DOI:
10.1039/D4SC05489J
(Perspective)
Chem. Sci., 2024,
15, 17739-17759
A perspective on next-generation hyperfluorescent organic light-emitting diodes
Received
15th August 2024
, Accepted 7th October 2024
First published on 8th October 2024
Abstract
Hyperfluorescence, also known as thermally activated delayed fluorescence (TADF) sensitized fluorescence, is known as a next-generation efficient and innovative process for high-performance organic light-emitting diodes (OLEDs). High external quantum efficiency (EQE) and good color purity are crucial parameters for display applications. Hyperfluorescent OLEDs (HF-OLEDs) take the lead in this respect as they utilize the advantages of both TADF emitters and fluorescent dopants, realizing high EQE with color saturation and long-term stability. Hyperfluorescence is mediated through Förster resonance energy transfer (FRET) from a TADF sensitizer to the final fluorescent emitter. However, competing loss mechanisms such as Dexter energy transfer (DET) of triplet excitons and direct charge trapping on the final emitter need to be mitigated in order to achieve fluorescence emission with high efficiency. Despite tremendous progress, appropriate guidelines and fine optimization are still required to address these loss channels and to improve the device operational lifetime. This perspective aims to provide an overview of the evolution of HF-OLEDs by reviewing both molecular and device design pathways for highly efficient narrowband devices covering all colors of the visible spectrum. Existing challenges and potential solutions, such as molecules with peripheral inert substitution, multi-resonant (MR) TADF emitters as final dopants, and exciplex-sensitized HF-OLEDs, are discussed. Furthermore, the operational device lifetime is reviewed in detail before concluding with suggestions for future device development.
1. Introduction
The study of organic light-emitting diodes (OLEDs) is considered one of the research fields that equally drive both academia and industry at the same time. Since the fabrication of the first OLED device by Tang and VanSlyke,1 this forefront technology has come a long way and has evolved continuously to date. Especially in the display market, the field has rapidly expanded over the years as OLEDs can be integrated to enable self-emissive displays with very high pixel density (500 ppi and beyond) that provide true dark tones. Furthermore, OLEDs can be made transparent and flexible, which enables applications such as wearables, signage, or packaging that require form factors beyond rigid, opaque devices.2–4
In an OLED, the electrically generated holes and electrons recombine in the emissive layer and produce 25% excitons in the singlet state and the remaining 75% in the triplet state, according to spin statistics.5 Initial research started with fluorescent emitters due to their simple structures, which can exhibit unity photon conversion efficiency in the photoluminescence process upon optical excitation.1,6,7 However, upon electrical excitation, fluorescent emitters can utilize only singlet excitons to emit light, while triplet excitons are lost by non-radiative processes, resulting in a maximum internal quantum efficiency (IQE) of 25%.8–11 The external quantum efficiency (EQE) of an OLED can be calculated from the expression:12–15
where
φPL is the effective radiative efficiency of the emitter,
ηr is the so-called spin factor (the theoretical limit of emissive excitons; 25% for a fluorescent OLED),
γ is the recombination efficiency, ideally 100%, and
ηop is the optical outcoupling efficiency, which can reach approximately 20% if layer thicknesses are optimized such that the emitter dipoles are situated at the optical maximum of the electromagnetic field, assuming isotropic emitter orientation. Even if the effective radiative efficiency (based on a very high photoluminescence quantum yield (PLQY)) is considered to be unity, the maximum EQE for fluorescent OLEDs is limited to only 5%. Complete utilization of the generated excitons is crucial to obtain high-efficiency OLEDs. To improve the EQE from 5%, heavy metal complexes such as iridium and platinum-based phosphorescent emitters were developed.
16–18 The noble metal atoms can promote efficient spin–orbit coupling (SOC) by mixing singlet and triplet states, thus enabling the triplet excitons to emit and harvest all generated excitons.
17,19,20 However, the high-EQE phosphorescent emitters are expensive and do not satisfy production costs.
21 Moreover, the stability of blue phosphorescent emitters is a major issue due to their short operational lifetime.
22,23 Over the decades, purely organic thermally activated delayed fluorescence (TADF) emitters have emerged, realizing 100% IQE through reverse intersystem crossing (RISC) ensuing from a small energy gap (Δ
EST ≲ 200 meV) between the lowest excited singlet (S
1) and triplet (T
1) states.
24–28 The device performance of TADF OLEDs gradually matched with that of phosphorescent OLEDs and even reached an EQE of up to 40%.
29,30 However, TADF emitters have long triplet exciton lifetimes,
31 causing poor device stability and severe efficiency roll-off at high luminance.
32 Also, TADF emitters have a broad spectrum due to their intramolecular charge transfer (ICT) state, resulting in low color purity.
33 Therefore, new approaches are essential to resolve these challenges and to further enhance efficiency, lifetime, and color purity.
Fig. 1 shows the timeline and properties of various generations of OLED emitters.
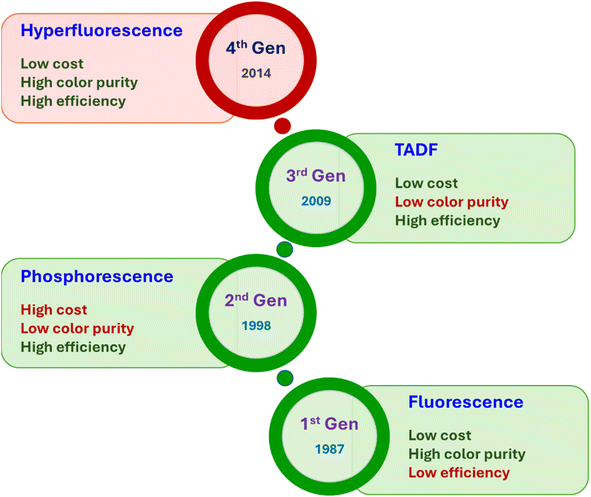 |
| Fig. 1 Timeline and properties of the different generations of OLED emitters. | |
Compared to phosphorescent and TADF emitters, conventional fluorescent emitters usually show narrowband emission with a full width at half maximum (FWHM) value of the OLEDs being less than 70 nm.34,35 A narrow spectrum is essential for obtaining color saturation, which is a critical requirement for the display industry. Yet, the problem of low-efficiency OLEDs still remains with fluorescent emitters. Recently, there has been a big leap in the device performance in fluorescent OLEDs by harvesting triplet excitons through an energy transfer mechanism from an assistant dopant or a sensitizer. This energy transfer approach is known as hyperfluorescence or TADF-sensitized fluorescence, which is becoming a cornerstone in developing an alternative strategy for next-generation high-performance OLED technology. The hyperfluorescent OLED (HF-OLED) technology combines a highly efficient TADF emitter as a sensitizer and a narrowband fluorescent emitter.36,37 Due to their spectral behaviour (Fig. 2a), hyperfluorescence could be the solution for OLED displays38 as it utilizes the advantages of both materials, holding enormous potential to achieve high EQE with narrowband emission and high stability. The primary energy transfer process is Förster energy transfer (FRET) from the lowest excited singlet state of the TADF emitter to the lowest singlet state of the final fluorescent dopant. However, during the process, several energy loss channels, such as Dexter energy transfer (DET) from the triplet state of the TADF emitter to the triplet state of the fluorescent emitter and direct charge trapping on the final fluorescent emitter can also take place and need to be blocked as these can deteriorate the device performance.39,40 Research towards HF-OLEDs is ongoing to improve their efficiency and lifetime. The confinement of all generated excitons in the multicomponent emissive layer and reduced carrier accumulation at the interfaces are also essential for maximizing the device performance. A simplified device structure of multilayer OLEDs is shown in Fig. 2b.
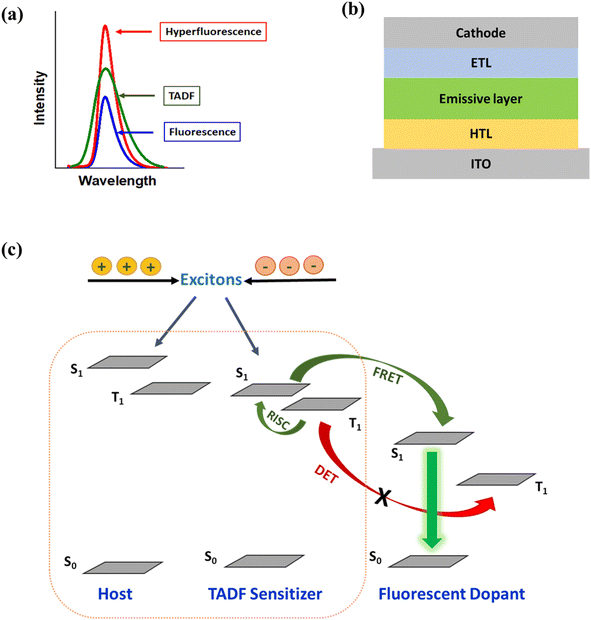 |
| Fig. 2 (a) Spectral behaviour of fluorescent, TADF and hyperfluorescent devices. (b) A simplified device structure of multilayer organic light-emitting diodes: ITO (indium tin oxide)/HTL (hole transporting layer)/emissive layer/ETL (electron transporting layer)/cathode. (c) A schematic diagram of energy transfer processes in a hyperfluorescent device. | |
In this perspective, the evolution of HF-OLEDs across all emission colors and current challenges are discussed in detail. Several recent approaches have been investigated to improve the overall performance of the multilayer HF-OLEDs. With color purity being a crucial parameter for display applications, various narrowband emitters which can be used as a final dopant are mentioned. HF-OLEDs can harvest triplet excitons, thereby reducing the excited state lifetime of the emissive layer and minimizing degradation. With the help of appropriate molecular designs and device engineering, these devices have the potential to achieve high EQE and extended operational lifetimes. This perspective focuses on material requirements, device structures and the suppression of energy loss mechanisms to achieve high-performance HF-OLEDs. There are many competitive potential materials that have emerged as sensitizers for fluorescent OLEDs, such as organic phosphorescent dyes41,42 and hybridized local and charge-transfer (HLCT) emitters;43,44 however, this perspective will focus solely on TADF-based sensitizers and their contribution to HF-OLEDs. Finally, an outlook providing suggestions to further improve the performance of HF-OLEDs is presented.
2. Working principle
The concept of hyperfluorescence combines a host, a highly efficient TADF material, and a narrow-spectrum fluorescent emitter that can achieve 100% IQE. The detailed mechanism of hyperfluorescence is shown in Fig. 2c. Upon electrical excitation, charge carriers are injected, which recombine to form excitons in the singlet and triplet states. The role of the TADF molecule is to enable efficient up-conversion of excitons from the triplet state into the singlet state via RISC and then to transfer the singlet state exciton to the final fluorescent guest emitter, where it will decay and emit a photon. In this approach, the TADF material, which is introduced as an assistant dopant or sensitizer, should have a high PLQY and must possess a high RISC rate in order to efficiently up-convert and harvest all excitons that are formed. Eventually, the fluorescent emitter is now capable of achieving three times higher device efficiency than conventional fluorescent devices that do not use sensitizers.45 The singlet and triplet energy levels of the materials should be appropriately aligned to maximize exciton confinement in the multicomponent emissive layer, and the triplet energy level of the host should be higher than those of both TADF and fluorescent emitters to prevent energy back transfer.
The main energy transfer process in an HF system is the long-range FRET process. Efficient FRET can be realized by ensuring sufficient spectral overlap between the emission spectrum of the TADF sensitizer and the absorption spectrum of the final fluorescent emitter. For efficient hyperfluorescence, the fraction of excited state energy that is transferred from the TADF sensitizer to the fluorescent emitter should be sufficiently high in order to achieve high FRET efficiency.46 This also minimizes potential emission from the TADF sensitizer by proper FRET management, hence increasing the color purity of the HF-OLEDs. The doping concentration of the fluorescent emitter in the emissive layer should be optimized in a way that ensures singlet energy transfer while minimizing loss processes like DET of triplet excitons and direct charge trapping.47 The energy transfer process between the sensitizer and final dopant can be explained with the help of FRET theory.48 The rate constant of the FRET (kFRET) process can be expressed as:
where
τD is the prompt decay lifetime of the TADF sensitizer in the absence of the final dopant,
R is the average molecular distance between the TADF sensitizer and fluorescent emitter, and
R0 is the Förster radius. Furthermore, the energy transfer efficiency (
ΦET) from the TADF sensitizer to the fluorescent emitter can be calculated from:
with
Here,
κ2 is the relative dipole orientation factor, taken as 2/3 assuming isotropic orientation,
49n is the refractive index, assumed to be 1.8 for most organic materials,
50NA is Avogadro's constant,
φf is the PLQY of the TADF sensitizer, and
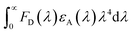
is the spectral overlap integral between the PL emission of the sensitizer with the absorption of the final dopant.
51 Thus, for high
ΦET, the spectral overlap integral and PLQY of the sensitizer need to be maximized. A highly efficient TADF sensitizer with an efficient FRET would suppress the loss processes and enable the development of high-performance OLEDs.
3. Evolution of hyperfluorescent OLEDs
Adachi and co-workers demonstrated the first HF-OLED in 2014, in which they used TADF molecules as sensitizers or assistant dopants to transfer electrically generated excitons to the final fluorescent emitters.36 The group reported highly efficient blue, green, red, and yellow HF-OLEDs with EQEmax ranging from 13% to 18%. Following that, several groups used this strategy to develop high-efficiency and pure color OLEDs. Kyulux also demonstrated impressive HF-OLEDs with narrow emission, providing high color purity.38 In order to enhance the OLED performance further, various modifications in the molecular design and in device structures have been investigated. Here, we will discuss the evolution of HF-OLEDs based on fluorescent emitters, covering the primary colors blue, green, and red, as well as white light devices. Some of the molecular structures of TADF sensitizers and fluorescent dopants are shown in Fig. 3a and b. The performances of HF-OLEDs using fluorescent dopants are summarized in Table 1.
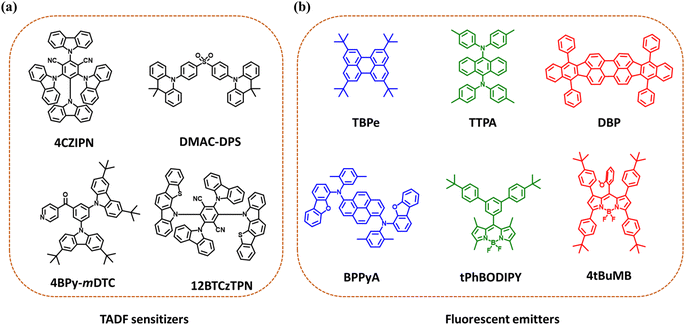 |
| Fig. 3 (a) Molecular structures of TADF emitters used as sensitizers for hyperfluorescent OLEDs. (b) Molecular structures of various blue, green and red fluorescent emitters used as final dopants. | |
Table 1 Hyperfluorescent OLEDs based on fluorescent dopants
Final dopant |
Sensitizer |
Host |
EL (nm) |
FWHM (nm) |
EQEmax/1000 cd m−2 (%) |
PEmax/1000 cd m−2 (lm W−1) |
CIE |
Ref. |
TBPe |
ACRSA |
DPEPO |
— |
— |
13.4/8.7 |
18/7 |
(0.17, 0.30) |
36
|
TTPA |
ACRXTN |
mCP |
— |
— |
15.8/11.7 |
47/30 |
(0.29, 0.59) |
36
|
TBRb |
PXZ-TRZ |
mCBP |
— |
— |
18.0/17.2 |
58/33 |
(0.45, 0.53) |
36
|
DBP |
Tri-PXZ-TRZ |
CBP |
— |
— |
17.5/10.9 |
28/10 |
(0.61, 0.39) |
36
|
TBPe |
CzAcSF |
— |
— |
— |
15.4/10.7 |
23.4/9.7 |
(0.15, 0.23) |
52
|
TBPe |
CzAcSF |
DPEPO |
— |
— |
18.1 |
31.2 |
(0.15, 0.22) |
52
|
TBPe |
tDCztTrz |
DPEPO |
— |
— |
13.7 |
— |
— |
53
|
TBPe |
TpAT-tFFO |
CzSi |
462 |
— |
18.7/17.1 |
— |
(0.15, 0.23) |
54
|
TBPe |
5Cz-TRZ |
mCBP |
— |
— |
24.0 |
— |
— |
55
|
TBRb |
5Cz-TRZ |
mCBP |
— |
— |
24.9 |
— |
— |
55
|
BPPyA |
DMAC-DMT |
DBFPO |
458 |
— |
19.0 |
— |
(0.14, 0.15) |
56
|
DNTBPe |
4TCzBN |
DOBNA-OAr |
468 |
63 |
32.7/18.8 |
47.7/17.7 |
(0.14, 0.21) |
57
|
TBPe |
4TCzBN |
DOBNA-OAr |
463 |
63 |
24.0/12.6 |
35.0/12.7 |
(0.15, 0.23) |
57
|
TBPe |
4TCzBN |
DCz-BTP |
466 |
— |
20.5/16.9 |
34.7/19.6 |
— |
58
|
6tBPA |
FTrzTCz |
DPEPO |
517 |
— |
17.9/14.0 |
— |
(0.24, 0.58) |
59
|
6tBPA |
TbCzTrz |
DPEPO |
— |
— |
14.6/9.2 |
— |
(0.25, 0.57) |
60
|
6tBPA |
BPAc |
DPEPO |
— |
— |
16.6/15.2 |
36.0/22.3 |
(0.23, 0.51) |
61
|
TAA-PPO |
4BPy-mDTC |
mCBP |
518 |
— |
17.8 |
48.1 |
(0.23, 0.58) |
62
|
C545T |
PXZ-DPS |
DMAC-DPS |
— |
— |
11.1 |
21.4 |
— |
63
|
PhtBuPAD |
PXZ-DPS |
PhCzTrz |
— |
— |
24.0/23.8 |
71.4/52.3 |
(0.36, 0.58) |
64
|
C545T |
3BPy-mDTC |
mCBP |
508 |
— |
23.0 |
69.9 |
(0.20, 0.56) |
65
|
tPhBODIPY |
4CzIPN |
DCZDCN |
— |
32 |
19.0/18.9 |
85.7/78.3 |
(0.26, 0.67) |
66
|
tPhBODIPY |
4CzIPN |
mCBP |
— |
32 |
18.8/18.6 |
74.5/53.3 |
(0.28, 0.67) |
67
|
DBP |
TXO-TPA |
mCBP |
— |
— |
16.9/2.6 |
27.8/1.8 |
(0.65, 0.35) |
68
|
DBP |
2,7-TXO-PhCz |
CBP |
— |
— |
15.9/4.9 |
31.2/5.5 |
(0.52, 0.45) |
69
|
4tBuMB |
4CzTPN |
DIC-TRZ |
617 |
44 |
19.4/17.2 |
— |
(0.64, 0.36) |
70
|
4tBuMB |
12BTCzTPN |
DIC-TRZ |
618 |
46 |
19.9/16.7 |
— |
(0.64/0.36) |
71
|
DBP |
FPXZ-DBPZ |
CBP |
— |
— |
18.1/— |
26/- |
(0.61, 0.38) |
72
|
DBP |
2BT12CzINN |
PBICT:DBTTP1 |
614 |
— |
14.7 |
12.7 |
(0.60, 0.39) |
73
|
TBRb |
4CzIPN-Me |
mCBP |
— |
— |
19.1/16.7 |
— |
(0.43, 0.54) |
74
|
TBRb |
PyCNTruX |
PBICT |
— |
— |
20.2 |
50.7/46.5 |
(0.45, 0.53) |
75
|
![[thin space (1/6-em)]](https://www.rsc.org/images/entities/char_2009.gif) |
Solution-processed HF-OLED
|
TBPe |
5CzCN |
DPOBBPE |
— |
— |
18.8 |
14.3 |
(0.14, 0.20) |
76
|
DBP |
DC-TC |
CBP |
— |
— |
8.0 |
— |
(0.61, 0.38) |
77
|
5TBUPH-BODIPY |
phCz-4CzTPN |
— |
616 |
47 |
4.9 |
— |
(0.64, 0.36) |
78
|
Cibalackrot |
4CzIPN-tBu |
CBP |
— |
— |
15.3/8.4 |
— |
— |
79
|
3.1 Blue hyperfluorescent OLEDs
High efficiency and long lifetime remain significant challenges for blue OLEDs as compared to red, yellow, and green OLEDs, because the wider bandgap of blue light leads to faster material degradation and, hence, shortened device operational lifetime. This is particularly true for deep blue phosphorescent and TADF emitters,23,80,81 where the high emission energy and long triplet exciton lifetime82 contribute to the generation of highly reactive ‘hot excited states’ through exciton annihilation processes.83 These are also lethal to the surrounding organic materials, thus particularly restricting device operational lifetimes. Fluorescent emitters instead possess much lower exciton lifetimes, which strongly improve device stability. For this reason, they are still in use for practical display and lighting applications, even though they provide much lower EQE.
Utilizing the benefits of fluorescent emitters, HF-OLEDs came into existence. The first HF blue OLED demonstrated by Nakanotani et al. showed an efficiency of 13% using the TADF sensitizer ACRSA with the fluorescent emitter TBPe.36 With time, various new molecular designs for blue TADF molecules have emerged, which can be utilized as sensitizers to deliver improved performance. Song et al. reported a blue-emitting TADF compound, CzAcSF, as the triplet-sensitizing host to efficiently transfer emission energy to TBPe without any external host.52 The CzAcSF molecule consisted of a weak diphenyl sulfone acceptor with moderate acridine and carbazole donors, which increased the band gap of CzAcSF towards deep blue emission. The EQEmax for the HF system was 15.4%, showing an enhancement from the ACRSA:TBPe system discussed above.36 The efficiency of the blue HF-OLED increased to 18.1% by incorporating the wide band gap DPEPO host in the emissive layer.84 Kang et al. designed two TADF sensitizers, tDCztTrz and tDCz2tTz, based on a triazine derivative with peripheral alkyl branches around the core, which led to an EQE of 13.7% with the TBPe emitter.53 The fast RISC rate for the TADF sensitizer is an essential factor for developing efficient HF-OLEDs. Hence, Wada et al. designed a TADF emitter, TpAT-tFFO, with a tilted alignment of the donor and acceptor, which exhibited fast RISC (107 s−1) due to near-degenerate charge transfer and locally excited triplet states.54 This fast RISC reduces the concentration of triplet excitons and various annihilation events in the emissive layer. The device achieved an EQEmax of 18.7% while sensitizing TBPe with a reduced roll-off and retaining the EQE of 11.8%, even at a high luminance of 10
000 cd m−2 (Fig. 4a–c). Cui et al. reported a sky-blue TADF molecule, 5Cz-TRZ, which formed charge resonance type triplet states leading to a small ΔEST and a fast RISC rate (107 s−1).55 The EQEmax achieved for the HF-OLED with TBPe was 24.0%. New emitters for final dopants have also emerged to contribute to the HF system. The main requirements are (1) a narrow blue emission spectrum with a wavelength range of 440–470 nm, (2) a small Stokes' shift, and (3) a large spectral overlap with TADF emission. Based on these norms, Ahn et al. developed high-efficiency deep-blue HF-OLEDs using BPPyA as a blue fluorescent emitter, which showed an emission maxima of 458 nm with high PLQY (98%).56 Optimized HF-OLEDs were fabricated using the blue TADF emitters DMAC-DPS, DMAC-DMT and SPAC-DMT as sensitizers. The devices exhibited deep-blue emission from BPPyA, and a maximum EQE of 19.0% was achieved using DMAC-DMT as a sensitizer (Fig. 4d–f). Very recently, Wu et al. designed a highly efficient fluorescent emitter by doping nitrogen atoms into polycyclic aromatic hydrocarbons of the TBPe emitter, named DNTBPe.57 The HF devices were fabricated using the DOBNA-OAr host, 4TCzBN as the TADF sensitizer, achieving an EQEmax of 32.7% for DNTBPe and 24.0% for TBPe final dopants.
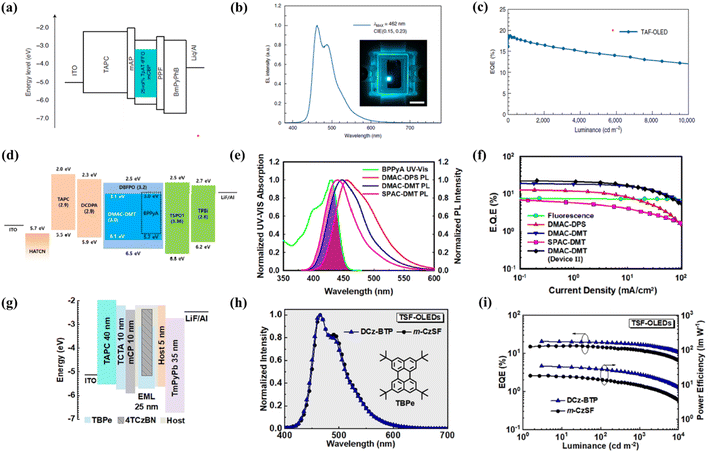 |
| Fig. 4 Blue hyperfluorescent OLEDs. (a–c) Device structure, electroluminescence spectra and efficiency of the mCP:TpAT-tFFO:TBPe emissive system. Reproduced with permission from ref. 54, Nature, 2020. (d–f) Device structure using DPEPO:DMAC-DMT:BPPyA as an emissive layer, overlap of absorption of BPPyA with the emission spectra of the TADF sensitizers and EQE of all the devices. Reproduced with permission from ref. 56, American Chemical society, 2018. (g–i) Device structure of the hyperfluorescent device with the 4TCzBN sensitizer and TBPe emitter, electroluminescence spectra and EQE–luminance–power efficiency plots. Reproduced with permission from ref. 58, John Wiley and Sons, 2022. | |
Although there has been great interest in exploring new blue emitters for HF-OLEDs, the development of host materials for blue devices has considerably lagged. Wang et al. reported a pyridine-fused bipolar host, DCz-BTP, which showed a low turn-on voltage of 2.8 V and a maximum EQE of 20.5% using the 4TCzBN sensitizer and TBPe emitter as shown in Fig. 4g–i.58 The pseudo-symmetric structure of the DCz-BTP host enabled lower driving voltage and enhanced electroluminescence performance as compared to the commonly used traditional hosts such as DPEPO and mCBP. Moreover, for solution-processed blue HF-OLEDs, these traditional hosts performed poorly due to their poor solubility. Jeon et al. developed a new soluble, high-triplet energy host, DPOBBPE, and demonstrated the first solution-processed blue HF-OLED with the blue TADF compound 5CzCN and TBPe to achieve an efficiency above 18%.76
3.2 Green hyperfluorescent OLEDs
Yun et al. demonstrated a green HF-OLED with a 6tBPA fluorescent emitter and a triazine derivative sensitizer, FTrzTCz showing an EQE of 17.9%. Incorporating fluorine in the acceptor core of the TADF sensitizer has induced strong charge transfer properties and a fast RISC process.59 6tBPA has also been doped with sky-blue TADF sensitizers, TbCzTrz and BPAc, resulting in reasonable performances with EQEs of 14.6% and 16.6%, respectively.60,61 One of our studies reported an anthracene-based green emitter, TAA-PPO, with an EQE of 7.2% without any sensitizer, and later the device performance was improved up to 17.8% using 4BPy-mDTC as the TADF sensitizer.62 Li et al. reported an enhancement of EQE from 9.0% to 11.1% by introducing a synergetic effect using dual-TADF hosts.63 The introduction of the second TADF emitter established an additional sensitizing route, thereby increasing the FRET rate and reducing exciton loss processes in the HF system. Zhang et al. reported a series of green dyes involving N9,N9,N10,N10-tetraphenylanthracene-9,10-diamine (PAD) as the emissive core.64 All the emitters (PAD, MePAD, tBuPAD, and PhtBuPAD) showed high PLQY (80–89% in toluene solution) and performed well when doped into the TADF sensitizer, PXZ-DPS, for green HF-OLEDs. The HF device achieved an EQE of 24% for the PhtBuPAD-based final emitter. In a recent report, we discussed the importance of appropriate material combinations for green HF-OLEDs by systematic investigation. Optimized energy gaps between the TADF sensitizers and final fluorescent emitters are crucial to improve the performance and reduce EQE roll-off of the devices.65 Recently, a family of well-known fluorescent emitters, tetracoordinate boron-dipyrromethene (BODIPY) derivatives, have emerged as suitable candidates for use as the final emitter in HF systems.85,86 BODIPY materials demonstrated high molar extinction coefficients, high PLQY (>90%), and narrowband emission (25–35 nm).87,88 Using these benefits, Song et al. developed an ultrapure green HF-OLED using a BODIPY derivative, named tPhBODIPY, as the final dopant, achieving an EQE of 18.9% at a practical luminance of 1000 cd m−2 with a narrow FWHM of 32 nm using the TADF sensitizer 4CzIPN (Fig. 5a–c).66 Nakamura et al. also reported highly efficient (EQE: 19%) and stable pure green HF-OLEDs with tPhBODIPY and 4CzIPN by using triple hole transporting layers (HTLs) in the device structure to reduce carrier accumulation at the layer interface.67
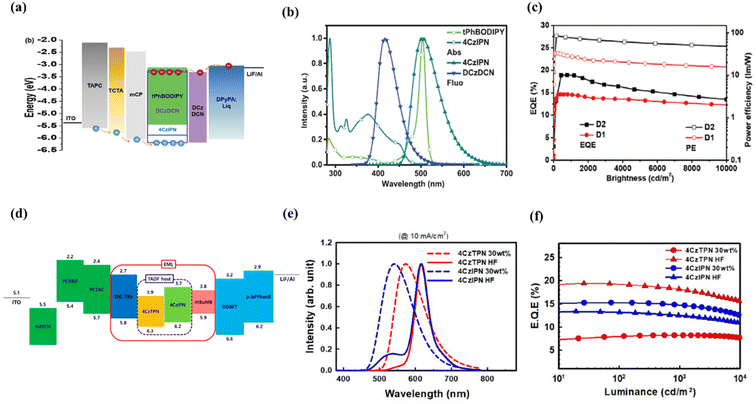 |
| Fig. 5 (a–c) Green hyperfluorescent OLEDs. The device structure used in fabrication, absorption and emission spectra of tPhBODIPY and 4CzIPN and the efficiency plot of the devices. Reproduced with permission from ref. 66, John Wiley and Sons, 2020. (d–f) Red hyperfluorescent OLEDs. The device structure of the HF-OLED, electroluminescence spectra of TADF-based and HF-based OLEDs and EQE vs. luminance of the devices. Reproduced with permission from ref. 70, American Chemical Society, 2021. | |
3.3 Red hyperfluorescent OLEDs
There are limited reports on high-performance metal-free red emitters. The main challenge with red materials is their rather low PLQY due to the energy gap law.89–91 Hence, the design and synthesis of efficient red TADF emitters for conventional TADF-OLEDs face substantial challenges. For a red TADF device, a high singlet radiative rate constant (kSr) is required, which can be achieved using planar structures, i.e. overlap of the frontier molecular orbitals (FMO), which could lead to a large ΔEST and, thus, the simultaneous realization of a large kSr and small ΔEST is challenging.39,92–94 Hence, the TADF materials with a small ΔEST are used as sensitizers for traditional red fluorescent emitters that possess a large kSr to achieve efficient red HF-OLEDs. Adachi et al. reported the first red HF system using the TADF molecule tri-PXZ-TRZ as a sensitizer with the well-known fluorescent red emitter DBP and achieved a maximum EQE of 17.5%.36 The DBP emitter has been widely used in the HF system, and OLEDs have been reported with an EQE of 16.9% with the TADF sensitizer TXO-TPA and 15.9% with the sensitizer 2,7-TXO-PhCz.68,69 In both cases, more than 90% energy transfer efficiency was reported from S1 of the TADF sensitizer to that of DBP, which benefitted in accelerating the RISC process. Red BODIPY materials have also been explored as the final dopant in HF devices. Jung et al. fabricated HF-OLEDs using the red BODIPY dopant, 4tBuMB with the TADF sensitizer 4CzTPN and achieved an EQE of 19.4% with a FWHM of 44 nm (Fig. 5d–f).70 When the assistant dopant was modified to 12BTCzTPN, the device efficiency was slightly enhanced to 19.9% with the same final dopant, 4tBuMB.71 Several solution-processed red HF devices have been fabricated with both DBP and BODIPY dopants. Using DC-TC as a sensitizer for DBP, the HF device showed an EQE of 8.0%, while the 5TBUPH-BODIPY emitter with the phCz-4CzTPN sensitizer could achieve a maximum EQE of 4.9%.77,78 Another fluorescent dopant based on the red organic soluble dye cibalackrot was utilized as the final emitter for the solution-processable HF device. The flat and rigid structure of the dye exhibited a high PLQY (96%) and a narrow spectrum (FWHM 37 nm), making it an ideal candidate for electroluminescent devices.95 Wallwork et al. reported an EQE of 15.3% for the solution-processed red HF OLEDs using the cibalackrot dye.79 Further development of highly efficient organic-based red devices is still in progress, and it would be interesting to see if these metal-free emitter devices could be incorporated into commercial applications.
3.4 White hyperfluorescent OLEDs
Hybrid full-organic white HF-OLEDs based on TADF emitters and fluorescent dopants have gained significant attention for achieving good color quality and high electroluminescence efficiency due to their potential in display panels and general lighting applications.96–98 One crucial aspect of developing white OLEDs for lighting applications is the color rendering index (CRI), a parameter that indicates how accurately colors of objects are reproduced under particular illumination conditions.99,100 The CRI should be as high as possible with values above 80 typically being considered suitable for practical lighting applications.101–103 For achieving such a high CRI, white light sources require broad and continuous emission across the entire visible spectrum.104 For a monochromatic HF device, emission arises only from the fluorescent emitter, while for white light, sufficient and well-balanced emission should occur from both TADF and fluorescent molecules (Fig. 6).
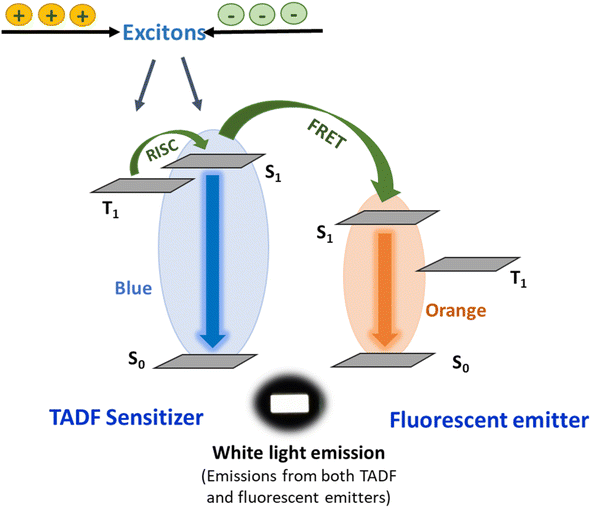 |
| Fig. 6 A schematic representation of white light emission from both the TADF sensitizer and fluorescent emitter. | |
White HF-OLEDs can be constructed using a single emissive layer (EML) or multiple emissive layers. Single EML white HF-OLEDs have simple device structures, where white light can be obtained by using the complementary color of the TADF sensitizer and fluorescent emitter. DMAC-DPS was widely used initially for blue emission with conventional emitters rubrene and TBRb as corresponding dopants.105–107 Wu et al. demonstrated a single EML white HF-OLED with DMAC-DPS and TBRb, achieving a maximum EQE of 14.6%.108 Later, various new blue TADF materials, such as 2tCz2CzBn (Fig. 7a and b), 3Ph2CzCzBn, and mSOAD have been developed for fabricating single EML devices using rubrene and TBRb.109,113,114 However, it was found that FRET from the TADF to fluorescent dopant becomes more effective than the direct emission from TADF molecules, resulting in poorly balanced white light. Moreover, the blue emission peak in the EL spectra also varied with increasing luminance of the device, indicating unstable color. To overcome these issues, the use of multiple emissive layers or the introduction of interlayers has been suggested.
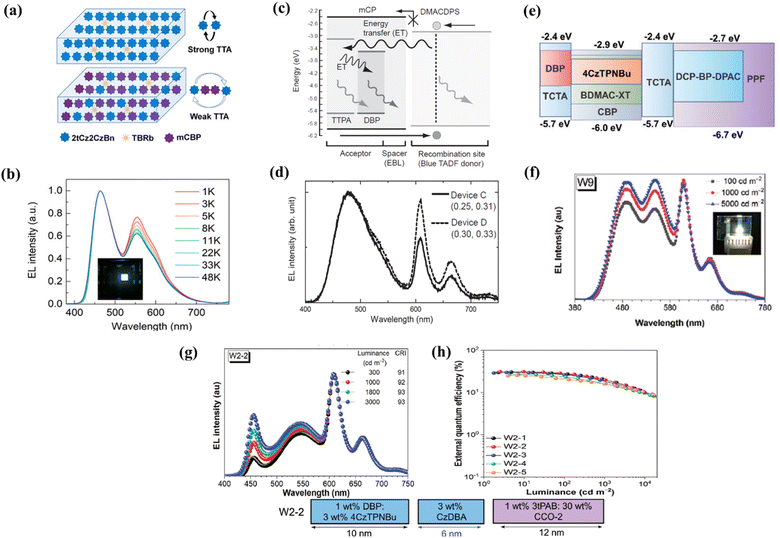 |
| Fig. 7 White hyperfluorescent OLEDs. (a and b) Schematic representation of 2tCz2CzBn:TBRb and mCBP:2tCz2CzBn:TBRb in the single emissive layer white OLEDs and electroluminescence spectra at various luminances. Reproduced with permission from ref. 109, American Chemical Society, 2020. (c and d) Schematic illustration of the energy transfer mechanism under electrical excitation with a 2 nm mCP emissive layer and electroluminescence spectra of the white device. Reproduced with permission from ref. 110, John Wiley and Sons, 2015. (e and f) Device structure using multiple emissive layers and electroluminescence spectra recorded at different luminances. Reproduced with permission from ref. 111, John Wiley and Sons, 2021. (g and h) Electroluminescence spectra and EQE of white OLEDs based on the interlayer sensitization strategy. Reproduced with permission from ref. 112, John Wiley and Sons, 2023. | |
Multiple emissive layer white HF-OLEDs consisting of more than one emissive layer are known to achieve better efficiency and CRI than single EML white OLEDs. Wu et al. fabricated an efficient multi-EML device employing three emissive layers, DMAC-DPS:0.5 wt% DBP, DMAC-DPS:1 wt% DBP:0.5 wt% TTPA and DMAC-DPA:0.2 wt% TTPA and achieved a maximum EQE of 18.2% and a CRI of 82.115 This sandwich-type emissive structure broadened the recombination region to reduce annihilation processes and ensured adequate blue emission. Another report showed EQE above 20% by carefully selecting emitters and layer thicknesses for blue and yellow EMLs. Here, the triplet state of the blue fluorescent emitter 4P-NPD matched well with the excited states of the green TADF molecule (PXZ-TRZ) used in the yellow EML (TBRb dopant), resulting in high exciton utilization.116 The performance of multi-EML white HF-OLEDs could also be enhanced by inserting a thin interlayer or spacer layer between the EMLs. Higuchi et al. reported an improvement in efficiency from 12.0% to 16.0% by adding a thin mCP spacer layer of 2 nm between the two emissive layers (Fig. 7c and d).110 The interlayer widens and adjusts the exciton recombination zone, preventing direct recombination on the fluorescent dopant. It helps the generated excitons to smoothly transfer to the adjacent layers and tune the color intensity of each EML for a high CRI value.117 The interlayer between the emissive layers may even be introduced in three-color or four-color white OLEDs (Fig. 7e and f) in order to control the exciton management and thus provide a high CRI and EQE.111,118 Recently, Liu et al. demonstrated an outstanding EQE of 30.8% and a power efficiency of 110.7 lm W−1 in a purely organic white OLED based on interlayer sensitization using the sky-blue emitter TCP-BP-SFAC, orange layer 4CzTPNBu and the red fluorescent dopant DBP.119 The group reported another white OLED showing a comprehensive high EQE of 31.0% and a remarkable CRI of 93 using the interlayer sensitization strategy.112 The synergistic effect of the adjacent TADF sensitizing layer (3 wt% CzDBA:DMAC-TRZ) combined with a low concentration of the TADF electron capturing agent 4CzTPNBu, co-doped with the red emitter DBP helped in achieving high device performance (Fig. 7g and h). The device performances of different white HF-OLEDs are summarized in Table 2.
Table 2 White hyperfluorescent OLEDs
Emissive layer |
EQEmax/1000 cd m−2 (%) |
PEmax/1000 cd m−2 (lm W−1) |
CIE |
CRI |
Ref. |
Single emissive layer
|
DMAC-DPS:(0.6%) rubrene |
7.48/7.31 |
15.9/14.7 |
(0.359, 0.439) |
— |
105
|
DPEPO:(50%) DMAC-DPS:(0.03%) TBRb |
17.6/14.5 |
41.0/22.7 |
(0.23, 0.31) |
44.7 |
106
|
DPEPO:(50%) DMAC-DPS:(0.05%) TBRb |
15.5/13.3 |
39.3/23.4 |
(0.28, 0.35) |
58.6 |
106
|
DPEPO:DMAC-DPS:(0.2%) TBRb |
14.6/11.9 |
51.6/26.9 |
(0.34, 0.47) |
— |
108
|
mCBP:(30%) 2tCz2CzBn:(0.2%) TBRb |
21.8/14.2 |
43.9/15.7 |
(0.292, 0.343) |
— |
109
|
mSOAD:(0.8%) rubrene |
9.8 |
29.0 |
(0.30, 0.45) |
— |
113
|
mCBP:(20%) 3Ph2CzCzBN:(0.5%) TBRb |
20.9/13.5 |
— |
(0.31, 0.41) |
60 |
114
|
mCPBC:(10%) 5TCzBN:(1%) TBRb |
19.6/15.4 |
52.2/29.1 |
(0.33, 0.45) |
— |
120
|
![[thin space (1/6-em)]](https://www.rsc.org/images/entities/char_2009.gif) |
Multiple emissive layers
|
DMAC DPS:(0.5%) DBP/DMAC-DPS:(1%) DBP:(0.5%) TTPA/DMAC-DPA:(0.2%) TTPA |
18.2/16.2 |
44.6/27.2 |
(0.318, 0.390) |
82 |
115
|
SF4-TPE:(30%) PXZ-TRZ:(1%) TBRb/SF4-TPE:(40%) 4P-NPD |
24.5/23.5 |
65.4/50.8 |
(0.47, 0.49) |
— |
116
|
(1%) DBP:(10%) TTPA:mCP/mCP (2 nm)/DMAC-DPS |
12.1 |
22.0 |
(0.25, 0.31) |
74 |
110
|
(0.4%) TBRb:(6%) 4CzPN/(0.8%) TBRb:(10%) 4CzPN:mCBP:(40%) Bepp2:mCBP/Bepp2 (3 nm)/(5%) DSA-Ph:MADN |
15.1/12.1 |
47.4/25.1 |
(0.35, 0.49) |
49 |
118
|
(0.4%) DBP:(6%) 4CzPN/(0.8%) TBRb:(10%) 4CzPN:mCBP:(40%) Bepp2:mCBP/Bepp2 (3 nm)/(0.3%) DBP:(5%) DSA-Ph:MADN |
12.9/10.2 |
28.2/17.2 |
(0.42, 0.46) |
78 |
118
|
TCTA:(1%) DBP/CBP:(30%) BDMAC-XT:(2%) 4CzTPNBu/TCTA (2 nm)/PPF:(20%) DCP-BP-DPAC |
22.8/12.0 |
57.9/24.5 |
(0.273, 0.408) |
73 |
111
|
TCTA:(1%) DBP/CBP:(30%) BDMAC-XT:(4%) 4CzTPNBu/TCTA (2 nm)/PPF:(20%) DCP-BP-DPAC |
20.3/11.1 |
56.1/22.8 |
(0.340, 0.409) |
87 |
111
|
(1%) DBP:TCP-BP-SFAC/(1.5%) 4CzTPNBu:TCP-BPSFAC/TCP-BP-SFAC |
30.8/20.6 |
106.8/52.6 |
(0.394, 0.476) |
— |
119
|
(1%) DBP:(3%) 4CzTPNBu:DMIC-TRZ/(3%) CzDBA:DMIC-TRZ/(1%) 3tPAB:(30%) CCO-2:mCBP |
31.0/20.0 |
80.5/33.6 |
(0.426, 0.412) |
93 |
112
|
4. Approaches to obtain high-performance HF-OLEDs
Obtaining high-performance HF devices is quite challenging as the FRET process is accompanied by competing loss channels. The two main efficiency loss mechanisms under electrical excitation in HF-OLEDs are (i) Dexter energy transfer from the triplet state of the sensitizer to the triplet state of the fluorescent emitter and (ii) direct charge trapping or carrier recombination on the fluorescent dopant. Both processes lead to the formation of triplet excitons on the final fluorescent emitter, from where they decay non-radiatively. These loss mechanisms not only affect efficiency but also the device lifetime since the long-lived triplet states may cause material degradation. Direct charge recombination on the fluorescent emitter can be minimized by doping the final dopant at very low concentrations (<1 wt%) into the emissive layer. Gottardi et al. studied the efficiency loss mechanisms in HF-OLEDs using Monte Carlo simulations and discussed the formation of charge-transfer states between the TADF sensitizer and the fluorescent molecule, which can substantially affect the EQE and roll-off characteristics.121 At lower voltages, the loss process is mainly attributed to the non-radiative triplet states, while at higher voltages, a large contribution arises from various annihilation processes, including singlet–triplet annihilation in the fluorescent emitter. Abroshan et al. studied the electronic transitions of a series of fluorescent dopants generally used in HF systems and found that if T2/T3 states of the fluorescent emitter are close to the S1 state, then those triplet states are typically similar in energy to T1(TADF). Thus, energy losses in the device arise via DET from T1(TADF) to T2/T3(fluorescent emitter).122 The work suggested a design principle for fluorescent dopants with large energy level differences between S1 and T2/T3 to effectively suppress DET. Hence, various strategies to suppress these energy loss processes have been reported and are described in the following.
4.1 Molecules with peripheral inert substitution
DET can be considered as the key loss process in HF-OLEDs.40 A fast RISC rate of the TADF sensitizer helps to reduce the triplet exciton density, hence controlling this loss process.123 Along with accelerated RISC, the intermolecular distance between the TADF sensitizer and the final fluorescent dopant plays a crucial role in minimizing DET. Fortunately, DET is a short-range energy transfer (∼1 nm), and the process can be effectively inhibited by increasing the distance between TADF and fluorescent molecules.20,124,125 One way to increase the intermolecular distance is through inserting electronically inert peripheral substitutions in the emitter (Fig. 8a) (either in the TADF sensitizer or in the fluorescent emitter or in both). Such bulky substitutions electronically shield the active core of the emitter, hindering orbital overlap and effectively managing DET by preventing intermolecular interactions between the sensitizer and the emitter (Fig. 8b).64 Zhang et al. observed the highest efficiency for PhtBuPAD-based HF devices, which have a bulky group in the molecular structure, among the series of PAD green emitters. The addition of the bulky unit in the final dopant proved to be efficacious in achieving high EQE and low roll-off by suppressing the energy loss paths from the TADF sensitizer to the final emitter.64,127 Xie et al. introduced an inert phenyl-fluorene unit onto the donor moiety of the TADF sensitizer to block energy loss pathways in HF-OLEDs.72 Molecular dynamics simulation results showed that the intermolecular distance between the molecules increased by 0.7 Å after adding the bulky group, which effectively decreased the DET rate and achieved a maximum EQE of 18.1% for red HF-OLEDs with narrow emission. Alam et al. reported a deep-blue emitter, KCTBC, with a twisted interlocked acceptor core framework, which showed an EQE of 13.9% with the sensitizer 4CzFCN.128 Bartkowski et al. introduced a new rational design by rigidifying the TADF sensitizer with π-extension conjugation and demonstrated a yellow HF-OLED showing an excellent EQE of 27% with narrowband emission (FWHM = 40 nm).129 Lee et al. very recently reported TADF emitters with isonicotinonitrile as the acceptor and benzothienocarbazole as the donor to create bulkiness in the molecules which can effectively block DET from the TADF sensitizer to the red fluorescent dopant DBP.73 The molecules suppressed DET, accelerated the RISC process, and achieved an EQE of 14.7% for red HF-OLEDs (Fig. 8c and d). Wei et al. reported single EML white HF-OLEDs with sterically shielded TADF emitters and fluorescent dopants, to modulate FRET and DET and achieved good electroluminescence performance.120 The electronically shielded peripheral units not only blocked DET to prevent triplet exciton quenching but also suppressed the FRET rate to the fluorescent dopant. Hence, they could retain the blue emission intensity from the TADF emitter for balanced white light.
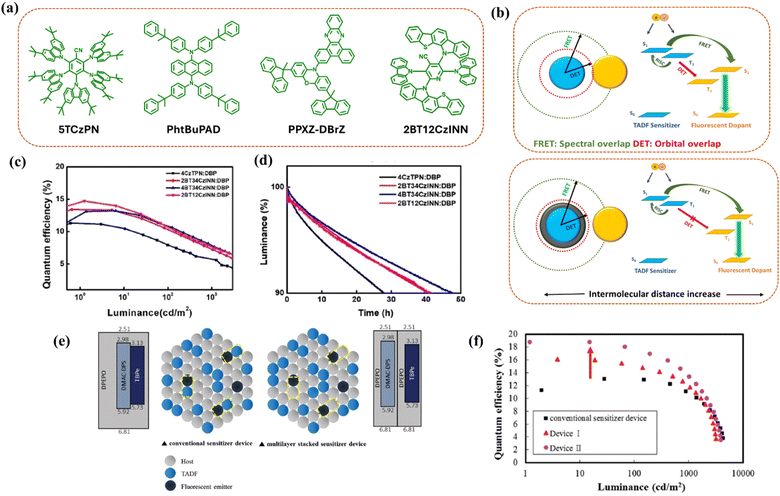 |
| Fig. 8 Peripheral inert substitution. (a) Molecular structures of emitters with bulky blocking peripheral units. (b) A schematic diagram of FRET and DET on intermolecular distances between the TADF sensitizer and the final fluorescent dopant along with the energy transfer processes without peripheral inert units (top) and with peripheral inert units (bottom). The blue and yellow spheroids represent the TADF sensitizer and fluorescent emitter, respectively, and the gray spheroid indicates the peripheral inert unit. (c and d) Efficiency vs. luminance plots and device lifetime of the HF-OLEDs based on TADF sensitizers with bulky blocking substitutions. Reproduced with permission from ref. 73, American Chemical Society, 2023. (e and f) Schematic illustration to manage Dexter energy transfer and quantum efficiency vs. luminance plots of the respective devices. Reproduced with permission from ref. 126, Royal Society of Chemistry, 2018. | |
Separating the molecules of TADF and the final dopant by inserting bulky blocking units seems to be an effective way to modulate the energy transfer mechanism in HF-OLEDs. Another way to manage DET is by constructing a multilayer emitting structure by spatially separating the sensitizer layer and the fluorescent emitter layer to confine all excitons similar to a quantum well structure.130,131 With the help of device engineering, Han et al. reported an improved efficiency from 13.1% to 18.8% by using simple molecules without any rigid unit (Fig. 8e and f).126 Jakoby et al. investigated the effect of singlet and triplet exciton diffusion lengths of TADF molecules in HF systems using Monte Carlo simulations.132 Since the concentration of the final emitter needs to be kept low, most of the excitons diffuse between the TADF molecules before reaching the final dopant. The work suggested two ways to improve HF efficiency: first, to incorporate steric protection in the emitter, as an unprotected emitter would increase the triplet motion and second, to develop TADF molecules with shorter triplet diffusion lengths as it could restrict triplet transport between the molecules.
4.2 Multi-resonant (MR) TADF emitters as final dopants
Lately, the development of organo-boron-based TADF dopants has caught attention due to their multi-resonance (MR) effect between boron and nitrogen (or oxygen) atoms which can minimize vibronic coupling and thus reduce the singlet–triplet energy gap (ΔEST).133 The electron-deficient boron and electron-rich nitrogen atoms were inserted into rigid polycyclic aromatic rings (DABNA-1 and DABNA-2).134 The boron and nitrogen atoms were placed in such a way that they induced an opposite resonance effect/multi-resonance character in the molecule. In this unique molecular design, unlike conventional TADF emitters, FMOs were distributed over the atoms with LUMOs on boron and carbon adjacent to nitrogen and HOMOs on nitrogen and carbon adjacent to boron. This atomic separation of FMOs leads to a small ΔEST, large oscillator strength, and narrow emission (FWHM ≤25 nm).135–138 This class of emitters is called MR-TADF emitters (Fig. 9a) and may achieve high efficiency in TADF OLEDs without compromising on the color purity of the emission.
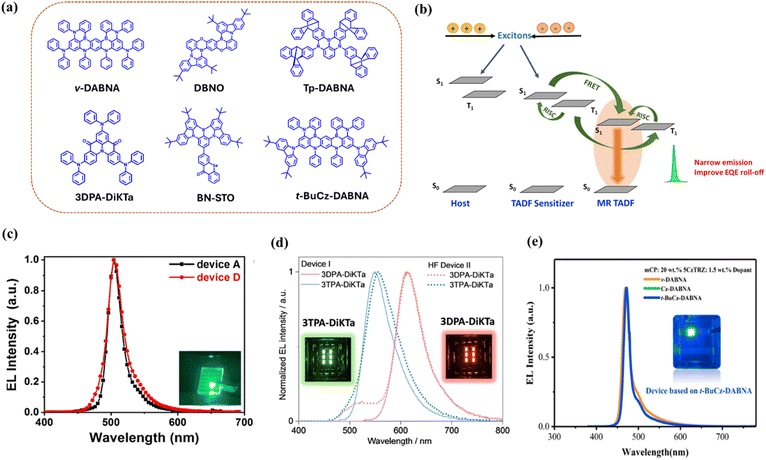 |
| Fig. 9 MR-TADF emitters as the final dopant. (a) Molecular structures of MR-TADF emitters. (b) A schematic diagram of the hyperfluorescent energy transfer mechanism for MR-TADF as the final dopant. (c) Electroluminescence spectra of DBNO-based green HF-OLEDs. Reproduced with permission from ref. 139, John Wiley and Sons, 2022. (d) Electroluminescence spectra of TADF and HF devices based on green and red MR-TADF molecules with a DiKTa acceptor core. Reproduced with permission from ref. 140, John Wiley and Sons, 2022. (e) Electroluminescence spectra of solution-processed blue HF devices based on t-BuCz-DABNA. Reproduced with permission from ref. 141, John Wiley and Sons, 2023. | |
Regardless of these benefits, MR-TADF emitters typically suffer from severe efficiency roll-off at high current density as the RISC rates of MR-TADF compounds are often lower due to the long triplet lifetime, which leads to increased roll-off.142 This limitation of MR-TADF materials can be overcome by utilizing them as final dopants in HF systems (Fig. 9b). In combination with an efficient TADF sensitizer, HF-OLEDs based on narrowband MR-TADF final dopants represent a promising approach for practical applications in commercial displays.
Kondo et al. reported the efficient organo-boron-based TADF emitter v-DABNA (ΔEST = 0.017 eV) featuring rigid benzene rings that are connected by boron and nitrogen atoms.143 The device exhibited the multiple resonance effect of boron and nitrogen atoms, which gave rise to an extremely sharp blue emission at 468 nm with a FWHM of 18 nm and a maximum EQE of 34.4%. These advantages of v-DABNA look promising and can be implemented to expand its properties in fabricating high-efficiency HF-OLEDs. Chan et al. developed a strategy to obtain high-performance deep-blue OLEDs by efficiently transferring exciton energy from the TADF sensitizer TPh2Cz2DPhCzBN (HDT-1) to the singlet state of narrow emitter v-DABNA.144 The device showed a maximum EQE of 27% and retained an EQE of 20% even at a high luminance of 1000 cd m−2. Furthermore, to boost the performance of the device, a two-unit stacked tandem HF-OLED was designed, achieving a maximum EQE of 41% and 32% at 1000 cd m−2 along with deep-blue and narrow emission. Mamada et al. also utilized the benefits of v-DABNA as a final dopant after carefully screening different D–A structure-based TADF sensitizers with benzonitrile and carbazole rings.145 The blue HF-OLED exhibited a maximum EQE of 22.4% with a reduced roll-off of 17.8% at 1000 cd m−2. Very recently, Lee et al. demonstrated a quadrupolar D–A–D type TADF sensitizer, DBA-DTMCz, which exhibited an outstanding EQEmax of 43.9% with the v-DABNA emitter.146 The device maintained its high EQE of 37.5% even at 1000 cd m−2, indicating low-efficiency roll-off due to suppressed DET. Han et al. reported a pure blue HF-OLED using a derivative of the DABNA family, a fused B–N emitter, tDABNA, which showed a high EQE of 31.4%, with DMAC-DPS as a sensitizer.147 Mubarok et al. proposed a bulky, sterically shielded triptycene-fused deep-blue MR-TADF emitter, Tp-DABNA, where the rigid units can suppress the DET process and help in improving the device performance while maintaining narrow emission (26 nm).148 The HF-OLED based on Tp-DABNA and sensitizer TDBA-SAF demonstrated an EQE of 28.7%.
Another MR-TADF emitter, α-3BNMes, based on a B–N heptacene core with CIEy <0.1 was utilized as the final dopant, achieving a deep-blue HF-OLED with an EQE of 15%.149 The color coordinates reported were one of the lowest for deep-blue HF-OLEDs. Cai et al. reported a B–O–N-embedded MR-TADF emitter, DBNO, which showed narrowband green emission (Fig. 9c) and demonstrated high horizontal molecular orientation (96%).139 The DBNO-based OLED showed an EQE above 30% but experienced serious roll-off at high brightness. However, the DBNO-based HF-OLED obtained a high EQEmax of 37.1%, retaining up to 20.6% at 1000 cd m−2 with a FWHM of 27 nm. Hu et al. proposed a green MR-TADF emitter, BN-STO, decorated with peripheral heavy-atom selenium integration showing a high EQE of 40.1% and high color purity (FWHM = 29 nm, CIEy = 0.70).150 Using the sky-blue sensitizer, 5TBuCzBN, the maximum EQE of the HF-OLED obtained with BN-STO is 39.8% while exhibiting reduced efficiency roll-off, with an EQE of 34.0% at 1000 cd m−2 and 23.4% at 5000 cd m−2. Furthermore, Wu et al. demonstrated green and red MR-TADF molecules with a DiKTa acceptor core showing excellent EL performance (Fig. 9d).140 The maximum EQE obtained for the green HF-OLED was 30%, and for the red HF-OLED was 17.9%, also featuring reduced roll-off at high luminance. In a recent report, Stavrou et al. described the impact of the molecular structure of the sensitizer on FRET efficiency to obtain efficient HF-OLEDs.151 The work utilized a contrasting green sensitizer, ACRSA, to assist the blue MR-TADF emitter v-DABNA and obtained a maximum EQE of 28.5%, while a pure ACRSA-based TADF OLED showed a low EQE of 11.0% with high roll-off due to longer exciton lifetime. The work suggested a sensitization process where long-excited state lifetime TADF emitters could facilitate efficient FRET while maintaining balanced rate constants to ensure device stability.
Currently, achieving efficient solution-processed HF devices remains a significant challenge. For instance, Zhang et al. developed the MR-TADF emitter t-BuCz-DABNA, which has a low-lying HOMO level. Here, the peripheral carbazole unit in the emitter suppressed molecular aggregation and improved solubility. The emitter was utilized to fabricate solution-processed HF-OLEDs (sensitizer: 5CzTRZ) (Fig. 9e) that achieved a maximum EQE of 29.2% and 26.0% at 1000 cd m−2, which is one of the best results reported for solution-processed HF-OLEDs.141 The performances of HF-OLEDs using MR-TADF emitters as final dopants are presented in Table 3.
Table 3 Hyperfluorescent OLEDs with MR-TADF emitters as final dopants
Final dopant |
Sensitizer |
Host |
EL (nm) |
FWHM (nm) |
EQEmax/1000 cd m−2 (%) |
PEmax/1000 cd m−2 (lm W−1) |
CIE |
Ref. |
v-DABNA |
TPh2Cz2DPhCzBN (HDT-1) |
mCBP |
470 |
18 |
27/20 |
41/16 |
(0.15, 0.20) |
144
|
v-DABNA |
4PhCz2BN |
mCBP |
470 |
18 |
22.4/17.8 |
25/9.4 |
(0.13, 0.15) |
145
|
v-DABNA |
DBA-DTMCz |
DBFPO |
473 |
21 |
43.9/37.5 |
— |
(0.12, 0.16) |
146
|
t-DABNA |
DMAC-DPS |
DPEPO |
|
31 |
31.4/19.8 |
— |
(0.13, 0.15) |
147
|
Tp-DABNA |
TDBA-SAF |
DPEPO |
462 |
29 |
28.7/— |
29.8/- |
(0.14, 0.13) |
148
|
α-3BNMes |
DtBuAc-DBT |
DPEPO |
442 |
49 |
15/— |
— |
(0.15, 0.10) |
149
|
DBNO |
5TCzBN |
PhCzBCz |
504 |
27 |
37.1/20.6 |
105.6/— |
(0.14, 0.53) |
139
|
BN-STO |
5TBuCzBN |
DMIC-TRZ |
511 |
32 |
39.8/34.0 |
138.4/— |
(0.15, 0.66) |
150
|
3TPA-DiKTa |
4CzIPN |
mCP |
556 |
70 |
30.0/20.0 |
111/— |
(0.42, 0.55) |
140
|
3DPA-DikTa |
4CzIPN |
mCP |
615 |
61 |
17.9/6.0 |
37/— |
(0.58, 0.39) |
140
|
v-DABNA |
ACRSA |
mCBPCN |
473 |
19 |
28.5/18.6 |
36/12 |
(0.13, 0.17) |
151
|
v-DABNA |
PPCzTrz- |
oCBP:CNmCBPCN |
473 |
24 |
33.0/25.2 |
— |
(0.13, 0.20) |
152
|
v-DABNA |
PCzTrz |
oCBP:CNmCBPCN |
473 |
29 |
33.5/23.8 |
— |
(0.12, 0.18) |
152
|
![[thin space (1/6-em)]](https://www.rsc.org/images/entities/char_2009.gif) |
Solution-processed HF-OLEDs
|
t-BuCz-DABNA |
5CzTRZ |
mCP |
472 |
16.6 |
29.2/26.0 |
33.9/19.0 |
(0.13, 0.18) |
141
|
4.3 Exciplex-sensitized HF-OLEDs
Another approach for harvesting both singlet and triplet excitons in HF-OLEDs is the use of exciplex-based co-host systems (Fig. 10a) with subsequent exciton transfer via FRET to the fluorescent emitter (Fig. 10b). Exciplexes form between two dissimilar molecules possessing different energy levels by resonance interaction or charge transfer. Such a co-host system containing donor- and acceptor-type molecules (Fig. 10c) can outperform traditional hosts in terms of operating voltage, efficiency roll-off and device lifetime.155–157 Exciplex hosts can exhibit TADF characteristics due to their inherent donor–acceptor-type structure, which reduces the exchange energy and hence leads to small ΔEST. However, the participating materials need to be selected carefully in order to ensure that the triplet levels of the two individual host molecules are higher than the triplet energy of the exciplex.156 Moreover, for exciplex to show TADF features, the HOMO and LUMO offsets between the donor and acceptor materials should be sufficiently large to ensure enough charge accumulation at the donor–acceptor interface.157,158
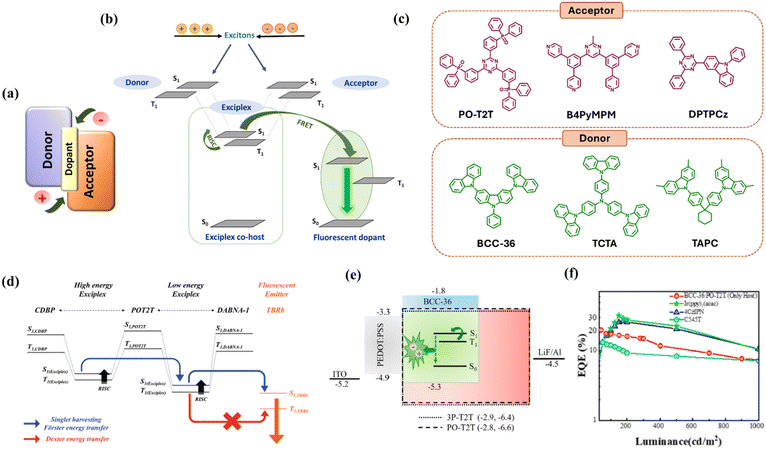 |
| Fig. 10 Exciplex co-host system. (a) A schematic diagram of an exciplex co-host system forming at the interface and a fluorescent dopant. (b) A schematic representation of the energy transfer from an exciplex co-host to a fluorescent dopant. (c) Molecular structures of donor and acceptor materials used to form exciplex hosts. (d) The cascade singlet harvesting mechanism using a high-energy exciplex and a low-energy exciplex to sensitize a fluorescent emitter. Reproduced with permission from ref. 153, John Wiley and Sons, 2020. (e and f) The device structure of an exciplex OLED and EQE vs. luminance plots of the exciplex device and sensitized OLEDs. Reproduced with permission from ref. 154, Elsevier, 2022. | |
Liu et al. demonstrated an exciplex co-host system with the TADF effect based on the blend TAPC:DPTPCz, where TAPC was the donor, and DPTPCz was the acceptor material. They used this co-host to sensitize the fluorescent emitter C545T, achieving an EQE of 14.5%.159 Another TADF type exciplex host TCTA:B4PyMPM has been explored by Kim and co-workers which has close to 0 eV ΔEST, indicating a fast RISC process.160 This green-emissive exciplex efficiently transferred energy to the red fluorescent emitter DCJTB and realized an EQE of 10.6%. Similar results for DCJTB in HF-OLEDs have also been obtained using the exciplex hosts TCTA:3PT2T and tris-PCz:CNT2T.161,162 Nguyen et al. utilized a TADF-type electron acceptor with a triazine derivative, 3Cz-TRZ, which formed an exciplex with tris-PCz and showed an EQE of around 10%.163 The performance improved two-fold (EQE ∼19%) when it was used as a co-host to sensitize v-DABNA.
Liang et al. introduced a high triplet energy electron acceptor based on benzimidazole-triazine, PIM-TRZ, which formed exciplexes with the electron donor TAPC.164 The pure exciplex device exhibited a low turn-on voltage of 2.3 eV and obtained an EQE of 21.7%. Using TAPC:PIM-TRZ as the co-host for the fluorescent emitter C545T, the maximum EQE was 20.2%, with 18.4% of EQE being retained at 1000 cd m−2, showing significantly reduced roll-off. Li et al. fabricated OLEDs with an EQE of 12.9% based on a double sensitizer system which deployed an exciplex co-host, TCTA:B4PyMPM, and the TADF molecule 4CzIPN acting as an additional sensitizer for the red fluorescent emitter DCJTB.165 Further improvement can be achieved using a cascade energy transfer via two TADF-type exciplexes to selectively transfer singlet excitons to the fluorescent emitter. Following this strategy, Lee et al. used CDBP:PO-T2T as a high-energy exciplex and PO-T2T:t-DABNA as a low-energy exciplex to enable efficient FRET to the fluorescent emitter TBRb (Fig. 10d) and obtained an EQE of 19.9%.153 Wang et al. fabricated solution-processed red fluorescent OLEDs using a diluted exciplex host, TCTA:PO-T2T, to reduce non-radiative triplet exciton loss and obtained an EQEmax of 8.1% for DCJTB.166 Kesavan et al. reported a solution-processed OLED with an EQE above 25% for the TADF exciplex BCC-36:PO-T2T which has been used as a co-host to sensitize the TADF emitter 4CzIPN (Fig. 10e and f).154 To conclude, sensitization of singlet excitons via TADF-type exciplex co-hosts offers an efficient alternative to conventional TADF assistant dopants in realizing efficient HF-OLEDs. However, achieving exciplex co-hosts for blue fluorescent dopants has been challenging as they require an extremely large energy gap in order to sensitize the blue emitters. The device performances of exciplex-sensitized HF-OLEDs are summarized in Table 4.
Table 4 Exciplex sensitized hyperfluorescent OLEDs
Final dopant |
Exciplex co-host |
EQEmax/1000 cd m−2 (%) |
PEmax/1000 cd m−2 (lm W−1) |
CIE |
Ref. |
(0.2%) C545T |
TAPC:DPTPCz |
14.5 |
46.1 |
(0.24, 0.55) |
159
|
(0.5%) DCJTB |
TCTA:B4PYMPM |
10.6 |
26.8 |
— |
160
|
(1.0%) DCJTB |
TCTA:3P-T2T |
10.15/10.03 |
21.5/18.9 |
— |
161
|
(1.0%) DCJTB |
Tris-PCz:CN-T2T |
9.7/9.1 |
23.3 |
(0.59, 0.40) |
162
|
(1.0%) v-DABNA |
Tri-PCz:3Cz-TRZ |
19/18 |
— |
— |
163
|
(0.6%) C545T |
TAPC:PIM-TRZ |
20.2/18.4 |
86.4/51.1 |
(0.29, 0.64) |
164
|
(0.5%) DCJTB |
TCTA:B4PYMPM:4CzIPN |
12.9/10.1 |
24.0/12.7 |
(0.58, 0.41) |
165
|
(0.5%) TBRb |
CDBP:POT2T:DABNA-1 |
18.7/18.0 |
46.7/36.0 |
(0.41, 0.56) |
153
|
(0.5%) TBRb |
CDBP:POT2T:t-DABNA |
19.9 |
— |
(0.45, 0.53) |
153
|
![[thin space (1/6-em)]](https://www.rsc.org/images/entities/char_2009.gif) |
Solution-processed HF-OLEDs
|
(0.5%) DCJTB |
TCTA:PO-T2T |
8.1 |
11.1 |
(0.52, 0.46) |
166
|
(1.0%) C545T |
BCC36:POT2T |
12.5/7.1 |
25/14.6 |
(0.24, 0.57) |
154
|
(7.5%) 4CzIPN |
BCC36:POT2T |
26.5/10.6 |
39.1/27.2 |
(0.26, 0.56) |
154
|
5. Status of operational lifetime
The long operational lifetime of OLEDs is one of the key requirements for commercial applications. Despite high EQE, the device lifetime for TADF-based OLEDs is low as compared to traditional fluorescent OLEDs167–169 due to the presence of long-lived excitons in triplet states, which lead to material degradation and short operational lifetime.170–172 Here, hyperfluorescent systems, which contain both TADF and fluorescent emitters, could offer prospects for realizing high efficiency and stability in OLEDs due to reduced excited state lifetime in the overall emission process and material stability of fluorescent dopants. For example, Furukawa et al. reported a yellow HF device in 2015 with 4CzIPN-Me as the TADF sensitizer and TBRb as the fluorescent emitter showing an operational lifetime LT50 of 3775 h at 1000 cd m−2, which was over two times longer than that of the conventional TADF-based device.74 However, in HF-OLEDs, the presence of triplet excitons on the TADF sensitizer results in accelerated degradation of the devices. These particularly long-lived excitons participate in various annihilation processes inside the device, such as singlet–triplet annihilation, triplet–triplet annihilation, and triplet–polaron annihilation, which accelerate the loss mechanism.173,174 Therefore, the management of these exciton loss processes is important to ensure a long lifetime in HF devices.
There are some factors which can be employed to improve the operational lifetime of HF-OLEDs. First, the TADF assistant dopant should possess a fast RISC rate, as well as a high bond dissociation energy (BDE). TADF molecules with low BDE hinder the stability of the device and lead to a shorter operational lifetime. Typically, the BDE of the TADF emitters should be higher than their exciton energy.175 In addition to stable TADF emitters, the host material and all charge-transporting layers in the multilayer structure must also exhibit high BDE to extend the device's overall durability.176 Second, the diffusion of triplet excitons from TADF to the final dopant should be minimized, which is possible by introducing bulky unit groups in the TADF sensitizer or in the final dopant. Third, the device structure needs to be optimized for a longer lifetime since OLEDs consist of a multilayer structure, in which each layer contributes to the overall performance and stability of the device. Here, appropriate charge transporting and exciton blocking layers need to be selected in combination with the respective emissive layer. Stacking of devices can improve the lifetime as reported by Chan et al., who showed that a two-unit stacked tandem HF-OLED with v-DABNA can increase the operational lifetime from an LT95 of 11 h at 1000 cd m−2 for the single unit to 18 h in the double unit (Fig. 11a and b).144 Nakamura et al. reported green HF-OLEDs with a BODIPY emitter, tPhBODIPY, as the final dopant in which they achieved an enhancement in the device lifetime from an LT50 of 8283 h to 14
443 h at 1000 cd m−2 by using three stacked hole transporting layers (HTLs) (Fig. 11c).67 Since charge accumulation at interfaces is detrimental to device stability,177,178 the improved energy alignment in the triple HTL structure helped reduce hole trapping at the HTL/EML interface, resulting in an enhanced lifetime.
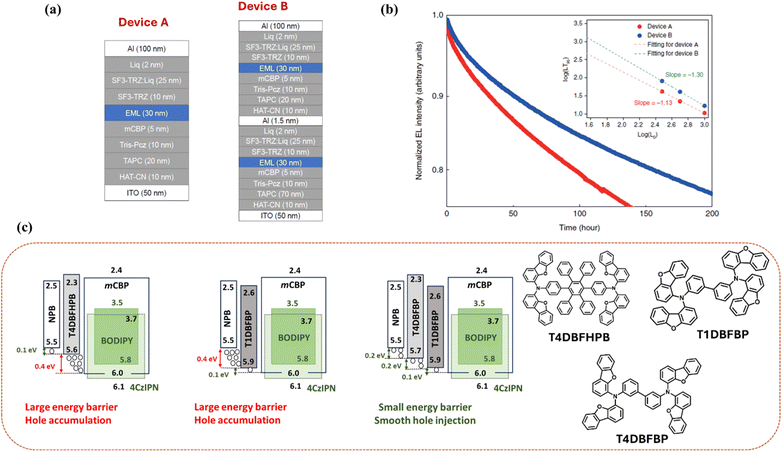 |
| Fig. 11 (a) Device structure of single-unit and two-unit stacked tandem hyperfluorescent OLEDs. (b) Electroluminescence intensity versus operation time at an initial luminance of 1000 cd m−2. Inset: LT95 as a function of L0. Reproduced with permission from ref. 144, Nature, 2021. (c) Schematic illustration of hole injection behaviour from the HTL to the EML using various HTLs, along with the molecular structures of the respective HTLs used. | |
Kim et al. reported a yellow TADF sensitizer with a strong bulky donor, 5,10-diphenyl-10,15-dihydro-5H-diindolo carbazole (TruX), having a distorted geometry from the linker plane. The HF device utilized TBRb as the final dopant and demonstrated a device lifetime LT90 of 1400 h at 1000 cd m−2.75 The extended lifetime in the device indicated efficient FRET due to the high RISC rate of the sensitizer, which reduced the long-delayed component and suppressed the triplet exciton population in the device. Lee et al. reported the bulky, high-RISC rate TADF sensitizer 2BT34CzINN to fabricate HF-OLEDs with DBP and achieved a device lifetime LT90 of 40 h at 3000 cd m−2.73 Shin et al. mentioned an operational lifetime LT90 of 1312 h at 3000 cd m−2 for a red HF-OLED with 12BTCzTPN as the TADF sensitizer and 4tBuMB as the final dopant.71 This is one of the longest lifetimes reported for red HF devices. Achieving high EQE and long lifetime simultaneously in blue OLEDs is challenging. Jeon et al. reported a high EQE of over 30% and a long lifetime LT50 of over 5000 h (at 100 cd m−2) for a deep-blue (CIEy <0.10) HF-OLED through triplet exciton recycling using PPCzTrz as the sensitizer with v-DABNA as the final dopant.152 Until now, yellow HF-OLEDs have already been commercialized in small display applications such as passive matrix OLED displays, while red and green devices have started showing good results and may be implemented in commercial products shortly.179,180 However, blue HF devices, still require significant optimization to obtain both high efficiency and stability to enter the commercial market.
6. Summary and outlook
In summary, hyperfluorescent OLEDs have shown great potential in achieving high efficiency, good color saturation and a long device operational lifetime, which paves the way for their implementation in practical applications. Efficient emitters are essential for high-performance devices, together with finely tuned molecular distances and energy levels of the TADF sensitizer and final emitter in order to improve device efficiency and roll-off characteristics. FRET between the TADF sensitizer and the final dopant is the primary energy transfer process in the HF-OLEDs; hence, sufficient spectral overlap between the emitters is important to increase the energy transfer efficiency. An efficient FRET process plays a vital role in enhancing the device efficiency for blue, green and red devices, whereas white HF-OLEDs require a well-balanced emission from both TADF and final emitters. Therefore, appropriate material selection and combination are crucial for achieving better device performance. HF-OLEDs are recognized as next-generation efficient devices with pure organic molecules, yet certain challenges must be addressed to fully harness their potential for commercialization.
One of the key challenges in HF-OLEDs is to control the loss mechanisms associated with the energy transfer process. Direct charge trapping on the fluorescent emitter can be reduced by minimizing the doping concentration in the emissive layer, thus preventing the formation of non-radiative triplet excitons. Another energy loss process arises due to DET of triplet excitons from the TADF sensitizer to the fluorescent dopant. DET can be strategically managed by increasing the intermolecular distance between the sensitizer and final dopant via inserting electronically inert bulky substitutions in the emitters. Using MR-TADF molecules as final dopants can be advantageous as these can also employ DET for maximum exciton utilization. Furthermore, MR-TADF emitters offer a narrowband spectrum and provide improved EQE roll-off properties in HF-OLEDs. Another approach for realizing efficient devices is using exciplex-sensitized co-host systems. For blue OLEDs, however, only very few exciplex co-hosts are available due to the very high triplet energy gap requirement.
The most critical issue of HF-OLEDs that currently limits their commercialization is the device operational lifetime, particularly for blue HF devices. Although there are several reports on extended operational lifetime, HF-OLEDs still exhibit shorter lifespans as compared to conventional fluorescent devices. The degradation of HF-OLEDs is mainly attributed to the presence of long-lived triplet excitons in the emissive layer, which take part in various annihilation processes. These processes are lethal to the organic layers in the device and, therefore, affect device stability. Hence, the design of the TADF sensitizer is important, emphasizing high BDE and providing a short-delayed fluorescence excited state lifetime while maintaining a stable triplet excited state density for high-efficiency HF devices. With such a robust molecular design and advanced device engineering, HF-OLEDs hold the potential to mitigate the remaining challenges and significantly improve both efficiency and operational lifetime.
Data availability
It is perspective and data availability is not applicable for this submission.
Author contributions
The project was conceptualized by P. R. and U. D. The manuscript was written by U. D. and G. P. N. contributed in the formatting manuscript under supervision C. M. and P. R.
Conflicts of interest
There are no conflicts to declare.
Acknowledgements
The authors thank IISc and the Science & Engineering Research Board (SERB), India, SERB-Power Grant (SPG) (Grant No. SPG/2020/000107) for financial support and The Indo-German Science & Technology Centre (IGSTC) for the WISER award (IGSTC/WISER 2022/RG/52/2022-23) and financial support. The authors thank the German BMBF (13XP5137) and the Deutsche Forschungsgemeinschaft (DFG, German research foundation) through GRK 2767 (451785257) for funding. This work was co-financed by tax revenue based on the budget approved by the Saxon State Parliament.
References
- C. W. Tang and S. A. VanSlyke, Appl. Phys. Lett., 1987, 51, 913–915 CrossRef CAS.
- Y. Sun, N. C. Giebink, H. Kanno, B. Ma, M. E. Thompson and S. R. Forrest, Nature, 2006, 440, 908–912 CrossRef CAS PubMed.
- S. Reineke, F. Lindner, G. Schwartz, N. Seidler, K. Walzer, B. Lüssem and K. Leo, Nature, 2009, 459, 234–238 CrossRef CAS PubMed.
- X. Cai and S.-J. Su, Adv. Funct. Mater., 2018, 28, 1802558 CrossRef.
-
M. Pope and C. E. Swenberg, Electronic Processes in Organic Crystals and Polymers, Oxford University PressNew, York, NY, 1999 Search PubMed.
- A. R. Brown, K. Pichler, N. C. Greenham, D. D. C. Bradley, R. H. Friend and A. B. Holmes, Chem. Phys. Lett., 1993, 210, 61–66 CrossRef CAS.
- R. H. Friend, R. W. Gymer, A. B. Holmes, J. H. Burroughes, R. N. Marks, C. Taliani, D. D. C. Bradley, D. A. Dos Santos, J. L. Brédas, M. Lögdlund and W. R. Salaneck, Nature, 1999, 397, 121–128 CrossRef CAS.
- A. R. Brown, D. D. C. Bradley, J. H. Burroughes, R. H. Friend, N. C. Greenham, P. L. Burn, A. B. Holmes and A. Kraft, Appl. Phys. Lett., 1992, 61, 2793–2795 CrossRef CAS.
- H.-H. Chou, Y.-H. Chen, H.-P. Hsu, W.-H. Chang, Y.-H. Chen and C.-H. Cheng, Adv. Mater., 2012, 24, 5867–5871 CrossRef CAS PubMed.
- H. Jung, S. Kang, H. Lee, Y.-J. Yu, J. H. Jeong, J. Song, Y. Jeon and J. Park, ACS Appl. Mater. Interfaces, 2018, 10, 30022–30028 CrossRef CAS PubMed.
- Y. Zhang and S. R. Forrest, Phys. Rev. Lett., 2012, 108, 267404 CrossRef.
- T. Tsutsui, E. Aminaka, C. P. Lin and D.-U. Kim, Philos. Trans. R. Soc. London, Ser. A, 1997, 355, 801–814 CrossRef CAS.
- S. Nowy, B. C. Krummacher, J. Frischeisen, N. A. Reinke and W. Brütting, J. Appl. Phys., 2008, 104, 123109 CrossRef.
- M. Furno, R. Meerheim, S. Hofmann, B. Lüssem and K. Leo, Phys. Rev. B: Condens. Matter Mater. Phys., 2012, 85, 115205 CrossRef.
- B. Sim, C.-K. Moon, K.-H. Kim and J.-J. Kim, ACS Appl. Mater. Interfaces, 2016, 8, 33010–33018 CrossRef CAS.
- M. A. Baldo, D. F. O'Brien, Y. You, A. Shoustikov, S. Sibley, M. E. Thompson and S. R. Forrest, Nature, 1998, 395, 151–154 CrossRef CAS.
- S. Reineke, T. C. Rosenow, B. Lüssem and K. Leo, Adv. Mater., 2010, 22, 3189–3193 CrossRef CAS.
- H. Sasabe and J. Kido, Eur. J. Org. Chem., 2013, 2013, 7653–7663 CrossRef CAS.
- C. Fan, L. Zhu, T. Liu, B. Jiang, D. Ma, J. Qin and C. Yang, Angew. Chem., 2014, 126, 2179–2183 CrossRef.
- M. A. Baldo, M. E. Thompson and S. R. Forrest, Nature, 2000, 403, 750–753 CrossRef CAS PubMed.
- B. Minaev, G. Baryshnikov and H. Agren, Phys. Chem. Chem. Phys., 2014, 16, 1719–1758 RSC.
- G. Schwartz, S. Reineke, T. C. Rosenow, K. Walzer and K. Leo, Adv. Funct. Mater., 2009, 19, 1319–1333 CrossRef CAS.
- J. Lee, H.-F. Chen, T. Batagoda, C. Coburn, P. I. Djurovich, M. E. Thompson and S. R. Forrest, Nat. Mater., 2016, 15, 92–98 CrossRef CAS PubMed.
- H. Uoyama, K. Goushi, K. Shizu, H. Nomura and C. Adachi, Nature, 2012, 492, 234–238 CrossRef CAS PubMed.
- H. Kaji, H. Suzuki, T. Fukushima, K. Shizu, K. Suzuki, S. Kubo, T. Komino, H. Oiwa, F. Suzuki, A. Wakamiya, Y. Murata and C. Adachi, Nat. Commun., 2015, 6, 8476 CrossRef CAS PubMed.
- Z. Yang, Z. Mao, Z. Xie, Y. Zhang, S. Liu, J. Zhao, J. Xu, Z. Chi and M. P. Aldred, Chem. Soc. Rev., 2017, 46, 915–1016 RSC.
- M. Y. Wong and E. Zysman-Colman, Adv. Mater., 2017, 29, 1605444 CrossRef.
- T. J. Penfold, F. B. Dias and A. P. Monkman, Chem. Commun., 2018, 54, 3926–3935 RSC.
- P. Jiang, J. Miao, X. Cao, H. Xia, K. Pan, T. Hua, X. Lv, Z. Huang, Y. Zou and C. Yang, Adv. Mater., 2022, 34, 2106954 CrossRef CAS.
- W. Yang, J. Miao, F. Hu, Y. Zou, C. Zhong, S. Gong and C. Yang, Adv. Funct. Mater., 2023, 33, 2213056 CrossRef CAS.
- F. B. Dias, T. J. Penfold and A. P. Monkman, Methods Appl. Fluoresc., 2017, 5, 012001 CrossRef.
- Q. Zhang, J. Li, K. Shizu, S. Huang, S. Hirata, H. Miyazaki and C. Adachi, J. Am. Chem. Soc., 2012, 134, 14706–14709 CrossRef CAS.
- F.-M. Xie, J.-X. Zhou, Y.-Q. Li and J.-X. Tang, J. Mater. Chem. C, 2020, 8, 9476–9494 RSC.
- C. H. Chen, J. Shi and C. W. Tang, Macromol. Symp., 1998, 125, 1–48 CrossRef CAS.
- M. Xie, M. Sun, S. Xue and W. Yang, Dyes Pigm., 2023, 208, 110799 CrossRef.
- H. Nakanotani, T. Higuchi, T. Furukawa, K. Masui, K. Morimoto, M. Numata, H. Tanaka, Y. Sagara, T. Yasuda and C. Adachi, Nat. Commun., 2014, 5, 4016 CrossRef CAS PubMed.
- Y. Giret, J. Eng, T. Pope and T. Penfold, J. Mater. Chem. C, 2021, 9, 1362–1369 RSC.
- J. Adachi, H. Kakizoe, P. K. D. Tsang and A. Endo, SID Int. Symp. Dig. Tech. Pap., 2019, 50, 95–98 CrossRef CAS.
- D. Zhang, L. Duan, C. Li, Y. Li, H. Li, D. Zhang and Y. Qiu, Adv. Mater., 2014, 26, 5050–5055 CrossRef CAS PubMed.
- N. Haase, A. Danos, C. Pflumm, P. Stachelek, W. Brütting and A. P. Monkman, Mater. Horiz., 2021, 8, 1805–1815 RSC.
- X. Wu, X. Peng, L. Chen, B. Z. Tang and Z. Zhao, ACS Mater. Lett., 2023, 5, 664–672 CrossRef CAS.
- Z. Chen, Q. Gu, M. Li, W. Qiu, Y. Jiao, X. Peng, W. Xie, D. Liu, K. Liu, Z. Yang and S.-J. Su, Adv. Opt. Mater., 2024, 12, 2302503 CrossRef CAS.
- J. Lv, Y. Huo, S. Xiao, Z. Zhao, L. Peng, Y. Liu, Z. Ren, D. Ma, S. Ying and S. Yan, Mater. Chem. Front., 2023, 7, 85–95 RSC.
- J. Jayabharathi, S. Thilagavathy, V. Thanikachalam and J. Anudeebhana, J. Mater. Chem. C, 2022, 10, 4342–4354 RSC.
- A. Endo and P. K. D. Tang, Dig. Tech. Pap. – Soc. Inf. Disp. Int. Symp., 2019, 50, 360–362 CrossRef.
- T. Baumann, M. Budzynski and C. Kasparek, Dig. Tech. Pap. – Soc. Inf. Disp. Int. Symp., 2019, 50, 466–469 CrossRef.
- S. Y. Byeon, D. R. Lee, K. S. Yook and J. Y. Lee, Adv. Mater., 2019, 31, 1–15 CrossRef.
- T. Főrster, Discuss. Faraday Soc., 1959, 27, 7–17 RSC.
- H. Wang, B. Yue, Z. Xie, B. Gao, Y. Xu, L. Liu, H. Sun and Y. Ma, Phys. Chem. Chem. Phys., 2013, 15, 3527 RSC.
- N. Aizawa, S. Shikita and T. Yasuda, Chem. Mater., 2017, 29, 7014–7022 CrossRef CAS.
- K. Gao, K. Liu, X. L. Li, X. Cai, D. Chen, Z. Xu, Z. He, B. Li, Z. Qiao, D. Chen, Y. Cao and S. J. Su, J. Mater. Chem. C, 2017, 5, 10406–10416 RSC.
- W. Song, I. Lee and J. Y. Lee, Adv. Mater., 2015, 27, 4358–4363 CrossRef CAS PubMed.
- Y. J. Kang, J. H. Yun and J. Y. Lee, Org. Electron., 2020, 78, 105604 CrossRef CAS.
- Y. Wada, H. Nakagawa, S. Matsumoto, Y. Wakisaka and H. Kaji, Nat. Photonics, 2020, 14, 643–649 CrossRef CAS.
- L.-S. Cui, A. J. Gillett, S.-F. Zhang, H. Ye, Y. Liu, X.-K. Chen, Z.-S. Lin, E. W. Evans, W. K. Myers, T. K. Ronson, H. Nakanotani, S. Reineke, J.-L. Bredas, C. Adachi and R. H. Friend, Nat. Photonics, 2020, 14, 636–642 CrossRef CAS.
- D. H. Ahn, J. H. Jeong, J. Song, J. Y. Lee and J. H. Kwon, ACS Appl. Mater. Interfaces, 2018, 10, 10246–10253 CrossRef CAS.
- Y. Wu, X. Liu, J. Liu, G. Yang, Y. Deng, Z. Bin and J. You, J. Am. Chem. Soc., 2024, 146, 15977–15985 CrossRef CAS.
- F. Wang, L. Zhang, W. Han, Z. Bin and J. You, Angew. Chem., Int. Ed., 2022, 61, e202205380 CrossRef CAS PubMed.
- J. H. Yun, K. H. Lee and J. Y. Lee, Dyes Pigm., 2020, 181, 108549 CrossRef CAS.
- J. S. Jang, S. H. Han, H. W. Choi, K. S. Yook and J. Y. Lee, Org. Electron., 2018, 59, 236–242 CrossRef CAS.
- J. S. Jang, S. H. Han and J. Lee, Adv. Photonics Res., 2021, 2, 2000109 CrossRef CAS.
- B. Sk, V. Thangaraji, N. Yadav, G. P. Nanda, S. Das, P. Gandeepan, E. Zysman-Colman and P. Rajamalli, J. Mater. Chem. C, 2021, 9, 15583–15590 RSC.
- M. Li, J. Wang, Y. Dai, Y. Zhang, L. Chen, L. Jin, Y. Tao, R. Chen and W. Huang, J. Phys. Chem. C, 2020, 124, 1836–1843 CrossRef CAS.
- D. Zhang, X. Song, M. Cai and L. Duan, Adv. Mater., 2018, 30, 1705250 CrossRef.
- U. Deori, N. Yadav, G. P. Nanda, K. L. Kumawat and P. Rajamalli, ACS Appl. Electron. Mater., 2023, 5, 4959–4967 CrossRef CAS.
- X. Song, D. Zhang, Y. Zhang, Y. Lu and L. Duan, Adv. Opt. Mater., 2020, 8, 1–10 Search PubMed.
- T. Nakamura, H. Sasabe, S. Abe, K. Kumada, R. Sugiyama, T. Hanayama and J. Kido, Mol. Syst. Des. Eng., 2023, 8, 866–873 RSC.
- Z. Li, X. Hu, G. Liu, L. Tian, H. Gao, X. Dong, T. Gao, M. Cao, C. S. Lee, P. Wang and Y. Wang, J. Phys. Chem. C, 2021, 125, 1980–1989 CrossRef CAS.
- Z. Li, X. Dong, G. Liu, L. Tian, X. Hu, T. Gao, H. Gao, Y. Qin, X. Gu, C. S. Lee, P. Wang, Y. Wang and Y. Liu, Energy Fuels, 2021, 35, 19104–19111 CrossRef CAS.
- Y. H. Jung, D. Karthik, H. Lee, J. H. Maeng, K. J. Yang, S. Hwang and J. H. Kwon, ACS Appl. Mater. Interfaces, 2021, 13, 17882–17891 CrossRef CAS PubMed.
- D. J. Shin, S. J. Hwang, J. Lim, C. Y. Jeon, J. Y. Lee and J. H. Kwon, Chem. Eng. J., 2022, 446, 137181 CrossRef CAS.
- W. Xie, X. Peng, M. Li, W. Qiu, W. Li, Q. Gu, Y. Jiao, Z. Chen, Y. Gan, K. kun Liu and S. J. Su, Adv. Opt. Mater., 2022, 10, 1–8 Search PubMed.
- J. Lee, U. Jo and J. Y. Lee, ACS Appl. Mater. Interfaces, 2023, 15, 21261–21269 CrossRef CAS.
- T. Furukawa, H. Nakanotani, M. Inoue and C. Adachi, Sci. Rep., 2015, 5, 8429 CrossRef CAS PubMed.
- J. H. Kim, K. H. Lee and J. Y. Lee, J. Mater. Chem. C, 2020, 8, 5265–5272 RSC.
- S. K. Jeon, H. J. Park and J. Y. Lee, ACS Appl. Mater. Interfaces, 2018, 10, 5700–5705 CrossRef CAS.
- D. Chen, X. Cai, X. Li, Z. He and C. Cai, J. Mater. Chem. C, 2017, 5, 5223–5231 RSC.
- G. Zhao, R. Zhou, G. Zhang, H. Chen, D. Ma, W. Tian, W. Jiang and Y. Sun, J. Mater. Chem. C, 2022, 10, 5230–5239 RSC.
- N. R. Wallwork, M. Mamada, A. Shukla, S. K. M. McGregor, C. Adachi, E. B. Namdas and S. C. Lo, J. Mater. Chem. C, 2021, 10, 4767–4774 RSC.
- Q. Zhang, B. Li, S. Huang, H. Nomura, H. Tanaka and C. Adachi, Nat. Photonics, 2014, 8, 326–332 CrossRef CAS.
- J.-A. Seo, Y. Im, S. H. Han, C. W. Lee and J. Y. Lee, ACS Appl. Mater. Interfaces, 2017, 9, 37864–37872 CrossRef CAS PubMed.
- D. Barman, K. Narang, R. Gogoi, D. Barman and P. K. Iyer, J. Mater. Chem. C, 2022, 10, 8536–8583 RSC.
- S. M. Leung, T. Chiu, C. Lin, J. Lee and M. Leung, J. Mater. Chem. C, 2019, 7, 5874–5888 RSC.
- I. H. Lee, W. Song, J. Y. Lee and S. H. Hwang, J. Mater. Chem. C, 2015, 3, 8834–8838 RSC.
- N. Boens, V. Leen and W. Dehaen, Chem. Soc. Rev., 2012, 41, 1130–1172 RSC.
- J. Bañuelos, V. Martín, C. F. A. Gómez-Durán, I. J. A. Córdoba, E. Peña-Cabrera, I. García-Moreno, Á. Costela, M. E. Pérez-Ojeda, T. Arbeloa and Í. L. Arbeloa, Chem.–Eur. J., 2011, 17, 7261–7270 CrossRef.
- A. Loudet and K. Burgess, Chem. Rev., 2007, 107, 4891–4932 CrossRef CAS PubMed.
- M. Chapran, E. Angioni, N. J. Findlay, B. Breig, V. Cherpak, P. Stakhira, T. Tuttle, D. Volyniuk, J. V. Grazulevicius, Y. A. Nastishin, O. D. Lavrentovich and P. J. Skabara, ACS Appl. Mater. Interfaces, 2017, 9, 4750–4757 CrossRef CAS PubMed.
- J. V. Caspar, B. P. Sullivan, E. M. Kober and T. J. Meyer, Chem. Phys. Lett., 1982, 91, 91–95 CrossRef CAS.
- W. Zeng, T. Zhou, W. Ning, C. Zhong, J. He, S. Gong, G. Xie and C. Yang, Adv. Mater., 2019, 31, 1901404 CrossRef.
- V. H. K. Fell, N. J. Findlay, B. Breig, C. Forbes, A. R. Inigo, J. Cameron, A. L. Kanibolotsky and P. J. Skabara, J. Mater. Chem. C, 2019, 7, 3934–3944 RSC.
- Q. Zhang, H. Kuwabara, W. J. Potscavage, S. Huang, Y. Hatae, T. Shibata and C. Adachi, J. Am. Chem. Soc., 2014, 136, 18070–18081 CrossRef CAS PubMed.
- W. Zeng, H.-Y. Lai, W.-K. Lee, M. Jiao, Y.-J. Shiu, C. Zhong, S. Gong, T. Zhou, G. Xie, M. Sarma, K.-T. Wong, C.-C. Wu and C. Yang, Adv. Mater., 2018, 30, 1704961 CrossRef PubMed.
- S. Das, S. Kundu, B. Sk, M. Sarkar and A. Patra, Org. Mater., 2021, 3, 477–487 CrossRef CAS.
- A. Shukla, N. R. Wallwork, X. Li, J. Sobus, V. T. N. Mai, S. K. M. McGregor, K. Chen, R. J. Lepage, E. H. Krenske, E. G. Moore, E. B. Namdas and S. Lo, Adv. Opt. Mater., 2020, 8, 1901350 CrossRef CAS.
- X.-L. Li, G. Xie, M. Liu, D. Chen, X. Cai, J. Peng, Y. Cao and S.-J. Su, Adv. Mater., 2016, 28, 4614–4619 CrossRef CAS PubMed.
- S. Kundu, B. Sk, P. Pallavi, A. Giri and A. Patra, Chem.–Eur. J., 2020, 26, 5557–5582 CrossRef CAS PubMed.
- D. Das, P. Gopikrishna, D. Barman, R. B. Yathirajula and P. K. Iyer, Nano Convergence, 2019, 6, 1–28 CrossRef CAS.
-
G. He, L. Zheng and H. Yan, in Proc. SPIE, 2010, vol. 7852, p. 78520A Search PubMed.
- K. T. Kamtekar, A. P. Monkman and M. R. Bryce, Adv. Mater., 2010, 22, 572–582 CrossRef CAS.
- T. Zhang, S.-J. He, D.-K. Wang, N. Jiang and Z.-H. Lu, Sci. Rep., 2016, 6, 20517 CrossRef CAS PubMed.
- N. Sun, Y. Zhao, F. Zhao, Y. Chen, D. Yang, J. Chen and D. Ma, Appl. Phys. Lett., 2014, 105, 13303 CrossRef.
- J.-H. Jou, S.-M. Shen, C.-R. Lin, Y.-S. Wang, Y.-C. Chou, S.-Z. Chen and Y.-C. Jou, Org. Electron., 2011, 12, 865–868 CrossRef CAS.
- G. Zhou, Q. Wang, C.-L. Ho, W.-Y. Wong, D. Ma and L. Wang, Chem. Commun., 2009, 3574–3576 RSC.
- B. Zhao, T. Zhang, W. Li, Z. Su, B. Chu, X. Yan, F. Jin, Y. Gao and H. Wu, Org. Electron., 2015, 23, 208–212 CrossRef CAS.
- W. Song, I. H. Lee, S. H. Hwang and J. Y. Lee, Org. Electron., 2015, 23, 138–143 CrossRef CAS.
- D. Zhang, M. Cai, Y. Zhang, D. Zhang and L. Duan, ACS Appl. Mater. Interfaces, 2015, 7, 28693–28700 CrossRef CAS PubMed.
- Z. Wu, L. Yu, X. Zhou, Q. Guo, J. Luo, X. Qiao, D. Yang, J. Chen, C. Yang and D. Ma, Adv. Opt. Mater., 2016, 4, 1067–1074 CrossRef CAS.
- F. M. Xie, S. J. Zou, Y. Li, L. Y. Lu, R. Yang, X. Y. Zeng, G. H. Zhang, J. Chen and J. X. Tang, ACS Appl. Mater. Interfaces, 2020, 12, 16736–16742 CrossRef CAS.
- T. Higuchi, H. Nakanotani and C. Adachi, Adv. Mater., 2015, 27, 2019–2023 CrossRef CAS PubMed.
- H. Liu, J. Chen, Y. Fu, Z. Zhao and B. Z. Tang, Adv. Funct. Mater., 2021, 31, 2103273 CrossRef CAS.
- H. Liu, Y. Fu, B. Z. Tang and Z. Zhao, Adv. Funct. Mater., 2023, 33, 2309770 CrossRef CAS.
- Z. Liu, B. Zhao, Y. Gao, H. Chen, B. Dong, Y. Xu, J. Li, H. Wang and W. Li, Opt. Mater., 2020, 110, 110510 CrossRef CAS.
- S.-J. Zou, F.-M. Xie, Y.-Q. Li, Y.-Z. Shi, Y. Shen, Z.-G. Ma, J.-D. Chen, H.-X. Wei, X.-H. Zhang and J.-X. Tang, Mater. Today Energy, 2021, 21, 100745 CrossRef CAS.
- Z. Wu, Q. Wang, L. Yu, J. Chen, X. Qiao, T. Ahamad, S. M. Alshehri, C. Yang and D. Ma, ACS Appl. Mater. Interfaces, 2016, 8, 28780–28788 CrossRef CAS PubMed.
- X. Tang, Y. Li, Y. K. Qu, C. C. Peng, A. Khan, Z. Q. Jiang and L. S. Liao, Adv. Funct. Mater., 2020, 30, 1–8 Search PubMed.
- Y. Chen, Q. Sun, Y. Dai, D. Yang, X. Qiao and D. Ma, J. Mater. Chem. C, 2020, 8, 13777–13785 RSC.
- Z. Wang, X. L. Li, Z. Ma, X. Cai, C. Cai and S. J. Su, Adv. Funct. Mater., 2018, 28, 1–9 Search PubMed.
- H. Liu, Y. Fu, B. Z. Tang and Z. Zhao, Nat. Commun., 2022, 13, 5154 CrossRef CAS.
- P. Wei, D. Zhang and L. Duan, Adv. Funct. Mater., 2020, 30, 1907083 CrossRef CAS.
- S. Gottardi, M. Barbry, R. Coehoorn and H. van Eersel, Appl. Phys. Lett., 2019, 114, 73301 CrossRef.
- H. Abroshan, V. Coropceanu and J.-L. Brédas, ACS Mater. Lett., 2020, 2, 1412–1418 CrossRef CAS.
- D. J. Shin, S. C. Kim and J. Y. Lee, J. Mater. Chem. C, 2022, 10, 4821–4830 RSC.
- D. L. Dexter, J. Chem. Phys., 2004, 21, 836–850 CrossRef.
- Y. Zhang and S. R. Forrest, Chem. Phys. Lett., 2013, 590, 106–110 CrossRef CAS.
- S. H. Han and J. Y. Lee, J. Mater. Chem. C, 2018, 6, 1504–1508 RSC.
- W. J. Chung and J. Y. Lee, J. Inf. Disp., 2021, 22, 49–54 CrossRef CAS.
- M. I. Alam, M. R. Nagar, S. R. Nayak, A. Choudhury, J. Jou and S. Vaidyanathan, Adv. Opt. Mater., 2022, 10, 2200376 CrossRef CAS.
- K. Bartkowski, P. Zimmermann Crocomo, M. A. Kochman, D. Kumar, A. Kubas, P. Data and M. Lindner, Chem. Sci., 2022, 13, 10119–10128 RSC.
- B. Zhao, Z. Su, W. Li, B. Chu, F. Jin, X. Yan, T. Zhang, F. Zhang, D. Fan, Y. Gao, J. Wang, H. Pi and J. Zhu, Nanoscale Res. Lett., 2013, 8, 529 CrossRef PubMed.
- S. H. Kim, J. Jang, J.-M. Hong and J. Y. Lee, Appl. Phys. Lett., 2007, 90, 173501 CrossRef.
- M. Jakoby, B. S. Richards, U. Lemmer and I. A. Howard, Phys. Rev. B, 2019, 100, 45303 CrossRef CAS.
- H. Hirai, K. Nakajima, S. Nakatsuka, K. Shiren, J. Ni, S. Nomura, T. Ikuta and T. Hatakeyama, Angew. Chem., 2015, 127, 13785–13789 CrossRef.
- T. Hatakeyama, K. Shiren, K. Nakajima, S. Nomura, S. Nakatsuka, K. Kinoshita, J. Ni, Y. Ono and T. Ikuta, Adv. Mater., 2016, 28, 2777–2781 CrossRef CAS.
- G. Liu, H. Sasabe, K. Kumada, A. Matsunaga, H. Katagiri and J. Kido, J. Mater. Chem. C, 2021, 9, 8308–8313 RSC.
- X. Liang, Z. Yan, H. Han, Z. Wu, Y. Zheng, H. Meng, J. Zuo and W. Huang, Angew. Chem., Int. Ed., 2018, 57, 11316–11320 CrossRef CAS PubMed.
- Y. J. Yu, F. M. Liu, X. Y. Meng, L. Y. Ding, L. S. Liao and Z. Q. Jiang, Chem.–Eur. J., 2023, 29, e202202628 CrossRef CAS PubMed.
- S. Ji, G. Xie and Q. Xue, Flexible Printed Electron., 2023, 8, 033003 CrossRef CAS.
- X. Cai, J. Xue, C. Li, B. Liang, A. Ying, Y. Tan, S. Gong and Y. Wang, Angew. Chem., Int. Ed., 2022, 61, e202200337 CrossRef CAS PubMed.
- S. Wu, A. Kumar Gupta, K. Yoshida, J. Gong, D. Hall, D. B. Cordes, A. M. Z. Slawin, I. D. W. Samuel and E. Zysman-Colman, Angew. Chem., Int. Ed., 2022, 61, e202213697 CrossRef CAS PubMed.
- K. Zhang, X. Wang, Y. Chang, Y. Wu, S. Wang and L. Wang, Angew. Chem., Int. Ed., 2023, 62, e202313084 CrossRef CAS PubMed.
- D. Hall, S. M. Suresh, P. L. dos Santos, E. Duda, S. Bagnich, A. Pershin, P. Rajamalli, D. B. Cordes, A. M. Z. Slawin, D. Beljonne, A. Köhler, I. D. W. Samuel, Y. Olivier and E. Zysman-Colman, Adv. Opt. Mater., 2020, 8, 1901627 CrossRef CAS.
- Y. Kondo, K. Yoshiura, S. Kitera, H. Nishi, S. Oda, H. Gotoh, Y. Sasada, M. Yanai and T. Hatakeyama, Nat. Photonics, 2019, 13, 678–682 CrossRef CAS.
- C. Y. Chan, M. Tanaka, Y. T. Lee, Y. W. Wong, H. Nakanotani, T. Hatakeyama and C. Adachi, Nat. Photonics, 2021, 15, 203–207 CrossRef CAS.
- M. Mamada, H. Katagiri, C. Y. Chan, Y. T. Lee, K. Goushi, H. Nakanotani, T. Hatakeyama and C. Adachi, Adv. Funct. Mater., 2022, 32, 2204352 CrossRef CAS.
- H. Lee, R. Braveenth, S. Muruganantham, C. Y. Jeon, H. S. Lee and J. H. Kwon, Nat. Commun., 2023, 14, 419 CrossRef CAS PubMed.
- S. H. Han, J. H. Jeong, J. W. Yoo and J. Y. Lee, J. Mater. Chem. C, 2019, 7, 3082–3089 RSC.
- H. Mubarok, A. Amin, T. Lee, J. Jung, J.-H. Lee and M. H. Lee, Angew. Chem., Int. Ed., 2023, 62, e202306879 CrossRef CAS PubMed.
- K. Stavrou, S. Madayanad
Suresh, D. Hall, A. Danos, N. A. Kukhta, A. M. Z. Slawin, S. Warriner, D. Beljonne, Y. Olivier, A. Monkman and E. Zysman-Colman, Adv. Opt. Mater., 2022, 10, 1–21 Search PubMed.
- Y. Hu, J. Miao, C. Zhong, Y. Zeng, S. Gong, X. Cao, X. Zhou, Y. Gu and C. Yang, Angew. Chem., Int. Ed., 2023, 62, e202302478 CrossRef CAS PubMed.
- K. Stavrou, L. G. Franca, A. Danos and A. P. Monkman, Nat. Photonics, 2024, 18, 554–561 CrossRef CAS.
- S. O. Jeon, K. H. Lee, J. S. Kim, S. G. Ihn, Y. S. Chung, J. W. Kim, H. Lee, S. Kim, H. Choi and J. Y. Lee, Nat. Photonics, 2021, 15, 208–215 CrossRef CAS.
- K. H. Lee and J. Y. Lee, Adv. Opt. Mater., 2020, 8, 2000328 CrossRef CAS.
- K. Kishore Kesavan, J. Jayakumar, M. Lee, C. Hexin, S. Sudheendran Swayamprabha, D. Kumar Dubey, F.-C. Tung, C.-W. Wang and J.-H. Jou, Chem. Eng. J., 2022, 435, 134879 CrossRef CAS.
- K. Goushi and C. Adachi, Appl. Phys. Lett., 2012, 101, 23306 CrossRef.
- K. Goushi, K. Yoshida, K. Sato and C. Adachi, Nat. Photonics, 2012, 6, 253–258 CrossRef CAS.
- Q. Wang, Q. Tian and Y. Zhang, J. Mater. Chem. C, 2019, 7, 11329–11360 RSC.
- M. Sarma and K.-T. Wong, ACS Appl. Mater. Interfaces, 2018, 10, 19279–19304 CrossRef CAS PubMed.
- X.-K. Liu, Z. Chen, C.-J. Zheng, M. Chen, W. Liu, X.-H. Zhang and C.-S. Lee, Adv. Mater., 2015, 27, 2025–2030 CrossRef CAS PubMed.
- K.-H. Kim, C.-K. Moon, J. W. Sun, B. Sim and J.-J. Kim, Adv. Opt. Mater., 2015, 3, 895–899 CrossRef CAS.
- B. Zhao, T. Zhang, B. Chu, W. Li, Z. Su, H. Wu, X. Yan, F. Jin, Y. Gao and C. Liu, Sci. Rep., 2015, 5, 10697 CrossRef PubMed.
- W.-Y. Hung, P.-Y. Chiang, S.-W. Lin, W.-C. Tang, Y.-T. Chen, S.-H. Liu, P.-T. Chou, Y.-T. Hung and K.-T. Wong, ACS Appl. Mater. Interfaces, 2016, 8, 4811–4818 CrossRef CAS PubMed.
- T. B. Nguyen, H. Nakanotani, T. Hatakeyama and C. Adachi, Adv. Mater., 2020, 32, 1906614 CrossRef CAS PubMed.
- B. Liang, J. Wang, Z. Cheng, J. Wei and Y. Wang, J. Phys. Chem. Lett., 2019, 10, 2811–2816 CrossRef CAS PubMed.
- D. Li, Y. Hu and L. S. Liao, J. Mater. Chem. C, 2019, 7, 977–985 RSC.
- Q. Wang, L. Chen, Q. Yang, Y. Xu, H. Wang and Z. Xie, Adv. Mater. Interfaces, 2022, 9, 2200830 CrossRef CAS.
- S.-W. Wen, M.-T. Lee and C. H. Chen, J. Disp. Technol., 2005, 1, 90–99 CrossRef CAS.
- D. Y. Kondakov, Philos. Trans. R. Soc., A, 2015, 373, 20140321 CrossRef PubMed.
- F. Xie, X. Yang, P. Jin, X. Wang, H. Ran, H. Zhang, H. Sun, S. Su and J. Hu, Adv. Opt. Mater., 2023, 11, 2202490 CrossRef CAS.
- H. Nakanotani, K. Masui, J. Nishide, T. Shibata and C. Adachi, Sci. Rep., 2013, 3, 2127 CrossRef CAS PubMed.
- M. Kim, S. K. Jeon, S.-H. Hwang and J. Y. Lee, Adv. Mater., 2015, 27, 2515–2520 CrossRef CAS PubMed.
- S. K. Jeon, H. L. Lee, K. S. Yook and J. Y. Lee, Adv. Mater., 2019, 31, 1803524 CrossRef PubMed.
- Q. Wang and H. Aziz, ACS Appl. Mater. Interfaces, 2013, 5, 8733–8739 CrossRef CAS PubMed.
- K. Thakur, B. van der Zee, G.-J. A. H. Wetzelaer, C. Ramanan and P. W. M. Blom, Adv. Opt. Mater., 2022, 10, 2101784 CrossRef CAS.
- D. Karthik, D. H. Ahn, J. H. Maeng, H. Lee, H. Yoo, R. Lampande, J. Y. Lee and J. H. Kwon, Dig. Tech. Pap. – Soc. Inf. Disp. Int. Symp., 2020, 51, 61–64 CrossRef CAS.
- J. Y. Kim, T. R. Hong, I. R. Choe, J. A. Lee, H. G. Ryu, B. M. Seo, J. H. Yang, C. W. Han, J. H. Baek, H. C. Choi and I. B. Kang, Dig. Tech. Pap. – Soc. Inf. Disp. Int. Symp., 2020, 51, 53–56 CrossRef CAS.
- J.-M. Kim, C.-H. Lee and J.-J. Kim, Appl. Phys. Lett., 2017, 111, 203301 CrossRef.
- A. Pinato, A. Cester, M. Meneghini, N. Wrachien, A. Tazzoli, S. Xia, V. Adamovich, M. S. Weaver, J. J. Brown, E. Zanoni and G. Meneghesso, IEEE Trans. Electron Devices, 2010, 57, 178–187 CAS.
- A. Endo, H. Kakizoe, T. Oyamada and J. Adachi, Dig. Tech. Pap. – Soc. Inf. Disp. Int. Symp., 2020, 51, 57–60 CrossRef CAS.
- J. Adachi, S. Otsu, H. Kakizoe and T. Oyamada, SID Int. Symp. Dig. Tech. Pap., 2021, 52, 232–235 CrossRef CAS.
|
This journal is © The Royal Society of Chemistry 2024 |
Click here to see how this site uses Cookies. View our privacy policy here.