DOI:
10.1039/D4SC05698A
(Review Article)
Chem. Sci., 2024,
15, 18259-18271
Cyclodextrin supramolecular assembly confined luminescent materials
Received
24th August 2024
, Accepted 11th October 2024
First published on 17th October 2024
Abstract
The macrocyclic supramolecular assembly confinement effect not only induces or extends the fluorescence/phosphorescence luminescence behavior of guest molecules but has also been widely applied in the research fields of chemistry, biology, and materials. This review primarily describes recent advances in cyclodextrin (CD) supramolecular assembly confined luminescent materials. Taking advantage of their hydrophobic cavity, CDs and their derivatives effectively encapsulate guest molecules and special functional groups or further assemble and polymerize to restrict the motion of guest chromophores, inducing and enhancing the luminescence behavior and realizing intelligent stimulus-responsive luminescence depending on changes in temperature, light, redox reactions and solvent polarity, which are successfully applied in targeted cell imaging, sensing, information encryption, anti-counterfeiting and flexible electronic light-emitting devices. With the emergence of new chromophores and CD primitives, spatial confinement within CD supramolecular assemblies will further realize the rapid development of supramolecular science and technology in circularly polarized luminescence, fluorescence/phosphorescence cascade energy transfer, light-harvesting energy-transfer systems and long persistent luminescent materials.
1. Introduction
The functional supramolecular assemblies generated by macrocyclic spatial confinement have recently become a hotspot due to their wide application in biomedicine, chemistry, and materials.1–5 Among macrocyclic compounds, cyclodextrin (CD), a kind of cyclopolysaccharide of D-galactose connected by 1,4-glycosidic bonds, has attracted extensive attention due to its low cost, negligible toxicity, and ease of modification.6 Possessing hydrophobic cavities, CDs exhibit a selective encapsulation capability for a range of organic/inorganic molecules with appropriate polarity and size to form macrocyclic spatially confined supramolecular complexes. As a result, α-, β-, and γ-CD molecules, each composed of 6, 7, and 8 glucose units, have become subjects of intensive research endeavors. Although there are numerous reviews that discuss a variety of applications for CDs and CD derivatives, including porous materials,7 contrast agents,8 drug delivery systems,9 catalysis,10 gels/slide-ring materials,11–13 supramolecular polymers,14 saccharide sensors,15 metal complexation,16etc., to the best of our knowledge, CD supramolecular assembly confined luminescent materials including fluorescence, phosphorescence, and smart stimulus-responsive luminescent materials, have rarely been comprehensively reviewed.
Taking advantage of their hydrophobic cavities and polyhydroxy, and modified functional groups, CDs and their derivatives can effectively confine guest molecules through covalent/non-covalent interactions to restrict the motion of chromophores, inducing and enhancing the fluorescence/phosphorescence behavior and further extending the assembly function in response to changes in temperature and solvent polarity to achieve intelligent stimulus-responsive luminescence. When CDs bind with special functional groups, controllable reversible luminescent supramolecular assemblies can be formed by light response and chemical reactions. Moreover, CDs can also be co-assembled with other macrocyclic molecules to form a cascaded supramolecular assembly system or be threaded/modified in polymers to form CD-based supramolecular polymers endowing the assemblies with new properties, such as efficient resonance energy transfer, excellent mechanical performance, and the ability to target cancer cells. In alkaline solution, CDs are capable of forming porous metal–organic frameworks (MOFs) to achieve circularly polarized luminescence (CPL) in the chromophore. On the other hand, the assembly of CDs and lanthanide metal complexes can adeptly regulate the rare-earth luminescence behaviors, realizing tunable multicolor luminescence. It can be seen that CD-based supramolecular assemblies with good biocompatibility, water solubility, and processability are promising luminescent materials with essential applications in cell imaging, sensing, information encryption, anti-counterfeiting, and flexible light-emitting devices.
This review describes recent advances in CD-based supramolecular assembly-confined luminescent materials, mainly including (1) CD-spatially confined guest molecular luminescent materials, (2) CD assemblies with stimulus-responsive luminescence behavior, (3) CD-based polymers and macrocyclic cascade luminescent assemblies, and (4) CD-based nanocages and lanthanide luminescent assemblies. We believe this review would illuminate and help to explore and construct supramolecular luminescence materials (Scheme 1).
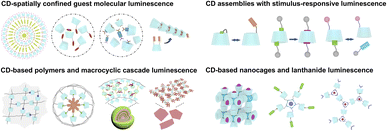 |
| Scheme 1 Schematic representation of four types of CD supramolecular luminescent materials: CD-spatially confined guest molecular luminescent materials, CD assemblies with stimulus-responsive luminescence, CD-based polymers and macrocyclic cascade luminescent assemblies, CD-based nanocages and lanthanide luminescent assemblies. | |
2. CD-spatially confined guest molecular luminescent materials
2.1 CD-confined fluorescent materials
Benefitting from the hydrophobic cavity, various fluorescent chromophores with appropriate molecular sizes can be encapsulated in the cavity of CDs to facilitate non-covalent assembly via host–guest interactions. When functional guest chromophores are confined within the hydrophobic cavity, their fluorescence emission can be induced or enhanced due to the macrocyclic spatial confinement that limits molecular motion to stable singlet excitons and suppresses nonradiative transitions. For example, coumarin derivatives, typical fluorescent chromophores acting as guest molecules, can be assembled with β-CD to form a 1
:
1 complex, resulting in enhanced fluorescence. It also can be covalently linked with benzothiazole, which exhibits good selectivity and affinity for Cu2+, achieving on–off fluorescence via intramolecular charge transfer (ICT).17 Leveraging the advantages of water solubility, membrane permeability, and non-toxicity, the assembly has been successfully applied in monitoring Cu2+ in HeLa cells. Yan et al. constructed a transient self-assembly utilizing α-CD, 4,4′-biphenyl dicarboxylic acid (BPDC), and Zn2+ for dynamically programmable fluorescence applications. In this system, the direct self-assembly of BPDC and Zn2+ formed a cruciform microcrystal that emitted invisible fluorescence in the ultraviolet region. Upon co-assembly with α-CD, slow coordination between BPDC and Zn2+ occurred, leading to an assembly in a new molecular arrangement that emitted visible cyan light. By varying the temperature and molar ratio, the transitory self-assembly's lifespan can be tuned across different timeframes (Fig. 1a).18
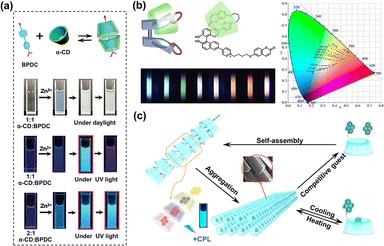 |
| Fig. 1 (a) Schematic illustration of the transient coordinating self-assembly based on host–guest interaction constructed with 4,4-biphenyl dicarboxylic acid (BPDC), α-CD, and Zn2+. This figure has been adapted from ref. 18 with permission from Wiley-VCH GmbH, copyright 2023. (b) A single host–guest complex (BPCY) comprises BPC with two flexible arms and γ-CD, showing adjustable multicolor fluorescence. This figure has been adapted from ref. 19 with permission from American Chemical Society, copyright 2016. (c) Schematic illustration of the assembly processes of dipyrene-substituted γ-CDs demonstrates responsive aggregation to temperature, pH and competitive guests. This figure has been adapted from ref. 21 with permission from Wiley-VCH GmbH, copyright 2022. | |
The encapsulation of CDs with fluorescent compounds can also change the molecular conformation, thereby realizing multicolor luminescence, including white emission based on a single fluorescent chromophore. Concretely, a coumarin alkyl bridged binaphthyl derivative (BPC) with two flexible arms was co-assembled with γ-CD to form a single-molecular self-folding host–guest complex that exhibited distinct dual-emission including blue-green and red light-bands, markedly different from that of the guest alone. By orthogonally modulating the γ-CD host molecule and excitation wavelength, truly colorful photoluminescence emission including nearly pure white-light emission was obtained. The combination of host-activated ICT and restriction of intramolecular rotation provides a possible explanation for the single-molecular self-folding multicolor photoluminescence behavior (Fig. 1b).19
Possessing polyhydroxy properties, CDs have good water solubility and facilitate derivatization; thus, the covalent connection of chromophores to CDs has also been widely studied and applied in the fields of sensing and imaging. Such assemblies have the advantages of strong binding forces and good structural stability, which alter their fluorescence properties and induce guests' CPL as a chiral cyclic molecular scaffold. Kida et al., for instance, created six pyrene-modified α-CD scaffolds (PCDs) that exhibited bright CPL with high molar extinction coefficients and a good dissymmetry factor. It was demonstrated that the covalent linkage of chiral macrocyclic molecular scaffolds with excimer fragments was a new and effective strategy for achieving organic CPL.20 Subsequently, Yang et al. constructed tubular supramolecular polymers with significantly high chiroptical activities by modifying two pyrene groups onto γ-CD. The experimental results indicated that the dipyrene-substituted γ-CD under flexible alkyl chain linkages (AC-P4 and AD-P4) formed a linear supramolecular assembly with one pyrene self-encapsulated into the cavity and another entering the adjacent γ-CD. The resulting tubular supramolecular aggregates exhibited distinct excimer-emission with high CPL brightness and dissymmetric factors. Due to the dynamic assembly of CDs with guest molecules, the assembly mode and its chiroptical properties can be effectively modulated by temperature, pH, and competitive complexation (Fig. 1c).21 Additionally, the configurationally confined CD assembly provides an alternative platform to fabricate clustering-triggered emission (CTE) in a dilute solution. Mao et al. designed supramolecular clustero-luminogens by employing amino acid-modified CDs and CD-based spatial confinement strategies. The hydrogen bond and complexation interaction facilitated conformation rigidification, resulting in bright fluorescence ranging from blue to cyan in a dilute solution. Among them, the histidine-modified CD (CDHis) was proven to be effective for the highly selective fluorescence detection of chlortetracycline (Fig. 2).22
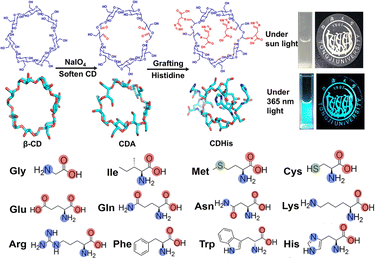 |
| Fig. 2 The clusterization-triggered emission of nonconventional luminophores constructed with amino acid modified β-CD in a dilute aqueous solution. This figure has been adapted from ref. 22 with permission from the authors, copyright 2023. | |
To improve the molecular recognition and assembly characteristics of CDs and expand their applicability, several functional moieties, including carboxyl and sulfate groups, have been modified onto the hydroxyl groups to synthesize multi-charged CDs. These CD derivatives can bind with molecules carrying opposite charges through electrostatic interactions, which not only effectively facilitates the formation of supramolecular assemblies, thereby bolstering their stability and modulating their fluorescent attributes, but also contributes to the light-harvesting systems, which are extensively applied in bioimaging/biosensing. For example, Singh et al. investigated the complexation between 1-pyrene methyl amine (PMA) and a sulfated β-CD derivative (SCD), revealing the primary association of SCD and PMA via electrostatic interactions, which exhibited a high degree of responsiveness to external stimuli, such as solvent polarity, pH, temperature and ionic strength, and achieved biosensing for arginine.23 Our group reported an SCD-based, highly efficient light-harvesting energy transfer system composed of SCD, an oligo(phenylenevinylene) derivative (OPV-I), and Nile red (NiR).24 SCD assembled with OPV-I to form nanospheres, improving the water solubility of aggregation-induced emission (AIE) guest molecules and achieving an obvious fluorescence enhancement. NiR, as an acceptor, was further doped in the hydrophobic layer of OPV-I/SCD, resulting in efficient fluorescence resonance energy transfer (FRET) with a high antenna effect (Fig. 3a). Subsequently, stimulus-responsive molecules like diarylethene derivatives as energy acceptors were introduced into these systems to obtain a photo-controlled light-harvesting supramolecular assembly system. It could co-assemble with polyanionic γ-CD, pyrene derivatives, and NiR to form a multi-component self-assembly nanoparticle, which enabled an excellent photo-controlled energy transfer process, achieving a reversible fluorescence switch regulated by UV or visible light (Fig. 3a).25
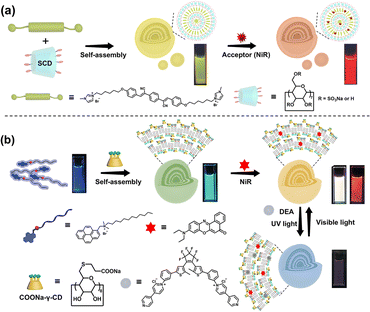 |
| Fig. 3 (a) An artificial supramolecular light-harvesting system constructed by co-assembling OPV-I, SCD, and NiR. This figure has been adapted from ref. 24 with permission from Wiley-VCH GmbH, copyright 2017. (b) A photo-controlled light-harvesting system constructed using a pyrene derivative, NiR, polyanionic γ-CD and a diarylethene derivative. This figure has been adapted from ref. 25 with permission from Wiley-VCH GmbH, copyright 2020. | |
2.2 CD-confined phosphorescent materials
In addition to fluorescent materials, the CD-confined guest chromophore approach is a popular strategy for creating room-temperature phosphorescent (RTP) materials due to its simple operation, good luminescence performance, and excellent water solubility. The supramolecular self-assembly of CDs with phosphors can effectively restrict guest molecules' motion and eliminate the quenching effect of water through CD-spatial confinement, thereby suppressing non-radiative transitions and achieving RTP emission in aqueous or solid phases. As early as 1982, Turro and co-workers discovered the phosphorescence emission from a β-CD and 1-bromonaphthalene/1-chloronaphthalene co-assembled aqueous solution system under a nitrogen environment.26 Recently, Li et al. reported an aqueous-phase ultralong RTP system obtained from p-biphenyl boronic acid and β-CD, which exhibited a second-level phosphorescence lifetime of 1.03 s. The persistent RTP behavior has been shown to depend on host–guest encapsulation and hydrogen bonding interactions, which effectively suppress nonradiative relaxation (Fig. 4a).27 Subsequently, our group constructed a β-CD-based solid supramolecular blue RTP material with terephthalic acid (PPA) as the guest molecule.28 Based on the β-CD-confinement to isolate and restrict the PPA chromophore, the supramolecular assembly PPA-CD exhibited an efficient deep-blue phosphorescence with high conversion efficiency. The secondary assembly of PPA-CD and poly(vinylalcohol) (PVA) resulted in a flexible processable supramolecular film, further enhancing the phosphorescence emission through hydrogen bond networks and presenting reversible thermal stimulus–response properties reflected by a blue and cyan phosphorescence color change. The thermally controlled binding behavior of PPA and β-CD was responsible for the thermochromism luminescence. These thermochromic phosphorescence materials have been successfully applied in multilevel information encryption, providing a simple supramolecular platform to fabricate temperature-responsive multicolor RTP materials (Fig. 4b).
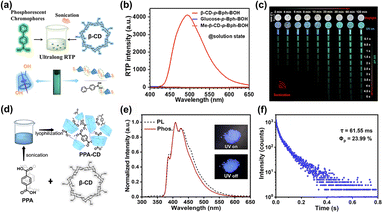 |
| Fig. 4 (a) Schematic illustration of supramolecular assembly β-CD-p-Bph-BOH with ultralong RTP emission in aqueous solution. (b) Phosphorescence spectra for the assemblies of p-Bph-BOH with different host molecules. (c) Afterglow images of the β-CD-p-Bph-BOH system under different sonication times. These figures have been adapted from ref. 27 with permission from American Chemical Society, copyright 2023. (d) Schematic illustration of the β-CD-based supramolecular assembly PPA-CD with deep-blue RTP. (e) Normalized steady-state PL and phosphorescence spectra of PPA-CD powder. Inset: photographs of PPA-CD under 300 nm UV irradiation (on/off). (f) Time-resolved decay spectrum and quantum yield for PPA-CD. These figures have been adapted from ref. 28 with permission from Wiley-VCH GmbH, copyright 2024. | |
Moreover, Ma et al. pioneered the synthesis of amorphous organic small molecule RTP materials by connecting chromophores to β-CD.29 Specifically, four organic phosphorescent functional groups were reacted with mono[6-O-(4-methyl-phenylsulfonyl)]-β-CD or 6-amino-β-CD, yielding a variety of CD derivatives. The strong intermolecular hydrogen bonds within β-CD effectively restricted the vibration of phosphorescent molecules and mitigated the impact of quenchers, thereby inducing colorful RTP emission. By utilizing CD derivatives' cavities to encapsulate the amantadine–coumarin fluorescent groups, excellent dual emissions of RTP and fluorescence can be achieved, ultimately producing multicolor photoluminescence, including white light emission (Fig. 5). Subsequently, they further covalently linked a series of benzaldehyde and benzoic acid derivatives to β-CD to obtain blue amorphous RTP materials. Compared to traditional crystalline RTP materials, this heavy-atom-free amorphous small molecule RTP material exhibits superior properties for applications in bioimaging, information storage, and light-emitting devices.30
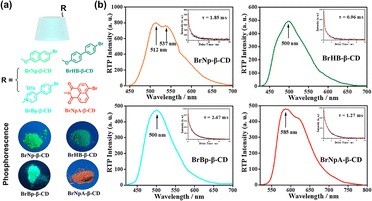 |
| Fig. 5 (a) Schematic illustration of amorphous small molecule RTP emissive β-CD derivatives and their photographs under UV light. (b) Phosphorescence and time-resolved decay spectra for these β-CD derivatives in the solid state. This figure has been adapted from ref. 29 with permission from American Chemical Society, copyright 2018. | |
Despite the excellent RTP performance of the macrocyclic self-assemblies of CDs and phosphors, it is necessary to develop supramolecular polymer materials for their practical application. On the one hand, the synergistic assembly of macromolecules and polymers can provide a rigid network that further restricts chromophore motion, thereby suppressing non-radiative transitions and improving luminescence performance.31,32 For example, our group reported a solid-state pure blue phosphorescent material based on a supramolecular assembly and in situ photopolymerization strategy using β-CD-isoquinoline, cucurbit[7]uril (CB[7]) and acrylamide.33 The polymer rigid network and the encapsulation of CB[7] immobilized the 6-bromoisoquinoline chromophore, achieving pure blue RTP emission at 450 nm with enhanced luminous performance. Benefiting from the excellent water solubility and processability of the supramolecular assembly, this system showed great potential for applications in writable materials and information encryption (Fig. 6a). Tian et al. constructed a supramolecular polymer system based on the host–guest recognition between γ-CD and 4-bromo-1,8-naphthalic anhydride derivative-based polyacrylamide copolymers, realizing aqueous RTP emission. Interestingly, the RTP properties of this system could be controlled by introducing poly-azobenzene, relying on competitive complexion and photoisomerization (Fig. 6b).34 This study enriches the construction strategies for intelligent light-stimulus response RTP materials. On the other hand, the flexibility and processability of polymers greatly expand the application range of solid supramolecular RTP materials. For example, the assembly of γ-CD and PVA, modified in situ with triphenylene boronic acid, successfully produced a moldable, flexible transparent film with minute-level afterglow.35 The extensive hydrogen-bonding network formed from co-assembling γ-CD and PVA chains, and CD-spatially confined chromophores effectively suppressed the nonradiative decay and promoted the long-lived RTP emission. Significantly, the resulting long afterglow flexible material can be fabricated into security inks, processable full-color afterglow objects, and noctilucent light sources (Fig. 6c).
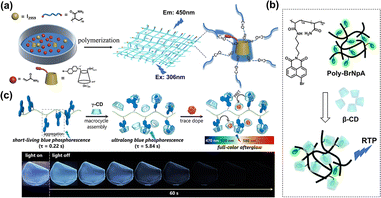 |
| Fig. 6 (a) Schematic illustration of a long-lived blue RTP supramolecular material co-assembled from β-CD-isoquinoline, CB[7] and polyacrylamide. This figure has been adapted from ref. 33 with permission from Wiley-VCH GmbH, copyright 2022. (b) The supramolecular assembly of γ-CD and a 4-bromo-1,8-naphthalic anhydride derivative-based polyacrylamide copolymer achieves RTP emission in an aqueous solution. This figure has been adapted from ref. 34 with permission from The Royal Society of Chemistry, copyright 2016. (c) Schematic illustration of the fabrication process for CD-based multicolor flexible RTP materials with minute-level afterglow. This figure has been adapted from ref. 35 with permission from the authors, copyright 2023. | |
Additionally, the co-assemblies of multi-charged CDs and guest chromophores carrying opposite charges, which rely on electrostatic interactions, can also effectively confine the phosphors to improve the RTP performance. Our group has synthesized a bromonaphthylpyridinium polymer (P-BrNp), and subsequently integrated it with sulfobutylether-β-CD through electrostatic interactions, creating a polymeric complex with marked RTP enhancement.36 In this system, P-BrNp had dual emission characteristics of fluorescence and RTP, culminating in an impressive white light emission with a quantum yield of up to 83.9%. The co-assembly with sulfobutylether-β-CD enhanced the phosphorescence emission of P-BrNp, transforming photoluminescence from white to yellow. Moreover, the incorporation of a photoresponsive divinyl monomer facilitated the phosphorescence resonance energy transfer (PRET) process, enabling a reversible on–off RTP emission, which was successfully utilized in controllable data encryption and multifunctional writable materials (Fig. 7).
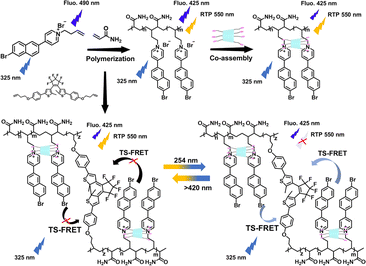 |
| Fig. 7 Schematic illustration of a solid supramolecular polymer with tunable ultra-strong white-light emission constructed using bromonaphthylpyridinium polymerization, sulfobutylether-β-CD, and a diarylethene derivative. This figure has been adapted from ref. 36 with permission from Wiley-VCH GmbH, copyright 2022. | |
3. CD assemblies with stimulus-responsive luminescence behavior
The CD-based intelligent supramolecular assemblies respond to chemical stimuli and environmental changes, including pH, temperature, and oxygen;37–39 they can not only achieve reversible topological morphological regulation via assembly/disassembly processes but also effectively alter the luminescence properties of materials, making them well-suited for applications in bioimaging, sensing, anti-counterfeiting and molecular switches. Azobenzene and anthracene, as two types of stimulus-responsive functional groups, have been extensively studied in CD-based intelligent assembly luminescent materials, owing to their compatible molecular sizes with CD cavities. The trans-azobenzene unit can be selectively encapsulated in the hydrophobic cavity of CDs, while the cis-structure cannot. On this basis, Ma et al. constructed a ternary photocontrollable reversible pseudo-rotaxane using sodium 2-hydroxy-5-((4-nitrophenyl)diazenyl)benzoate, β-CD and the competitive guest α-bromonaphthalene (Fig. 8a),40 and further covalently modified the azobenzene onto the CD to construct a binary RTP photoresponsive material (Fig. 8b),41 providing a simple and effective pathway for developing intelligent RTP materials. Our group reported a non-covalent hypoxia-responsive ternary fluorescent supramolecular probe created from SCD, azobenzene-pyrazole salt (1), and fluorochrome rhodamine 123 (Rho123).42 The SCD/1 complex exhibited an excellent fluorescence quenching effect on Rho123 through photoinduced electron transfer (PET). Under hypoxic conditions, the azobenzene groups were reduced, releasing Rho123 to recover fluorescence, which was successfully applied for hypoxic cell imaging (Fig. 8c).
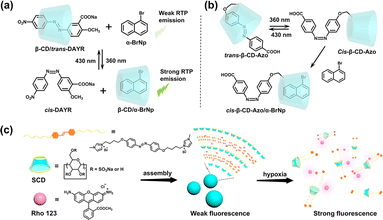 |
| Fig. 8 (a) The reversible assembly mode based on the β-CD/DAYR/α-BrNp ternary system for photocontrolled RTP emission. (b) Photoresponse phenomenon of pseudorotaxane β-CD-Azo and the co-assembly with α-BrNp. (c) Schematic illustration of a non-covalent hypoxia-responsive ternary fluorescent supramolecular probe. This figure has been adapted from ref. 42 with permission from American Chemical Society, copyright 2022. | |
Anthracene and its derivatives have significant photoreactivity and can be easily photo-oxidized to anthraquinones under ultraviolet light to achieve smart stimulus-responsive photo-switching or undergo photodimerization, which can be reversibly transformed by photoreversible dynamic covalent bonds for cross-linking and fixing of assemblies. Therefore, many anthracene-CD-based photo-controllable supramolecular systems have been reported. For example, by covalently linking anthraquinone with β-CD through an alkyl chain (AQ-β-CD), the β-CD cavity can encapsulate the anthraquinone unit and self-assemble into linear nanofibers with special oxygen responsiveness and photoreduction luminescence capabilities.43 Due to the hydrophobic nature of the CD cavity coupled with its protective barrier against oxygen molecules, the anthraquinone units can be rapidly photo-reduced to 9,10-anthracenediol, generating strong yellow fluorescence, followed by conversion back to anthraquinone by oxidation of oxygen. It has been successfully applied as fluorescent ink for information encryption (Fig. 9a). Ma et al. crafted a polymer featuring 2-anthracenecarboxylic ester (AC) units and have embedded photo-reversible dynamic covalent bonds through the γ-CD-mediated photo-crosslinking process. This innovative approach facilitated the mutual conversion of poly-AC-CD and poly-An-CD under different light irradiation accompanied by a transition between blue light and cyan phosphorescence, which greatly expanded the potential applications of intelligent optical materials (Fig. 9b).44
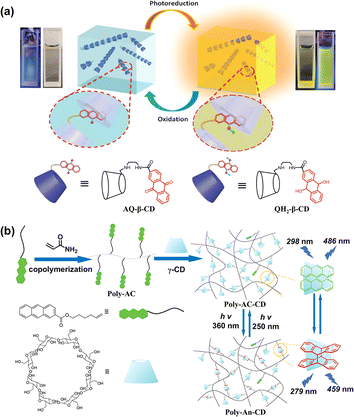 |
| Fig. 9 (a) Photoreduction and oxidation luminescence properties of AQ-β-CD-based supramolecular non-covalent polymers in aqueous solution. This figure has been adapted from ref. 43 with permission from the authors, copyright 2020. (b) Schematic diagram of dynamic self-assembly of γ-CD and anthracene-acrylamide copolymers and its reversible photo-responsiveness. This figure has been adapted from ref. 44 with permission from Wiley-VCH GmbH, copyright 2021. | |
Furthermore, functional derivatives of CDs have been shown to effectively enhance the binding affinity of functional groups for optical sensing and biosensing. Liu et al. attached a coumarin derivative to β-CD to construct a self-included ratiometric fluorescent sensor, significantly enhancing its binding affinity to glutathione (GSH) compared to the guest alone and finally realizing dynamic sensing of GSH in HeLa cells (Fig. 10a).45 The same authors further developed a boron-dipyrromethene (BODIPY)-modified β-CD for the detection of the glutathione thiyl radical (G
), demonstrating an excellent ratiometric fluorescence response featuring both green and red emissions with a fast response time, which was successfully used for G
detection in living A549 cells (Fig. 10b).46
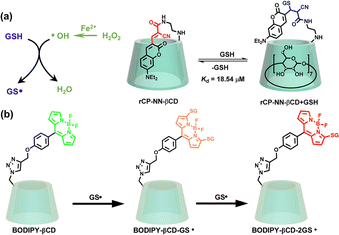 |
| Fig. 10 (a) Schematic illustration of the self-included assembly of rCP-NN-βCD and its reversible response to GSH through the Michael addition reaction. This figure has been adapted from ref. 45 with permission from The Royal Society of Chemistry, copyright 2023. (b) Boron-dipyrromethene (BODIPY)-modified β-CD as a ratio-metric fluorescent probe for detecting glutathione thiyl radicals. This figure has been reproduced from ref. 46 with permission from Wiley-VCH GmbH, copyright 2021. | |
4. CD-based polymers and macrocyclic cascade luminescent assemblies
4.1 CD-based macrocyclic cascade self-assembly
The supramolecular complexes based on CD spatial confinement can also be collaboratively assembled with other macrocyclic host molecules such as cucurbit[n]uril (CB[n]), which provides more options for developing high-performance fluorescent/phosphorescent systems, resonance energy transfer systems, and intelligent stimulus-responsive materials. As depicted in Fig. 11a, the β-CD modified bromophenyl pyridine derivative, serving as a phosphor guest, can be co-assembled with CB[8] through cation–dipole interactions and hydrophobic effects to form a supramolecular complex in a 2
:
1 stoichiometric ratio. Benefiting from the β-CD cavity's effective binding with rhodamine B (RhB), the distance between the energy donor, bromophenyl pyridine, and the RhB acceptor was significantly reduced, leading to the successful construction of a light-harvesting PRET system with a high energy transfer efficiency of up to 84% and an ultra-high antenna effect of 36.42.47 In Fig. 11b, by leveraging the encapsulation of α-CD with azobenzene and the stabilization of charge-transfer interactions between naphthalene and a bispyridinium guest within CB[8], a multi-component linear supramolecular non-covalent polymer was successfully established. Due to the photo-induced isomerization of the azobenzene moieties and the chemical reduction of the bispyridinium units, this linear non-covalent polymer demonstrated a pronounced sensitivity to various stimuli, including temperature, light irradiation, and chemical redox processes, ultimately achieving the assembly and disassembly of supramolecular nanostructures accompanied by different luminescence characteristics.48 Multi-charged CD derivatives like sulfobutylether-β-CD (SBE-βCD) are also an essential primary medium for developing multicomponent macrocyclic cascade assembly luminescent materials. For example, our group constructed a multi-level supramolecular assembly based on SBE-βCD and CB[8], realizing topological morphology transformation and multicolor luminescence regulation.49 In this system, the guest molecule tetraphenylethylene pyridinium salt (TPE-Py) was assembled in a cascade with CB[8] and SBE-βCD via host–guest encapsulation and electrostatic interactions, respectively, culminating in the formation of nanosheets with a significant enhancement in fluorescence intensity (20 times). While the primary assembly of TPE-Py with SBE-βCD alone resulted in nanoparticles with a relatively weaker enhancing effect, attributed to the absence of the CB[8]-confinement effect. Upon the incorporation of the near-infrared (NIR) dye, sulfonated aluminum phthalocyanine (AlPcS4), as an energy acceptor, the cascade assembly nanosheet structure exhibited an enhanced energy transfer efficiency of 75% and a pronounced antenna effect of 29.3, surpassing that of the nanoparticles, which was successfully applied to multiple luminescent information storage applications (Fig. 11c).
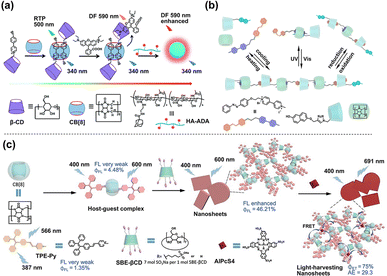 |
| Fig. 11 (a) A light-harvesting PRET supramolecular assembly composed of bromophenyl-pyridine-modified β-CD, CB[8] and RhB. This figure has been adapted from ref. 47 with permission from the authors, copyright 2021. (b) A supramolecular polymer consisting of naphthalene-modified α-CD, an azobenzene-dipyridine derivative, and CB[8] showed responsiveness to various external stimuli. (c) Schematic Illustration of multistage assembly between TPE-Py, CB[8] and SBE-βCD, and the diagram of the FRET process with AlPcS4. This figure has been adapted from ref. 49 with permission from Wiley-VCH GmbH, copyright 2023. | |
4.2 CD-based flexible polyrotaxane luminescent materials
Unlike CD-based host–guest complexes and macrocyclic collaborative assemblies, using CD-threaded polymers to form polyrotaxane and pseudopolyrotaxane has become a widely used strategy to construct supramolecular soft materials,50–52 which can effectively improve the mechanical properties and offer a potential pathway for constructing intelligent luminescent materials. Utilizing the stretchability of CD-based supramolecular slide-ring polyrotaxanes, reversible mechanoresponsive dynamic regulation can be successfully applied to mechanochromic luminescence materials. For example, our group fabricated a multifunctional mechanochromic slide-ring supramolecular elastomer by copolymerizing a mechanoresponsive acrylate-modified perylene diimide derivative with 2-(2-methoxyethoxy)ethyl acrylate, and a polyrotaxane cross-linker which was crafted by threading acrylate-modified α-CD on polyethylene glycol (PEG) and securing the ends with adamantine stoppers. The elastomer possessed enhanced mechanical reversibility inherent to slide-ring materials and displayed significant mechanochromic fluorescence changes through the reversible dimer-to-monomer transformation of perylene diimide molecules.53 Furthermore, based on host–guest complexation and molecular size effects, an efficient phosphorescent pseudopolyrotaxane system was constructed using the molecular recognition between the polymer-modified phosphorescent chromophore and CDs, which was significantly applied in the development of luminescent gels and intelligent stimulus-responsive materials. Specifically, by threading α-CD through the linear bromobenzaldehyde bridged PEG, a supramolecular polypseudorotaxane dry hydrogel material was successfully constructed via hydrogen bonding and hydrophobic interactions.54 The spatial confinement provided by CD for brominated aromatic aldehyde induced RTP emission at 550 nm, leading to white light emission based on the combined fluorescence–phosphorescence properties. Interestingly, the photoluminescence of this polypseudorotaxane dry hydrogel can be reversibly switched from blue to white light emission in response to moisture, endowing the material with intelligent stimuli-responsive luminescence characteristics (Fig. 12a). Subsequently, utilizing the recognition and encapsulation capabilities of α-CD for benzene and naphthalene derivatives, our group assembled these two luminophore-modified PEG derivatives with α-CD to construct excitation-dependent and time-dependent multicolor persistent RTP materials. By introducing diarylethylene (DAE) groups, photoresponsive multicolor RTP switching can be achieved owing to the photoisomerization of DAE and energy transfer, enriching the application prospects of intelligent luminescent supramolecular systems in information storage and processing (Fig. 12b).55
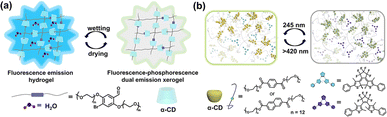 |
| Fig. 12 (a) Schematic illustration of a CD-based polypseudorotaxane xerogel exhibiting RTP and water-stimulated switchable white-light emission features. This figure has been adapted from ref. 54 with permission from Wiley-VCH GmbH, copyright 2019. (b) Schematic illustration of photoreversible multicolor RTP supramolecular pseudopolyrotaxanes. This figure has been adapted from ref. 55 with permission from Wiley-VCH GmbH, copyright 2022. | |
Taking advantage of strain-induced crystalline domains formed by CDs on pseudopolyrotaxane,56 the non-radiative transition and motion of the chromophores can be effectively inhibited, thereby significantly increasing its phosphorescence intensity. In our recent study, we reported a supramolecular elastomer constructed from waterborne polyurethane (WPU) and a pseudopolyrotaxane system of α-CD threaded naphthalene-modified PEG, endowing it with reversible mechanical stretching-induced RTP enhancement behavior.57 CDs, enriched with hydroxyl groups, not only achieved persistent RTP emission through the macrocyclic confinement effect and hydrogen bonding with WPU but also realized a strain-induced crystallization toughening effect under stretching conditions, with a threefold increase in phosphorescence intensity at 200% strain. This supramolecular elastomer exhibited ultra-high RTP stability in water and chemical environments, as well as at high temperatures, effectively expanding the application range of supramolecular phosphorescent materials (Fig. 13).
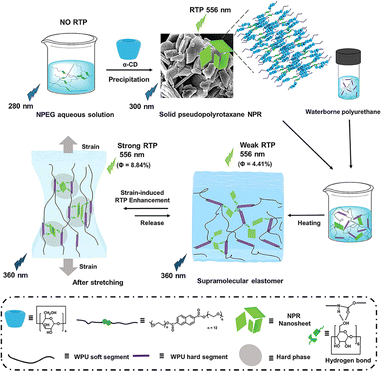 |
| Fig. 13 Schematic illustration of α-CD pseudopolyrotaxane elastomers exhibiting reversible mechanical stretching-induced RTP enhancement behavior. This figure has been reproduced from ref. 57 with permission from the authors, copyright 2024. | |
4.3 CD-based supramolecular polymer luminescent materials
When CDs are co-polymerized with functional fluorophores or polymer monomers, CD-based supramolecular polymers can be constructed58 with excellent performance in catalysis, drug delivery, environmental applications, and materials science, especially in luminescent materials. For example, CDs can be co-polymerized with tetraphenylethylene derivatives to construct stable supramolecular networks with solid luminescence capability.59 The undisturbed CD cavities adsorb dye molecules to establish the FRET process, realizing multicolor luminescence, which provides an alternative platform for developing photoluminescent tunable supramolecular solid materials (Fig. 14). On the other hand, CD derivatives can also be used to prepare CD-modified polymers through copolymerization with vinyl groups or condensation polymerization with other unique functional groups.60 The multivalent interactions between polymers, polymers and CDs, as well as CDs and guests not only promote molecular recognition and self-assembly but can also immobilize the chromophores and inhibit quenching agents to improve luminous properties. For example, Tian et al. reported a self-healing, photo-activated RTP supramolecular polymeric gel based on β-CD-modified polyacrylamide and poly-α-bromonaphthalene.61 The supramolecular gel exhibited CD-induced RTP emission and self-healing properties owing to the host–guest specific recognition and complexation effect of β-CD with the phosphorescent group. Then, they further introduced an azobenzene guest polymer (poly-azo) to construct a ternary supramolecular polymer. Under irradiation, the azobenzene unit engaged in a dynamic tunable competitive coordination process with β-CD, enabling the reversible regulation of the RTP signal (Fig. 15a).
 |
| Fig. 14 Schematic illustration of a porous polymer of tetraphenylethene crosslinked β-CD that can further encapsulate fluorescent groups and use the FRET process to prepare photo-induced luminescent tunable organic solid materials. This figure has been reproduced from ref. 59 with permission from The Royal Society of Chemistry, copyright 2018. | |
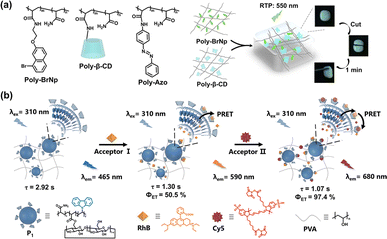 |
| Fig. 15 (a) Schematic illustration of a CD-based supramolecular polymer hydrogel with rapid self-healing properties and photo-responsive RTP emission. This figure has been adapted from ref. 61 with permission from Wiley-VCH GmbH, copyright 2014. (b) An efficient PRET solid supramolecular system was constructed through the polymerization of modified β-CD for multicolor-delayed fluorescence anti-counterfeiting. This figure has been adapted from ref. 62 with permission from Wiley-VCH GmbH, copyright 2023. | |
Moreover, CD-modified supramolecular polymers have been demonstrated to enhance the PRET process, leading to the induction of effective multicolor-delayed fluorescence. As a case in point, Liu et al. reported a solid supramolecular terpolymer formed by the copolymerization of acrylamide, carbazole (CZ), and a β-CD derivative. The supramolecular polymer was co-doped with PVA and dyes (RhB and Cy5) to fabricate a highly efficient tandem PRET system, achieving a NIR afterglow with a lifetime of up to 1.07 s.62 The experimental findings indicated that the CD-copolymer could effectively enrich dye molecules, bringing the donor and acceptor closer and promoting the PRET process. Additionally, by tuning the excitation wavelength, the ratio of donor to acceptor molecules, and the environmental stimuli of moisture and dryness, the flexible polymer film has been endowed with adjustable luminescence lifetime and color, along with a repeatable switchable afterglow, which has been effectively harnessed for applications in information security and anti-counterfeiting measures (Fig. 15b).
4.4 HACD-based self-assembly for targeted imaging
Among various CD-modified polymers, CD-modified hyaluronic acid (HACD) has garnered the most significant research attention, attributed to its good water solubility, high biocompatibility, and easy processability. The cavity of CD is proficient at encapsulating photosensitizers, fluorescent chromophores, and other size-matched molecules to form multifunctional supramolecular assemblies. Hyaluronic acid (HA) not only confers the complex with the ability to target cancer cells but also enhances biocompatibility and stability through multivalent interactions, expanding its biological applications. First, HACD can co-assemble with AIE guest molecules to directly induce their aggregation to form primary assembly nanoparticles, achieving supramolecular aggregation-induced emission in aqueous solutions. For example, adamantane-modified tetraphenylethylene as guest molecules was co-assembled with β-CD-modified hyaluronic acid to form well-dispersed nanoparticles with 30–50 nm diameters, exhibiting enhanced AIE fluorescence.63 By taking advantage of the specificity of HA for targeting HA receptor-positive cancer cells, targeted fluorescence imaging of cancer cells was achieved (Fig. 16a). HACD can also serve as a secondary assembly matrix to co-assemble with CB[n] primary assembly units, restricting the nonradiative transitions of the assembly through multivalent interactions, thereby further enhancing the fluorescence or phosphorescence emission of the assembly. Our group reported an HACD-based macrocycle-confined multivalent supramolecular assembly integrated with alkyl-bridged triphenylamine-vinyl pyridinium, a 4-bromophenyl pyridine salt derivative, CB[8] and β-CD-grafted hyaluronic acid, which exhibited macrocycle-confined cascade-enhanced dual-emission properties, including green RTP and two-photon NIR fluorescence.64 Cellular and in vivo imaging studies have demonstrated that the cascade assembly was adeptly employed for targeted imaging of cancer cells, circumventing the interference posed by background spontaneous fluorescence, which revealed its significant potential for applications in biological theragnostics (Fig. 16b).
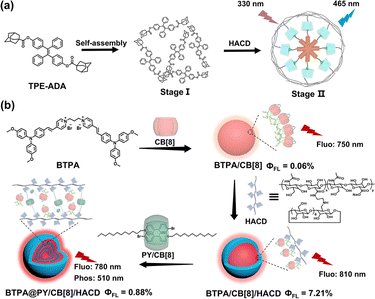 |
| Fig. 16 (a) Schematic illustration of the supramolecular assembly of a tetraphenylethylene derivative and HACD with enhanced AIE fluorescence. This figure has been adapted from ref. 63 with permission from American Chemical Society, copyright 2020. (b) A supramolecular cascade assembly based on HACD has macrocycle-confined dual-emission properties, including green RTP and two-photon NIR fluorescence. This figure has been adapted from ref. 64 with permission from Wiley-VCH GmbH, copyright 2023. | |
Additionally, the cascade assemblies based on HACD are capable of encapsulating a variety of commercial dye molecules as energy transfer acceptors to construct PRET systems in an aqueous solution, which is widely applied in NIR delayed fluorescence targeted cell imaging, significantly enhancing the signal-to-noise ratio and eliminating background fluorescence interference. Our group has constructed an efficient non-covalent polymerization-activated RTP harvesting system with NIR-delayed fluorescence through the cascade assembly of CB[7], HACD, 6-bromoisoquinoline derived phosphorescent groups, and organic NIR-dyes.65 It was found that CB[7] can effectively bind with 6-bromoisoquinoline derivatives to induce RTP emission at 540 nm. The further assembly of HACD facilitated non-covalent polymerization, not only causing an 8-fold enhancement in the initial phosphorescence intensity and an extension of the phosphorescence lifetime from 59.0 μs to 0.581 ms but also achieving efficient PRET upon introduction of dyes, resulting in long-lifetime NIR delayed fluorescence, which has been effectively utilized for the targeted NIR imaging of lysosomes within cancer cells (Fig. 17).
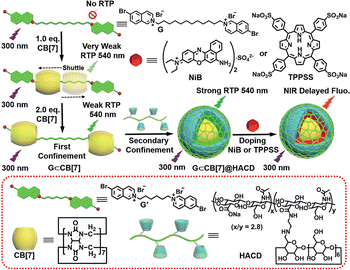 |
| Fig. 17 Schematic illustration of a HACD-mediated, noncovalent polymerization-activated ultra-strong NIR PRET system in aqueous solution. This figure has been reproduced from ref. 65 with permission from Wiley-VCH GmbH, copyright 2022. | |
Capitalizing on the cavity encapsulation and electrostatic interactions, HACD can also bind with supramolecular assemblies as energy acceptors to construct PRET systems with a large Stokes shift and even realize single-molecule PRET behavior accompanied by multi-level topological morphology regulation. As shown in Fig. 18a, phenyl-bridged bis(triphenylamine)-tetracationic salt derivative (G1) was co-assembled with CB[8] to form a reticular organic two-dimensional nanosheet with a stoichiometric ratio of 1
:
2, which exhibited NIR fluorescence at 810 nm. Notably, this assembly G1/CB[8], as an energy acceptor, could be further co-assembled with RTP donors and α-CD-modified hyaluronic acid to form irregular nanoparticles through electrostatic interactions and encapsulation of benzoate groups by CD cavities, thus facilitating an efficient PRET process to obtain NIR delayed fluorescence at 810 nm with a notable Stokes shift of 502 nm.66 Furthermore, we reported a HACD-mediated single-molecule PRET system that showed a large Stokes shift and emitted NIR delayed fluorescence emission. This system was meticulously constructed using a tetraphenylethylene-phenylpyridine derivative (TPE-DPY), CB[n] (n = 7,8), and β-CD-modified hyaluronic acid. Leveraging the distinct binding affinities, the primary assembly of CB[n] (n = 7,8) to TPE-DPY endowed the system with CB-confinement-induced RTP emission and tunable topological morphologies, transitioning from nanospheres to nanorods and further to nanoplates. The secondary assembly, driven by HACD, initiated an intramolecular PRET process from phenyl pyridine moieties to the methoxy-tetraphenylethylene units, enabling NIR delayed fluorescence emission at 700 nm. This sophisticated macrocycle confined and polysaccharide-activated PRET system has been effectively utilized for mitochondria-targeted imaging in cancer cells (Fig. 18b).67
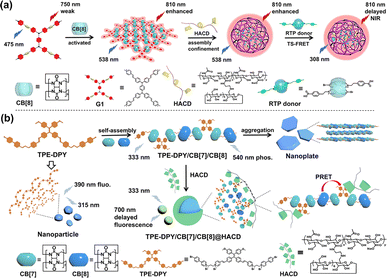 |
| Fig. 18 (a) Schematic illustration of the cascade assembly based on aromatic bridged-double (trianiline), CB[8], and HACD, accompanied by effective NIR luminescence with a large Stokes shift through PRET. This figure has been adapted from ref. 66 with permission from American Chemical Society, copyright 2023. (b) Schematic illustration of the supramolecular self-assembly between TPE-DPY, CB[7], and CB[8], as well as HACD-mediated single-molecule PRET for NIR targeted cell imaging. This figure has been adapted from ref. 67 with permission from the authors, copyright 2024. | |
5. CD-based nanocages and lanthanide luminescent assemblies
5.1 CD-MOF luminescent material
Stoddart et al. first proposed the construction of porous metal–organic frameworks (CD-MOFs) using alkali metal ions K+ and γ-CD that formed a body-centered cubic arrangement of (γ-CD)6 cubes connected by eight-coordinate K+ ions.68 Subsequently, they found that alkali metals such as Na, Rb, and Cs salts could also be easily obtained to form other CD-MOFs with isostructural frameworks, thereby enriching the porous material family.69 Taking advantage of the chiral feature and inherent cubic voids of γ-CD-MOF, Liu and co-workers reported a crystalline CPL material constructed from γ-CD-MOFs and achiral luminescent groups with different charges and sizes that cover both the aggregation-induced quenching (ACQ) and AIE molecules.70 The research demonstrated that the chiral cubic voids of γ-CD-MOFs exhibited significant guest size selectivity and effectively induced CPL. Specifically, small luminescent groups tended to be encapsulated into the γ-CD cavities, while large luminescent groups were encapsulated in the cubic voids, inducing positive or negative CPL. A pronounced negative CPL signal could be obtained when the dimensions of the luminophore closely matched the cubic voids. This work presents a versatile supramolecular approach for preparing crystalline CPL materials harnessing diverse achiral luminescent groups (Fig. 19). Furthermore, core–shell structured CD-MOFs were developed for multicolor luminescent functional materials. Based on the effective inclusion of fluorescein and RhB by γ-CD, Gong et al. encapsulated different dye guest molecules into γ-CD-MOF to construct core–shell crystals with tunable thickness and different dye layers through an epitaxial seed growth approach.71 Importantly, the γ-CD cavity and the cage in the MOF showed a significant synergistic effect on fluorescence enhancement. An efficient white light emission could be effectively achieved by leveraging the superposition of the emission spectra of various dyes in the core–shell structure.
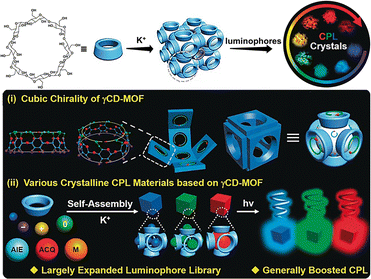 |
| Fig. 19 A crystalline CPL material constructed using γ-CD-MOFs and achiral luminescent groups with different charges and sizes. This figure has been adapted from ref. 70 with permission from Wiley-VCH GmbH, copyright 2020. | |
5.2 Lanthanide luminescence based on CDs
Various lanthanide supramolecular luminescent systems based on CD have been constructed and intensely studied, endowing luminescent systems with numerous characteristics and advantages.72–74 On the one hand, incorporating CD hosts into rare earth luminescent materials is a proven strategy for improving their aqueous solubility. Since CDs occupying a significant volume act as part of a ligand, they can hinder the coordination between water and rare earth ions to reduce the hydration of rare earth complexes in aqueous solutions, enhancing the luminescence of rare earth materials and expanding their application fields. On the other hand, the orderly, controllable, and diverse assembly patterns of CD-based supramolecular systems endow rare earth systems with dynamic controllability and intelligent responsiveness. For example, Liu et al. modified the pyridine dicarboxylic acid ligand with a photo-responsive anthracene group to construct a photo-tunable water-soluble supramolecular assembly with rare earth Eu and γ-CD (Fig. 20a).75 This assembly exhibited dual emission, including red light emission from Eu(III) and blue light emission from the anthracene-modified pyridine dicarboxylic acid. By controllably adjusting the illumination time, the supramolecular assembly emitted multicolor fluorescence in aqueous solutions and solid films. It even enabled white light imaging in living cells, providing new information processing and biological imaging strategies. Moreover, upon the coordination of azobenzene pyridine dicarboxylic acid and Eu3+, it can further be assembled with acrylamide-modified α-CD to obtain a photo-responsive supramolecular hydrogel through free-radical copolymerization (Fig. 20b).76 Owing to the host–guest complexation and synergistic metal coordination, the supramolecular hydrogel exhibited photo-switchable luminescence behavior and excellent fatigue resistance, accompanied by enhanced mechanical strength.
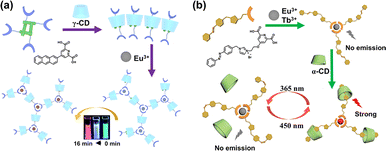 |
| Fig. 20 (a) Schematic illustration of a ternary supramolecular assembly composed of γ-CD, anthracene, and Eu3+ for application in photochromic multicolor fluorescence emission. This figure has been adapted from ref. 75 with permission from the authors, copyright 2019. (b) Schematic illustration of a CD-confined supramolecular lanthanide photoswitch based on azobenzene pyridine dicarboxylic acid, α-CD, and Ln3+. This figure has been adapted from ref. 76 with permission from Wiley-VCH GmbH, copyright 2022. | |
6. Conclusions and outlook
In conclusion, we have mainly summarized the research progress of supramolecular self-assembly luminescent materials based on CD monomers, CD derivatives, and CD-based polymers. Benefiting from their hydrophobic cavities, CDs can encapsulate size-matching guest luminophores and special functional groups to construct stable water-soluble host–guest complexes, inducing or enhancing fluorescence and phosphorescence emission through the macrocyclic spatial confinement effect. Possessing multiple hydroxyl groups, CDs provide favorable conditions for the formation of hydrogen bonds and the modification of fluorescent/phosphorescent chromophores or stimulus-responsive groups, achieving efficient solid-state supramolecular phosphorescence, stimulus-responsive intelligent luminescence, and supramolecular fluorescent probes. Multicharged CDs enhance molecular recognition and introduce new driving forces for macrocyclic self-assembly; they can be co-assembled with fluorescent luminophores that carry opposite charges through electrostatic interactions, leading to the creation of supramolecular AIE systems, fluorescent light-harvesting systems, and enabling the topological morphology control of multi-level supramolecular assemblies. CD-based polymers, like HACD, serve as cascaded media to co-assemble with other macrocyclic molecules, which not only effectively improves the luminescence behavior of guest molecules and enables efficient PRET for NIR persistent luminescence bioimaging but also significantly improves the biocompatibility of the assembly, endowing it with the ability to target cancer cells. In addition, CD-threaded polymers, which form polyrotaxane and pseudopolyrotaxane, have been effectively utilized for constructing phosphorescent supramolecular soft materials and mechano-responsive luminescent materials. CD-MOFs and lanthanide metal coordination assemblies can effectively induce CPL emission from guest chromophores, regulate the luminescence behavior of rare earth elements, and achieve tunable multicolor luminescence. This review highlights the widespread applications of CD-based supramolecular luminescent systems in cell imaging, sensing, flexible devices, anti-counterfeiting, and information encryption.
Unlike molecular aggregation and directional assembly achieved through hydrogen bonding, electrostatic interaction, and π–π stacking, CD-confined assembly primarily depends on molecular recognition and strong binding affinity to induce or promote guest luminescence. This CD-confinement effect should also be applicable to crown ethers, cucurbiturils, calixarenes, and other macrocyclic compounds. With the continuous emergence of functional chromophores and CD host primitives, as well as the construction of functional assemblies, the supramolecular assemblies utilizing the CD-confinement effect will find broad applications in biological diagnosis and treatment, chemical recognition and reactions, and luminescent materials, which will surely promote the development of supramolecular chemistry.
Author contributions
X. Z. searched the literature and wrote the manuscript. Y. L. and H. Z. edited and modified the manuscript.
Conflicts of interest
There are no conflicts to declare.
Acknowledgements
We thank the National Natural Science Foundation of China (NNSFC, Grant No. 22131008), China Fundamental Research Funds for the Central Universities, and the Haihe Laboratory of Sustainable Chemical Transformations for financial support.
Notes and references
- X.-Y. Ma and Y. Liu, Acc. Chem. Res., 2021, 54, 3403–3414 CrossRef PubMed.
- D. H. Qu, Q. C. Wang, Q. W. Zhang, X. Ma and H. Tian, Chem. Rev., 2015, 115, 7543–7588 CrossRef PubMed.
- D. Y. Xia, P. Wang, X. F. Ji, N. M. Khashab, J. L. Sessler and F. H. Huang, Chem. Rev., 2020, 120, 6070–6123 Search PubMed.
- X.-Y. Lou and Y.-W. Yang, Adv. Opt. Mater., 2018, 6, 1800668 CrossRef.
- X.-Y. Lou and Y.-W. Yang, Aggregate, 2020, 1, 19–30 CrossRef.
- Y. Chen, F. Huang, Z.-T. Li and Y. Liu, Sci. China: Chem., 2018, 61, 979–992 CrossRef.
- J. F. Feng, M. Tan, S. Zhang and B. J. Li, Macromol. Rapid Commun., 2021, 42, 2100497 CrossRef PubMed.
- Y. Shepelytskyi, C. J. Newman, V. Grynko, L. E. Seveney, B. DeBoef, F. T. Hane and M. S. Albert, Molecules, 2020, 25, 5576 CrossRef PubMed.
- D. A. Real, K. Bolaños, J. Priotti, N. Yutronic, M. J. Kogan, R. Sierpe and O. Donoso-González, Pharmaceutics, 2021, 13, 2131 CrossRef CAS PubMed.
- S. B. Hong, M. Y. Liu, W. Zhang and W. Deng, Chin. J. Org. Chem., 2015, 35, 325–336 CrossRef.
- S. Y. Liu, J. X. Zheng, J. Q. Wang, S. H. Liu, X. L. Zhang, D. Bao and P. Zhang, Gels, 2023, 9, 854 CrossRef PubMed.
- K. Ito, Chem. Pharm. Bull., 2017, 65, 326–329 Search PubMed.
- Y. Q. Zhao, Z. Zheng, C. Y. Yu and H. Wei, J. Mater. Chem. B, 2023, 12, 39–63 RSC.
- J. Wang, Z. Q. Qiu, Y. M. Wang, L. Li, X. H. Guo, D. T. Pham, S. F. Lincoln and R. K. Prud'homme, Beilstein J. Org. Chem., 2016, 12, 50–72 Search PubMed.
- Y. Tsuchido, S. Fujiwara, T. Hashimoto and T. Hayashita, Chem. Pharm. Bull., 2017, 65, 318–325 Search PubMed.
- Y. Xu, A. K. Rashwan, A. I. Osman, E. M. Abd El-Monaem, A. M. Elgarahy, A. S. Eltaweil, M. Omar, Y. T. Li, A. H. E. Mehanni, W. Chen and D. W. Rooney, Environ. Chem. Lett., 2023, 21, 447–477 CrossRef PubMed.
- R. I. Khan and K. Pitchumani, RSC Adv., 2016, 6, 20269–20275 RSC.
- T. Gu, H. Li, T. Wu, X. Li, H. Li, H. Zhao, Y. Xiao, J. Huang and Y. Yan, Adv. Funct. Mater., 2024, 34, 2311864 CrossRef.
- Q.-W. Zhang, D. Li, X. Li, P. B. White, J. Mecinović, X. Ma, H. Ågren, R. J. M. Nolte and H. Tian, J. Am. Chem. Soc., 2016, 138, 13541–13550 CrossRef PubMed.
- H. Shigemitsu, K. Kawakami, Y. Nagata, R. Kajiwara, S. Yamada, T. Mori and T. Kida, Angew. Chem., Int. Ed., 2022, 61, e202114700 CrossRef PubMed.
- C. Tu, W. Wu, W. Liang, D. Zhang, W. Xu, S. Wan, W. Lu and C. Yang, Angew. Chem., Int. Ed., 2022, 61, e202203541 Search PubMed.
- Q. Li, X. Wang, Q. Huang, Z. Li, B. Z. Tang and S. Mao, Nat. Commun., 2023, 14, 409 CrossRef PubMed.
- G. Singh and P. K. Singh, Langmuir, 2019, 35, 14628–14638 CrossRef PubMed.
- J.-J. Li, Y. Chen, J. Yu, N. Cheng and Y. Liu, Adv. Mater., 2017, 29, 1701905 CrossRef PubMed.
- J.-J. Li, H.-Y. Zhang, G. Liu, X. Dai, L. Chen and Y. Liu, Adv. Opt. Mater., 2021, 9, 2001702 CrossRef CAS.
- N. J. Turro, J. D. Bolt, Y. Kuroda and I. Tabushi, Photochem. Photobiol., 1982, 35, 69–72 CrossRef.
- D. Li, Z. Liu, M. Fang, J. Yang, B. Z. Tang and Z. Li, ACS Nano, 2023, 17, 12895–12902 CrossRef PubMed.
- X. Zhou, X. Zhao, X. Bai, Q. Cheng and Y. Liu, Adv. Funct. Mater., 2024, 14, 2400898 CrossRef.
- D. Li, F. Lu, J. Wang, W. Hu, X.-M. Cao, X. Ma and H. Tian, J. Am. Chem. Soc., 2018, 140, 1916–1923 CrossRef PubMed.
- C. Zhao, Y. Jin, J. Wang, X. Cao, X. Ma and H. Tian, Chem. Commun., 2019, 55, 5355–5358 RSC.
- Z.-Y. Zhang, W.-W. Xu, W.-S. Xu, J. Niu, X.-H. Sun and Y. Liu, Angew. Chem., Int. Ed., 2020, 59, 18748–18754 CrossRef PubMed.
- J. Wang, X.-Y. Lou, J. Tang and Y.-W. Yang, J. Polym. Sci., 2023, 61, 903 Search PubMed.
- Y. Sun, Y. Chen, L. Jiang, X. Yu, Y. Qin, S. Wang and Y. Liu, Adv. Opt. Mater., 2022, 10, 2201330 CrossRef.
- H. Chen, L. Xu, X. Ma and H. Tian, Polym. Chem., 2016, 7, 3989–3992 RSC.
- X.-K. Ma, Q. Cheng, X. Zhou and Y. Liu, JACS Au, 2023, 3, 2036–2043 CrossRef CAS PubMed.
- Y.-Y. Hu, X.-Y. Dai, X. Dong, M. Huo and Y. Liu, Angew. Chem., Int. Ed., 2022, 61, e202213097 CrossRef PubMed.
- Y. M. Zhang, Y. H. Liu and Y. Liu, Adv. Mater., 2020, 32, 1806158 CrossRef PubMed.
- Z.-Y. Li, Y. Chen, H. Wu and Y. Liu, ChemistrySelect, 2018, 3, 3203–3207 CrossRef.
- H.-L. Sun, Y. Chen, J. Zhao and Y. Liu, Angew. Chem., Int. Ed., 2015, 54, 9376–9380 CrossRef PubMed.
- X. Ma, J. Cao, Q. Wang and H. Tian, Chem. Commun., 2011, 47, 3559–3561 RSC.
- J. Cao, X. Ma, M. Min, T. Cao, S. Wu and H. Tian, Chem. Commun., 2014, 50, 3224–3226 RSC.
- H.-J. Wang, H.-Y. Zhang, C. Zhang, B. Zhang, X. Dai, X. Xu and Y. Liu, ACS Appl. Polym. Mater., 2022, 4, 2935–2940 CrossRef.
- L. Chen, Y. Chen, H.-G. Fu and Y. Liu, Adv. Sci., 2020, 7, 2000803 CrossRef CAS PubMed.
- X. Lin, Q. Xu and X. Ma, Adv. Opt. Mater., 2022, 10, 2101646 CrossRef CAS.
- Z. Liu, M. Tian, H. Zhang and Y. Liu, Chem. Commun., 2023, 59, 896–899 RSC.
- Z. Liu, X. Dai, Q. Xu, X. Sun and Y. Liu, Chin. J. Chem., 2022, 40, 493–499 CrossRef.
- F.-F. Shen, Y. Chen, X. Dai, H.-Y. Zhang, B. Zhang, Y. Liu and Y. Liu, Chem. Sci., 2021, 12, 1851–1857 RSC.
- J. Zhao, Y.-M. Zhang, H.-L. Sun, X.-Y. Chang and Y. Liu, Chem.–Eur. J., 2014, 20, 15108–15115 CrossRef PubMed.
- M. Tian, Z. Wang, X. Yuan, H. Zhang, Z. Liu and Y. Liu, Adv. Funct. Mater., 2023, 33, 2300779 Search PubMed.
- R. Du, Q. Jin, T. Zhu, C. Wang, S. Li, Y. Li, X. Huang, Y. Jiang, W. Li, T. Bao, P. Cao, L. Pan, X. Chen, Q. Zhang and X. Jia, Small, 2022, 18, 2200533 CrossRef PubMed.
- A. Hashidzume, H. Yamaguchi and A. Harada, Eur. J. Org Chem., 2019, 2019, 3344–3357 CrossRef.
- G. Wenz, B.-H. Han and A. Müller, Chem. Rev., 2006, 106, 782–817 CrossRef PubMed.
- Y. Zhang, Y. Chen, H. Zhang, L. Chen, Q. Bo and Y. Liu, Adv. Opt. Mater., 2023, 11, 2202828 CrossRef.
- J.-J. Li, H.-Y. Zhang, Y. Zhang, W.-L. Zhou and Y. Liu, Adv. Opt. Mater., 2019, 7, 1900589 CrossRef.
- Y. Zhang, C. Zhang, Y. Chen, J. Yu, L. Chen, H. Zhang, X. Xu and Y. Liu, Adv. Opt. Mater., 2022, 10, 2102169 Search PubMed.
- C. Liu, N. Morimoto, L. Jiang, S. Kawahara, T. Noritomi, H. Yokoyama, K. Mayumi and K. Ito, Science, 2021, 372, 1078–1081 Search PubMed.
- Y. Zhang, Y. Chen, J.-Q. Li, S.-E. Liu and Y. Liu, Adv. Sci., 2024, 11, 2307777 CrossRef PubMed.
- F. Seidi, Y. Jin and H. Xiao, Carbohydr. Polym., 2020, 242, 116277 CrossRef PubMed.
- Q. Zhao and Y. Liu, Chem. Commun., 2018, 54, 6068–6071 RSC.
- Y. Gao, G. Li, Z. Zhou, L. Gao and Q. Tao, Int. J. Biol. Macromol., 2017, 105, 74–80 CrossRef PubMed.
- H. Chen, X. Ma, S. Wu and H. Tian, Angew. Chem., Int. Ed., 2014, 53, 14149–14152 CrossRef PubMed.
- Q. Cheng, X.-K. Ma, X. Zhou, Y.-M. Zhang and Y. Liu, Small, 2024, 20, 2309732 CrossRef PubMed.
- Y. Yang, Y.-J. Jin, X. Jia, S.-K. Lu, Z.-R. Fu, Y.-X. Liu and Y. Liu, ACS Med. Chem. Lett., 2020, 11, 451–456 CrossRef CAS PubMed.
- X. Zhou, X. Bai, X. Zhang, J. Wu and Y. Liu, Adv. Opt. Mater., 2024, 12, 2301550 CrossRef CAS.
- X.-Y. Dai, M. Huo, X. Dong, Y.-Y. Hu and Y. Liu, Adv. Mater., 2022, 34, 2203534 CrossRef CAS PubMed.
- J. Yu, J. Niu, J. Yue, L.-H. Wang and Y. Liu, ACS Nano, 2023, 17, 19349–19358 CrossRef CAS PubMed.
- X. Zhou, X. Bai, F. Shang, H.-Y. Zhang, L.-H. Wang, X. Xu and Y. Liu, Nat. Commun., 2024, 15, 4787 CrossRef CAS.
- R. A. Smaldone, R. S. Forgan, H. Furukawa, J. J. Gassensmith, A. M. Z. Slawin, O. M. Yaghi and J. F. Stoddart, Angew. Chem., Int. Ed., 2010, 49, 8630–8634 Search PubMed.
- R. S. Forgan, R. A. Smaldone, J. J. Gassensmith, H. Furukawa, D. B. Cordes, Q. Li, C. E. Wilmer, Y. Y. Botros, R. Q. Snurr, A. M. Z. Slawin and J. F. Stoddart, J. Am. Chem. Soc., 2012, 134, 406–417 CrossRef CAS PubMed.
- L. Hu, K. Li, W. Shang, X. Zhu and M. Liu, Angew. Chem., Int. Ed., 2020, 59, 4953–4958 CrossRef CAS PubMed.
- Y. Chen, B. Yu, Y. Cui, S. Xu and J. Gong, Chem. Mater., 2019, 31, 1289–1295 Search PubMed.
- N. Cheng, Y. Chen, J. Yu, J.-j. Li and Y. Liu, Bioconjugate Chem., 2018, 29, 1829–1833 Search PubMed.
- Z. Kotková, L. Helm, J. Kotek, P. Hermann and I. Lukeš, Dalton Trans., 2012, 41, 13509–13519 RSC.
- M. Zhao, B. Li, P. Wang, L. Lu, Z. Zhang, L. Liu, S. Wang, D. Li, R. Wang and F. Zhang, Adv. Mater., 2018, 30, 1804982 CrossRef PubMed.
- W. Zhou, Y. Chen, Q. Yu, P. Li, X. Chen and Y. Liu, Chem. Sci., 2019, 10, 3346–3352 RSC.
- H.-J. Yu, H. Wang, F.-F. Shen, F.-Q. Li, Y.-M. Zhang, X. Xu and Y. Liu, Small, 2022, 18, 2201737 CrossRef PubMed.
|
This journal is © The Royal Society of Chemistry 2024 |
Click here to see how this site uses Cookies. View our privacy policy here.