrush arms. Treating the cyclic bottlebrushes with trifluoroacetic acid in THF converts the tert-butylacrylate repeat units into acrylic acid. Dissolving the amphiphilic bottlebrush in THF forms spherical nano-assemblies with multiple pores. Dropwise addition of water to this solution converts the porous spheres to spheres containing only a single hole (nanobowls). Identical linear bottlebrushes only form the porous spherical nano-assemblies, indicating the topology can affect the self-assembly behaviour of amphiphilic copolymers. The unsaturated bonds of polyacetylenes are excellent sites to add functionality in post-polymerization modification; however, they also act as pathways for electron mobility making cyclic polyacetylenes semi-conducting polymers.
4.3 Synthesis of conductive cyclic polymers
Doping and irradiating polyacetylenes alters their conductivity.5,7,42,69,70 The effect of a cyclic topology on conductivity is challenging to interrogate though some new insights are emerging.71 Exposing a dilute solution of 6 to acetylene gas rapidly produces freestanding films of cyclic poly(acetylene) (cPA) (Fig. 8).47 An important feature is cPA synthesized with 6 contains 99% trans double bonds regardless of the polymerization temperature, whereas linear poly(acetylene) (lPA) usually requires heating to convert cis to trans. The methodology allows for rapid synthesis under dilute conditions that temporarily generates soluble or homogenized cPA. Notoriously difficult to functionalize, cyclic brush polymers were generated from the temporarily soluble polymers using a similar bromination ATRP strategy as previously outlined.47,50 AFM imaging of cPA brush polymers add to the support of a cyclic topology.
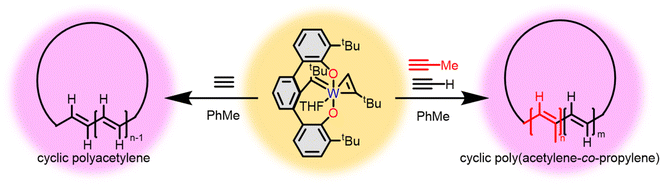 |
| Fig. 8 Synthesis of cyclic polyacetylene (left) and cyclic poly(acetylene-co-propylene) using tungsten tethered alkylidene 6. | |
Consistent with prior literature cyclic copolymers of acetylene and propyne exhibit significantly lower conductivities relative to cPA.72 Distortion of backbone planarity caused by the methyl substituent reduces the conductivity. However, simply stretching cPA either by hand or using dynamic mechanical analysis (DMA) drastically increases its conductivity.73 By stretching I2 doped cPA to 1.6 times its original length (Fig. 9) the conductivity of the sample increases by 5× while stretching lPA failed to produce similar results (Table 2). More stretchable films of cPA, due either to the cyclic topology or low crosslink density, lead to improved alignment of the polyacetylene fibrils and thus improved conductivity.
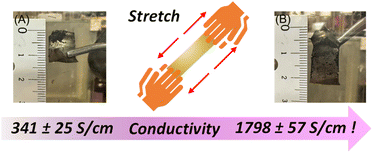 |
| Fig. 9 Photographs of cPA films (A) before and (B) after manual stretching. | |
Table 2 Conductivities of I2 doped cPA and lPA films before and after stretching
Sample |
Composition |
Conductivity (S cm−1) |
Non-stretched cPA |
(CHI0.34)n |
341 ± 25 |
Hand-stretched cPA |
(CHI0.31)n |
1798 ± 57 |
Non-stretched lPA |
(CHI0.27)n |
524 ± 48 |
Hand-stretched lPA |
(CHI0.28)n |
485 ± 65 |
4.4 Synthesis of functional cyclic polymers
Catalyst 6 also polymerizes a variety of functional alkynes.49 For example, 6 initiates the polymerization 4-ethynylanisole to produce cyclic poly(4-ethynylanisole).48 Cleaving the methyl group using BBr3 generates cyclic poly(4-ethynylphenol) (cPEP-OH). cPEP-OH is pH responsive; it was soluble at pH > 11 and insoluble at pH ≤ 11.
Similarly, 6 initiates the polymerization of boron functionalized monomers to yield cyclic poly(4-ethynylphenylboronate esters) (cPEPB).25 Using Suzuki coupling methods cPEPB is easily functionalized (92% by NMR). Treating boronate ester containing cyclic polymers with an excess of methylboronic acid in the presence of trifluoroacetic acid yields cyclic poly(4-ethynylphenylboronic acid) (cPEPB-OH) as depicted in Fig. 10. Dissolving cPEPB-OH in THF and treating with a diglycerol crosslinker produces gels. Gels produced from cPEPB-OH and linear lPEPB-OH have similar network stability, but the gel derived from the cyclic version has a higher storage modulus (G′).
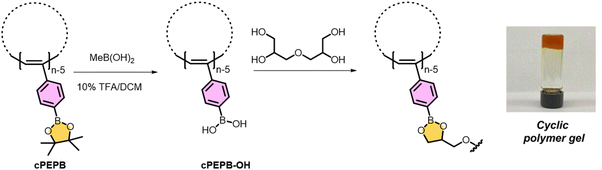 |
| Fig. 10 Synthesis of a cyclic polymer gel by post polymerization modification of cPEPB followed by crosslinking with diglycerol. Reprinted with permission from Macromolecules, 2024, 57, 1779–1787. Copyright 2024 American Chemical Society. | |
5. Guidance for handling of cyclic polyacetylenes and cyclic polymer catalysts
Given the sensitivity of both the described catalysts and produced polymers it is important for those who wish to perform the synthesis of cyclic polyacetylenes to know how to properly handle both. Transition metal catalysts 1 and 6 are well known to be highly oxophilic49,74 making them highly sensitive to oxygen exposure. Thus, all steps of their synthesis and any polymerizations conducted using them must be performed in oxygen- and moisture-free environments. Despite its extreme reactivity it is also worth mentioning that catalyst 6 is highly stable in solution (months) and in the solid state (years) when properly stored (low temperature free of oxygen).
Less obviously, cyclic polyacetylenes degrade rapidly in oxygenated solutions49,75 and slowly in the solid state. Thus, precipitation should be performed without exposure to oxygen (into deoxygenated solution). Additionally, GPC and NMR analysis should be performed in the absence of oxygen which again is easily achieved by preparing the samples in an inert atmosphere glovebox in appropriately airtight sample holders. The reader should also note that cyclic polyacetylenes are prone to electrocyclization upon extended dissolution in air free solvent at low temperatures.29 Or in the solid state slowly at room temperature and quickly when exposed to elevated temperatures.76
6. Conclusions
Thousands of reports over the past 60 years extoll the fascinating properties, chemistry, characterization, post-polymerization functionalization, imaging, stereochemistry, and application of linear polyacetylenes.77–80 New access to cyclic versions of linear polyacetylenes creates exciting opportunities to now investigate the role of topology. The ring expansion routes extolled herein are particularly exciting because they provide access to cyclic polymers free of functional groups in the polymer backbone used to produce comparable polymers via a ring-closing. Additional advantages of these methods over traditional ring closing methods include the possibility of eliminating the presence of linear polymer impurities typical of ring closing methods and eliminating the requirement of conducting the polymerization in highly dilute conditions. Combined, these advantages and the discovery of highly active catalysts 1 and 6 enable the large-scale synthesis of cyclic polymers. Access to large quantities of cyclic polyacetylenes will accelerate the discovery of new applications and will enable the properties of polyacetylenes to be re-examined but now with a cyclic topology.
In particular, access to cyclic polyolefins, such as cyclic polypropylene, provide an opportunity to exploit the inherent properties of cyclic polymers on commercially relevant materials. Catalyst 6 is central to new cyclic polymer discoveries since it possesses high polymerization activity, broad monomer scope, and operates under mild conditions if water and oxygen are absent. New catalyst systems continue to evolve. The simplicity of Maeda's in situ generated initiators provide easy access to disubstituted cyclic polyacetylenes. Broadening access further, Mindiola et al. demonstrates that alkylidynes in general are likely to play a vital role in the further development of cyclic polymer catalyst design.
Polymerization of monosubstituted alkynes with low catalyst loadings (0.003 mol%) using 6 is now possible. However, the polymerization of disubstituted alkynes requires 5 mol% of catalyst and 10–20 mol% additive. More active systems for the polymerization of disubstituted alkynes are desirable. Additionally, the polymerization mechanisms for the catalyst systems reported thus far need more investigation to achieve ultimate control over cyclic polymer molecular weight, stereochemistry, dispersity, and monomer scope. Developing “living” catalysts with high initiation efficiency will allow control over both the molecular weight and dispersity of the produced polymers. Living catalysts for cyclic polymer synthesis will exponentially expand the field by enabling the synthesis of cyclic block copolymers.
Improvements in the polymerization of cyclic polymers from alkynes represent a major step towards realizing commercial applications. The scalable (decagram) synthesis of cyclic polyolefins from cyclic polyacetylenes via hydrogenation will enable experiments that probe the role of topology on commercially relevant polymers. In the near term the next steps will be to exploit the new access to large quantities of cyclic polymers from diverse alkyne monomers to further elucidate the role of a cyclic topology on the physical properties of polymers, composites, blends, thin films, nanostructured materials, crosslinked networks, vitrimers and many other advanced materials.
Data availability
The data that support the findings of this review can be found in the cited references.
Author contributions
PTB composed the manuscript, curated the figures, tabulated the data, and created the artwork. ASV edited the manuscript.
Conflicts of interest
The authors declare the following competing financial interest(s): University of Florida, P. T. B. and A. S. V. have filed patents related to this work. A. S. V. is an equity stake holder in Oboro Labs Inc.
Acknowledgements
The authors thank the National Science foundation for past and continuing support for the development of cyclic polymer catalysts (CHE-2154377) and polymers (CHE-2108266).
Notes and references
- C. Kan and Y. Lam, Appl. Sci., 2021, 11, 3914 CrossRef CAS.
- Z. Zhang, M. Liao, H. Lou, Y. Hu, X. Sun and H. Peng, Adv. Mater., 2018, 30, 1–19 Search PubMed.
- S. Y. Son, G. Lee, H. Wang, S. Samson, Q. Wei, Y. Zhu and W. You, Nat. Commun., 2022, 13, 1–11 Search PubMed.
- J. Noh, G. U. Kim, S. Han, S. J. Oh, Y. Jeon, D. Jeong, S. W. Kim, T. S. Kim, B. J. Kim and J. Y. Lee, ACS Energy Lett., 2021, 6, 2512–2518 CrossRef CAS.
- N. Basescu, Z. X. Liu, D. Moses, A. J. Heeger, H. Naarmann and N. Theophilou, Nature, 1987, 327, 403–405 CrossRef CAS.
- J. Tsukamoto, A. Takahashi and K. Kawasaki, Jpn. J. Appl. Phys., 1990, 29, 125–130 CrossRef CAS.
- M. Audenaert, G. Gusman and R. Deltour, Phys. Rev. B: Condens. Matter Mater. Phys., 1977, 39, 7380–7382 Search PubMed.
- Y. W. Park, C. Parker, Y. S. Lee and C. O. Yoon, Solid State Commun., 1988, 65, 147–150 CrossRef CAS.
- D. Ofer, L. Y. Park, R. R. Schrock and M. S. Wrighton, Chem. Mater., 1991, 3, 573–575 CrossRef CAS.
- A. C. Pauly, D. Varnado Jr, C. W. Bielawski and P. Theato, Macromol. Rapid Commun., 2014, 35, 210–213 CrossRef CAS PubMed.
- B. T. Luppi, A. V. Muralidharan, N. Ostermann, I. T. Cheong, M. J. Ferguson, I. Siewert and E. Rivard, Angew. Chem., Int. Ed., 2022, 61, e202114586 CrossRef CAS PubMed.
- T. Sugimoto, T. Koremoto, T. Inoue, R. Nomura and T. Masuda, Polym. Bull., 1999, 42, 55–60 CrossRef CAS.
- J. Qu, Y. Suzuki, M. Shiotsuki, F. Sanda and T. Masuda, Polymer, 2007, 48, 4628–4636 CrossRef CAS.
- Y. Dong, J. W. Y. Lam, H. Peng, K. K. L. Cheuk, H. S. Kwok and B. Z. Tang, Macromolecules, 2004, 37, 6408–6417 CrossRef CAS.
- E. A. Imhoff and D. B. Fitchen, Solid State Commun., 1982, 44, 1–4 CrossRef.
- B. Zhao, K. Pan and J. Deng, Macromolecules, 2018, 51, 7104–7111 CrossRef CAS.
- X. Lou, Q. Zeng, Y. Zhang, Z. Wan, J. Qin and Z. Li, J. Mater. Chem., 2012, 22, 5581–5586 RSC.
- L. Lauchlan, S. Etemad, T. C. Chung, A. J. Heeger and A. G. MacDiarmid, Phys. Rev. B: Condens. Matter Mater. Phys., 1981, 24, 3701–3711 CrossRef CAS.
- J.
rti and H. Kuzmany, Phys. Rev. B: Condens. Matter Mater. Phys., 1988, 38, 5634–5639 CrossRef PubMed.
- J. L. Hua, J. W. Y. Lam, H. Dong, L. Wu, K. S. Wong and B. Z. Tang, Polymer, 2006, 47, 18–22 CrossRef CAS.
- S. V. Frolov, A. Fujii, D. Chinn, M. Hirohata, R. Hidayat, M. Taraguchi, T. Masuda, K. Yoshino and Z. Valy Vardeny, Adv. Mater., 1998, 10, 869–872 CrossRef CAS.
- E. Yashima, K. Maeda, H. Lida, Y. Furusho and K. Nagai, Chem. Rev., 2009, 109, 6102–6211 CrossRef CAS PubMed.
- C. Zhang, L. Liu and Y. Okamoto, Trends Anal. Chem., 2020, 123, 115762 CrossRef CAS.
- E. Anger, H. Iida, T. Yamaguchi, K. Hayashi, D. Kumano, J. Crassous, N. Vanthuyne, C. Roussel and E. Yashima, Polym. Chem., 2014, 5, 4909–4914 RSC.
- D. Konar, V. K. Jakhar, K. A. Stewart, D. W. Lester, A. S. Veige and B. S. Sumerlin, Macromolecules, 2024, 57, 1779–1787 CrossRef CAS.
- D. Vlassopoulos, R. Pasquino and F. Snijkers, Progress in the rheology of cyclic polymers, Topol. Polym. Chem., 2013, 291–316 CAS.
- H. R. Kricheldorf and S. M. Weidner, Polymer, 2023, 276, 125946 CrossRef CAS.
- J. N. Hoskins and S. M. Grayson, Macromolecules, 2009, 42, 6406–6413 CrossRef CAS.
- V. Percec and J. G. Rudick, Macromolecules, 2005, 38, 7241–7250 CrossRef CAS.
- A. H. El-Sagheer and T. Brown, Int. J. Pept. Res. Ther., 2008, 14, 367–372 CrossRef CAS.
- O. Saether, D. J. Craik, I. D. Campbell, K. Sletten, J. Juul and D. G. Norman, Biochemistry, 1995, 34, 4147–4158 CrossRef CAS PubMed.
- D. Laimou, T. Katsila, J. Matsoukas, A. Schally, K. Gkountelias, G. Liapakis, C. Tamvakopoulos and T. Tselios, Eur. J. Med. Chem., 2012, 58, 237–247 CrossRef CAS PubMed.
- R. P. White and J. E. G. Lipson, Macromolecules, 2016, 49, 3987–4007 CrossRef CAS.
- Z. Miao, D. Pal, W. Niu, T. Kubo, B. S. Sumerlin and A. S. Veige, Macromolecules, 2020, 53, 7774–7782 CrossRef CAS.
- W. Niu, S. A. Gonsales, T. Kubo, K. C. Bentz, D. Pal, D. A. Savin, B. S. Sumerlin and A. S. Veige, Chem, 2019, 5, 237–244 CAS.
- G. B. McKenna, G. Hadziioannou, P. Lutz, G. Hild, C. Strazielle, C. Straupe, P. Rempp and A. J. Kovacs, Macromolecules, 1987, 20, 498–512 CrossRef CAS.
- J. F. Mark, Macromolecules, 1993, 26, 1442–1444 CrossRef.
- S. Lecommandoux, R. Borsali, M. Schappacher, A. Deffieux, T. Narayanan and C. Rochas, Macromolecules, 2004, 37, 1843–1848 CrossRef CAS.
- J. E. Poelma, K. Ono, D. Miyajima, T. Aida, K. Satoh and C. J. Hawker, ACS Nano, 2012, 6, 10845–10854 CrossRef CAS PubMed.
- C. Chen, M. K. Singh, K. Wunderlich, S. Harvey, C. J. Whitfield, Z. Zhou, M. Wagner, K. Landfester, I. Lieberwirth, G. Fytas, K. Kremer, D. Mukherji, D. Y. W. Ng and T. Weil, Nat. Commun., 2021, 12, 1–7 CrossRef PubMed.
- C. K. Chiang, A. J. Heeger and A. G. MacDiarmid, Ber. Bunsenges. Phys. Chem., 1979, 83, 407–417 CrossRef CAS.
- Y. W. Park, A. J. Heeger, M. A. Druy and A. G. MacDiarmid, J. Chem. Phys., 1980, 73, 946–957 CrossRef CAS.
- B. Z. Tang, H. Z. Chen, R. S. Xu, J. W. Y. Lam, K. K. L. Cheuk, H. N. C. Wong and M. Wang, Chem. Mater., 2000, 12, 213–221 CrossRef CAS.
- S. Sarkar, K. P. McGowan, S. Kuppuswamy, I. Ghiviriga, K. A. Abboud and A. S. Veige, J. Am. Chem. Soc., 2012, 134, 4509–4512 CrossRef CAS PubMed.
- C. D. Roland, T. Zhang, S. Venkatramani, I. Ghiviriga and A. S. Veige, Chem. Commun., 2019, 55, 13697–13700 RSC.
- C. D. Roland, H. Li, K. A. Abboud, K. B. Wagener and A. S. Veige, Nat. Chem., 2016, 8, 791–796 CrossRef CAS PubMed.
- Z. Miao, S. A. Gonsales, C. Ehm, F. Mentink-Vigier, C. R. Bowers, B. S. Sumerlin and A. S. Veige, Nat. Chem., 2021, 13, 792–799 CrossRef CAS PubMed.
- Z. Miao, T. Kubo, D. Pal, B. S. Sumerlin and A. S. Veige, Macromolecules, 2019, 52, 6260–6265 CrossRef CAS.
- P. T. Boeck, R. Yadav, B. S. Sumerlin and A. S. Veige, Macromolecules, 2023, 57, 71–77 CrossRef.
- D. Pal, Z. Miao, J. B. Garrison, A. S. Veige and B. S. Sumerlin, Macromolecules, 2020, 53, 9717–9724 CrossRef CAS.
- Z. Miao, D. Pal, W. Niu, T. Kubo, B. S. Sumerlin and A. S. Veige, Macromolecules, 2020, 53, 7774–7782 CrossRef CAS.
- K. P. McGowan, M. E. O'Reilly, I. Ghiviriga, K. A. Abboud and A. S. Veige, Chem. Sci., 2013, 4, 1145–1155 RSC.
-
A. M. Esper, An Interesting Pincer Ligand Capable of Reversible CC Bond Cleavage and its Role in Tungsten Catalysis, University of Florida, 2023 Search PubMed.
- A. Bray, A. Mortreux, M. Petit and T. Szymanska-buzar, J. Chem. Soc., Chem. Commun., 1993, 197–199 RSC.
-
US Pat., US011708430B2, 2023, pp. 1–18 Search PubMed.
- M. G. Jafari, J. B. Russell, H. Lee, B. Pudasaini, D. Pal, Z. Miao, M. R. Gau, P. J. Carroll, B. S. Sumerlin, A. S. Veige, M. Baik and D. J. Mindiola, J. Am. Chem. Soc., 2024, 146, 2997–3009 CrossRef CAS PubMed.
- F. Basuli, B. C. Bailey, D. Brown, J. Tomaszewski, J. C. Huffman, M. H. Baik and D. J. Mindiola, J. Am. Chem. Soc., 2004, 126, 10506–10507 CrossRef CAS PubMed.
- J. B. Russell, D. Konar, T. Keller, M. R. Gau, P. J. Carroll, J. Telser, D. W. Lester, A. S. Veige, B. S. Sumerlin and D. J. Mindiola, Angew. Chem., Int. Ed., 2024, 63, e202318956 CrossRef CAS PubMed.
- T. Masuda, M. Yamagata and T. Higashimura, Macromolecules, 1984, 17, 126–129 CrossRef CAS.
- T. Masuda, T. Takahashi and T. Higashimura, Macromolecules, 1985, 18, 311–317 CrossRef CAS.
- T. Masuda, A. Niki, E. Isobe and T. Higashimura, Macromolecules, 1985, 18, 2109–2113 CrossRef CAS.
- K. Tsuchihara, T. Masuda and T. Higashimura, Macromolecules, 1992, 25, 5816–5820 CrossRef CAS.
- T. Masuda, E. Isobe and T. Higashimura, J. Am. Chem. Soc., 1983, 105, 7473–7474 CrossRef CAS.
- S. Sueyoshi, T. Taniguchi, S. Tanaka, H. Asakawa, T. Nishimura and K. Maeda, J. Am. Chem. Soc., 2021, 143, 16136–16146 CrossRef CAS PubMed.
- D. Nakaguchi, T. Taniguchi, T. Nishimura and K. Maeda, Macromolecules, 2023, 56, 5873–5880 CrossRef CAS.
- M. Miyairi, T. Taniguchi, T. Nishimura and K. Maeda, Angew. Chem., Int. Ed., 2023, 62, 1–13 Search PubMed.
- S. Von Kugelgen, D. E. Bellone, R. R. Cloke, W. S. Perkins and F. R. Fischer, J. Am. Chem. Soc., 2016, 138, 6234–6239 CrossRef CAS PubMed.
- D. Pal, J. B. Garrison, Z. Miao, L. E. Diodati, A. S. Veige and B. S. Sumerlin, Macromolecules, 2022, 55, 7446–7453 CrossRef CAS.
- E. T. Kang, P. Ehrlich, A. P. Bhatt and W. A. Anderson, Macromolecules, 1984, 17, 1020–1024 CrossRef CAS.
- E. T. Kang, K. G. Neoh, T. Masuda, T. Higashimura and M. Yamamoto, Polymer, 1989, 30, 1328–1331 CrossRef CAS.
- B. J. Lidster, S. Hirata, S. Matsuda, T. Yamamoto, V. Komanduri, D. R. Kumar, Y. Tezuka, M. Vacha and M. L. Turner, Chem. Sci., 2018, 9, 2934–2941 RSC.
- Z. Miao, A. M. Esper, S. S. Nadif, S. A. Gonsales, B. S. Sumerlin and A. S. Veige, React. Funct. Polym., 2021, 169, 105088 CrossRef CAS.
- Y. H. Shen, R. Yadav, A. J. Wong, A. H. Balzer, T. H. Epps, B. S. Sumerlin and A. S. Veige, React. Funct. Polym., 2024, 195, 105810 CrossRef CAS.
- S. A. Gonsales, I. Ghiviriga, K. A. Abboud and A. S. Veige, Dalt. Trans., 2016, 45, 15783–15785 RSC.
- S. M. A. Karim, R. Nomura and T. Masuda, J. Polym. Sci. Part A Polym. Chem., 2001, 39, 3130–3136 CrossRef CAS.
- V. Percec, J. G. Rudick, P. Nombel and W. Buchowicz, J. Polym. Sci. Part A Polym. Chem., 2002, 40, 3212–3220 CrossRef CAS.
- T. Masuda, Polym. Rev., 2017, 57, 1–14 CrossRef CAS.
- J. Liu, J. W. Y. Lam and B. Z. Tang, Chem. Rev., 2009, 109, 5799–5867 CrossRef CAS PubMed.
- F. Freire, E. Quiñoá and R. Riguera, Chem. Rev., 2016, 116, 1242–1271 CrossRef CAS PubMed.
- K. Akagi, Chem. Rev., 2009, 109, 5354–5401 CrossRef CAS PubMed.
|
This journal is © The Royal Society of Chemistry 2024 |
Click here to see how this site uses Cookies. View our privacy policy here.