Thermochromic and conductive hydrogels with tunable temperature sensitivity for dual sensing of temperature and human motion†
Received
15th September 2023
, Accepted 4th December 2023
First published on 5th December 2023
Abstract
Skin-like body sensors have important applications in soft-bodied intelligent robots, electronic skins, and medical health monitoring. Accurate sensing of pressure and temperature are two core elements of skin-like sensors that mimic skins. Skin-like sensors convert sensed external stimuli such as pressure and temperature into electrical signals, enabling visual monitoring of external environmental stimuli. However, there are very limited reports on hydrogel-based and dual stimuli-responsive (strain/temperature) sensors. To this end, we report a highly flexible strain/temperature dual stimuli-responsive sensor based on poly(acrylamide) (PAAM) hydrogels. Our prepared conductive ionic hydrogels are endowed with the ability to undergo phase changes at different temperatures by introducing inexpensive and stable sodium dodecyl sulfate (SDS) thermotropic phase-change units, which can turn from opaque to transparent within 10 s when temperature is increased, and to opaque again when temperature is decreased. In this study, we have succeeded in producing a dual-responsive multifunctional ionic conductive hydrogel (SN-PAAM) that is responsive to temperature/strain dual stimuli and has excellent mechanical properties: high tensile strength (581 kPa), extra long elongation (1836%), high sensitivity (GF = 1.62 to 2.46), and short response time (120 to 130 ms). To the best of our knowledge, these combined functions are not achievable with conventional strategies. Therefore, the hydrogels developed in this research could have good prospects for different applications such as flexible wearable devices, skin-like sensors, visualization of bionic skins, dual response interactive devices, and in the field of anti-counterfeiting and encryption.
Introduction
In recent years, skin-like sensors have attracted widespread interest from researchers as they have important applications in soft-bodied intelligent robots, electronic skins, and medical health monitoring.1–5 Skin is not only the largest organ in a human body but also plays a major role in how we perceive our external environment.6 Accurate sensing of pressure and temperature are two core functions of skin-like sensors that mimic skins. Skin-like sensors convert sensed external stimuli such as pressure and temperature into electrical signals, enabling visual monitoring of external environmental stimuli.7–10 Traditional rigid sensors are less flexible and stretchable, making them limited in wearable electronics. Hydrogels are soft, stretchable, and biocompatible, making them promising flexible and stretchable sensors for smart wearable electronic devices.11–13 Ion-conductive hydrogels are highly conductive due to their unique porous structure, which provides efficient channels for ion transport.14,15 The ion-conductive hydrogels are also highly elastic, highly transparent, and biocompatible, which allows them to be used as flexible strain sensors with a large strain range and high sensitivity.16–19 By designing a network structure with various physical cross-linkages such as hydrophobic association between polyacrylic acid (PAA) polymer chains, metal ion coordination, and chain entanglement, Xin et al.16 have produced a strong, stretchable, self-healing, and freeze-resistant ion-conductive hydrogel. The physical network of chain entanglement caused by the salting effect enhances the mechanical strength and promotes electron transport after the addition of NaCl. This study provides new insights into the design of multifunctional ionic skins for wearable electronic devices. Wu et al.14 developed a comprehensive conductive hydrogel combining high mechanical stability, strong adhesion and excellent mechanical sensitivity. It consists of tannic acid@bacterial cellulose (TA@BC), acrylic acid (AA), and 2-acrylamide-2-methylpropanesulfonic acid (AMPS). They form the backbone of a double network by introducing high-modulus bacterial cellulose (BC) and covering BC with tannic acid (TA), improving its uniform distribution in the hydrogel. And the natural polyphenol TA forms hydrogen bonds with the polymer to stiffen the polymer network. This versatile ionic conductive hydrogel, which exhibited excellent mechanical properties in both tensile (83.4 kPa, 1235.9% strain) and compressive (207.2 kPa, 79.9% strain) stress–strain tests, offers a new strategy for the development of next-generation smart materials. Wang et al.20 have developed a new strategy by combining polyacrylamide-grafted gelatine (PAAM/GE) hydrogels with polyurethane (PU) electro-spun fibre membranes in a glycerol–water binary solvent system, a novel fibre-reinforced organo-hydrogel was developed. The organo-hydrogel exhibited excellent mechanical properties (stress up to 3.09 MPa and strain up to 614%), frost resistance (40 °C), and high ionic conductivity at room temperature (1.51 S m−1) due to the synergistic effect of the PU electro-spun fibre membrane and the PAAM/GE hydrogel. The prepared organic hydrogels also show excellent sensitivity, stability and reproducibility in detection of human motion. However, these skin-like sensors can only detect strain or pressure, neglecting the monitoring of ambient temperature stimulus.
Real-time sensing of ambient temperature is another key function of flexible wearable sensors.21 However, there are very limited reports on hydrogel-based dual stimuli-responsive (strain/temperature) sensors. To this end, we report a highly flexible strain/temperature dual stimuli-responsive sensor based on sodium dodecyl sulcate (SDS)-incorporated PAAM hydrogels. A facile method is proposed to fabricate a dual stimuli-responsive ion-conductive hydrogel (SN-PAAM) with multifunctional properties based on acrylamide (AAM), SDS, lauryl methacrylate (LMA), and sodium chloride (NaCl). With good mechanical properties, excellent electrical conductivity and dynamic visual display capabilities, the most attractive feature of the SN-PAAM ionic hydrogels is their dual response to strain and temperature. Our conductive ionic hydrogels have the ability to undergo phase transitions at different temperatures by introducing inexpensive and stable SDS thermotropic phase-change units, which can change from opaque to transparent within 10 s when temperature is increased, and to opaque again when temperature is decreased. The SN-PAAM hydrogels also exhibit good response to different strains and temperatures and transmit change information through changes in relative resistance. The SN-PAAM hydrogels have a wide strain sensing range (1–1000%) and good sensing capability over a wide temperature range. The transparent–opaque phase transition also allows it to be used in applications such as anti-counterfeit encryption, providing new ideas for anti-counterfeit and encryption. SN-PAAM organic hydrogel strain sensors exhibit excellent sensitivity and response time over a wide operating range. The GF of the organic hydrogel is stable at 1.62 over the strain range of 0–200%, while it rises to 2.46 with strains greater than 200%. during loading–unloading, the SN-PAAM organic hydrogel has a fast response time as short as 120 ms and a recovery time of 130 ms, which is similar to the response time of human skins (∼100 ms).4 To the best of our knowledge, these combined functions are not achievable with conventional strategies (previous studies summarized in Table S1, ESI†). In summary, our SN-PAAM ion-conductive hydrogel design strategy promises to be a new generation of multi-stimuli-responsive hydrogels for visualized bionic skin, interactive wearables, anti-counterfeiting, and encryption.
Materials and methods
Materials
Acrylamide (AAM, 99.0%), sodium dodecyl sulcate (SDS, 97%), potassium persulfate (KPS, 99.5%), N,N′-methylenebisacrylamide (MBAA, 98%), lauryl methacrylate (LMA, 95%) were supplied by General-reagent® (Shanghai, China). N,N,N,N′-tetramethylethylenediamine (TEMED, 99.5%) was supplied by Adamas (Shanghai, China). Sodium chloride (NaCl) was supplied by Aladdin (Shanghai, China). All chemicals were used without further purification. Deionized water was used throughout the experiments.
Preparation of hydrogels
Preparation of AAM hydrogels.
2 g of AAM was added to 20 mL of deionized water and stirred at room temperature until the system was both clear and transparent. Then 0.06 g of MBAA, 0.04 g of KPS and 65 μL of TMEDA were added and stirred. Finally, the sample was loaded into a mold and placed in an oven at 35 °C for 3 hours to obtain the hydrogel.
Preparation of hydrophobic-conjugated thermotropic hydrogels.
Thermotropic phase change hydrogels were obtained by a free radical polymerization mechanism. The procedure was as follows: 1 g of SDS and 65 μL of LMA were added to 20 mL of deionized water, stirred at room temperature until clear and transparent, then different masses of NaCl (0.3, 0.6, 0.9, and 1.2 g) were added. After stirring for 0.5 h at room temperature, 2 g of AAM was added and stirred for 1 h. Finally, 0.04 g of KPS and 35 μL of TMEDA were added into the molds and placed in an oven at 35 °C for 3 h to obtain the hydrogel (SN-PAAM) samples.
Microstructural observation of the hydrogels
Cross-sectional structure of the PAAM and SN-PAAM hydrogels was observed using a scanning electron microscope (SEM) (Thermo Scientific™, USA), the samples were dried in a freeze-dryer for 48 hours and the dried samples were coated with palladium on a sputter coater and observed by SEM at an accelerating voltage of 10 kV.
Measurements and testing of structure and properties
Detailed description of transparency testing, FT-IR measurements, rheological testing, measurement of mechanical properties of the hydrogels, and performance testing of hydrogel sensors are provided in the ESI.†
Results and discussion
Design strategy and characterization of SN-PAAM hydrogels
In order to construct a dual stimuli-responsive hydrogel, we introduced an anionic surfactant, SDS, with an adjustable critical solubility temperature (Krafft point, Tk) into the ionic hydrogel,22,23 making the hydrogel dual responsive to temperature and strain. Our ionic hydrogels were prepared using a one-pot method, as shown in Fig. 1, where the SN-PAAM hydrogels were obtained by a free radical polymerization mechanism. SDS, LMA, and NaCl were first stirred in deionized water until the system was clear and transparent, then AAM, crosslinker and initiator were added and stirred to obtain the hydrogel precursor solution. Finally, the precursor solution was loaded into a mold and placed in an oven for 3 hours to obtain a sample of the hydrogel (SN-PAAM). In this work, PAAM was used as the framework structure with hydrophobic LMA to form the main structure of the hydrogel, which is the hydrophobic associative microregion, and the anionic surfactant SDS combined with NaCl to form the hydrogel micelles, which acted as the hydrophobic associative centre of the SN-PAAM hydrogel.24–26 Miraculously, we found that by varying the amount of NaCl, not only could the mechanical properties of the SN-PAAM hydrogel be adjusted, but the thermotropic phase transition temperature of the hydrogel would also change regularly. The PAAM-LMA micro-region (hydrophobic associative micro-region) in the SN-PAAM hydrogel is partially decoupled by external forces to dissipate energy, and when the external forces are removed, the hydrophobic associative micro-region is re-formed and the network structure is restored. When the external force is removed, the hydrophobic zones re-form and restore the network structure. This dynamic physical structure provides an effective energy dissipation mechanism for the hydrogel and enhances the mechanical strength of the SN-PAAM hydrogel. The thermo-responsive unit SDS-NaCl is the basis for the formation of hydrogel micelles, and the temperature-driven thermogenic phase change capability of SN-PAAM hydrogels is achieved by varying SDS-NaCl to modulate micelle size.27–31 In contrast, the inorganic salt NaCl in the hydrogel network has two main functions, the first of which is to reduce the critical micelle concentration (CMC) of SDS.31 The cation in the salt interacts with the –SO42− head group of SDS in the hydrophobic layer, reducing electrostatic repulsion and leading to a reduction in CMC. The other function is that NaCl ions can diffuse or move freely in the porous hydrogel network, providing excellent the electrical conductivity to the hydrogel.30
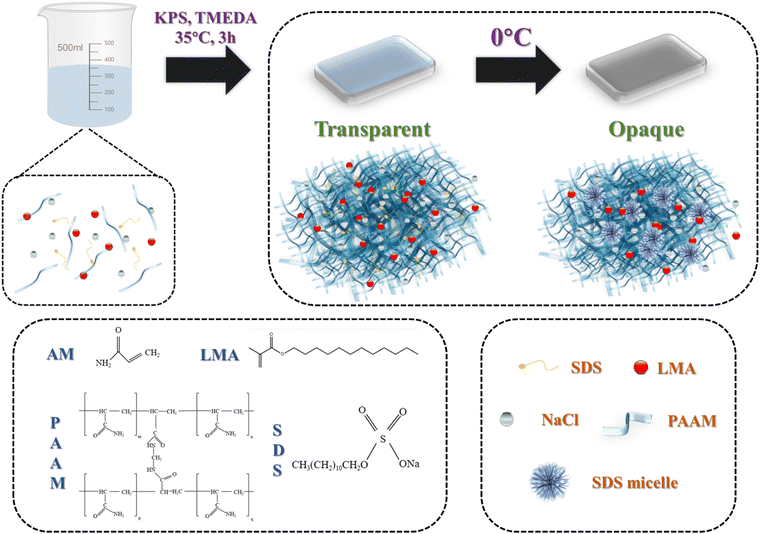 |
| Fig. 1 Structure and formation mechanism of photochromic hydrogels. | |
The morphology and microstructure of pure PAAM hydrogel and SN-PAAM hydrogel (in the opaque state) were characterized using scanning electron microscopy (SEM). As shown in Fig. 2(a)–(c), the original PAAM hydrogel exhibited a uniform morphology with a three-dimensional structure and a smooth surface. However, the hydrogel with SDS and NaCl added showed a relatively compact three-dimensional structure. Furthermore, the surface of the SN-PAAM hydrogel became rough and uneven due to the presence of 500-nanometer diameter SDS micelles and aggregated inorganic particles.29,32 This is also the reason why SN-PAAM exhibits thermal-responsive properties. Additionally, we tested the optical transparency of pure PAAM hydrogel and SN-PAAM hydrogel (with 4% NaCl content, opaque at 20 °C) at room temperature (20 °C) using a UV spectrophotometer. As shown in Fig. 2(f), at a wavelength of 400 nm, the PAAM hydrogel had the highest visible light transmittance, reaching nearly 90%. On the other hand, the SN-PAAM hydrogel became opaque due to the aggregation of SDS-NaCl micelles, with only 5% visible light transmittance at a wavelength of 400 nm.
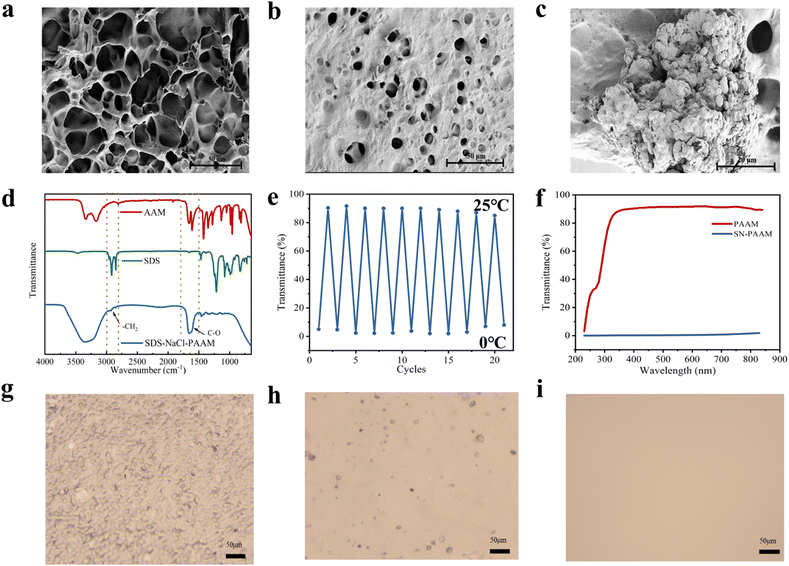 |
| Fig. 2 (a)–(c) SEM images of SN-PAAM hydrogels without/with NaCl. (d) FT-IR spectra of hydrogels. (e) Cycling tests of thermochromic SN-PAAM hydrogels. (f) Transmittance curves of SN-PAAM hydrogels without/with NaCl at room temperature (25 °C). (g)–(i) Optical micrographs of SN-PAAM hydrogel at room temperature (25 °C) after different period of time ((g) 0 min, (h) 2 min, and (i) 3 min). | |
In addition, the composition and structure of the SN-PAAM hydrogel were determined. Fig. 2(d) shows the FT-IR spectra of AAM, SDS, NaCl, and SN-PAAM hydrogel. For the AAM spectrum, the characteristic absorption peaks at 3415 cm−1, 3159 cm−1, 1670 cm−1, 1602 cm−1, and 1433 cm−1 correspond to the free –NH–, the coordinated –NH–, the C
O stretching vibration, the N–H bending vibration, and the methylene deformation, respectively.30,31 For the surfactant SDS spectrum, the characteristic absorption peaks at 2916 cm−1, 2852 cm−1, and 1218 cm−1 correspond to the asymmetric –CH2 stretching vibration, the symmetric –CH2 stretching vibration, and the S
O stretching vibration, respectively.29–31 In the SN-PAAM hydrogel, the broad and strong absorption band at 3322 cm−1 is assigned to the N–H stretching of –NH2 and the stretching vibration of O–H (from structural water). The small double peaks in the range of 2952–2852 cm−1 are caused by the asymmetric and symmetric vibrations of –CH2 from both SDS molecules and the polymer skeleton (PAAM).32
The strong and narrow C–O stretching band33 of the AAM group can be observed at 1660 cm−1. The S
O stretching from SDS remains in the same position, indicating that the SDS micelles are physically distributed within the matrix. Furthermore, we compared the FT-IR spectra of SN-PAAM hydrogel in transparent and opaque states and found them to be almost identical, indicating that there was no chemical reaction in the gel network during the thermal phase transition.
Mechanical properties of SN-PAAM organic hydrogels
The mechanical properties of the SN-PAAM hydrogels are excellent due to the presence of hydrophobic interactions in the SN-PAAM by the process of conjoining–reconjoining. We evaluated the tensile strength and elongation behaviour of hydrogel samples with different NaCl (0–4%) contents by tensile experiments. Fig. 3(a) shows the stress–strain curves of SN-PAAM hydrogels with different NaCl contents, while Fig. 3(b) shows the toughness and elastic modulus of SN-PAAM hydrogels with different NaCl contents. When NaCl was introduced into the PAAM hydrogel system, with increase of NaCl content, the SN-PAAM hydrogels were enhanced in both strain and stress. The SN-PAAM (NaCl = 4%) hydrogels showed high tensile strength of 581 kPa, high elastic modulus of 29.19 kPa, ultra-long elongation of 1836%, and 479.67 kJ m−3 of excellent toughness; all the values were much higher than those of the pure PAAM hydrogels (67 kPa, 8.44 kPa, 665%, and 244.6 kJ m−3, respectively). This is mainly due to the existence of mechanisms for effective energy dissipation in the internal network of the hydrogels, which is PAAM-LMA hydrophobic association micro-regions and SDS-NaCl hydrophobic association centres.24,25 The addition of NaCl facilitates the formation of large size SDS micelles, which can increase the solubility of hydrophobic monomers.31,32 And with the increase of NaCl content, the hydrogen bonding interaction between the hydrogel backbone is enhanced and the mechanical properties increase to a certain extent. The elongation at break of the SN-PAAM hydrogels reached a maximum of 1900% when the NaCl content was 3.0%, while the elongation at break of the SN-PAAM hydrogels decreased to 1836% when the NaCl content was 4.0% and the tensile strength increased slightly; both the elongation at break and the tensile strength started to decrease when the NaCl content was 5.0%.
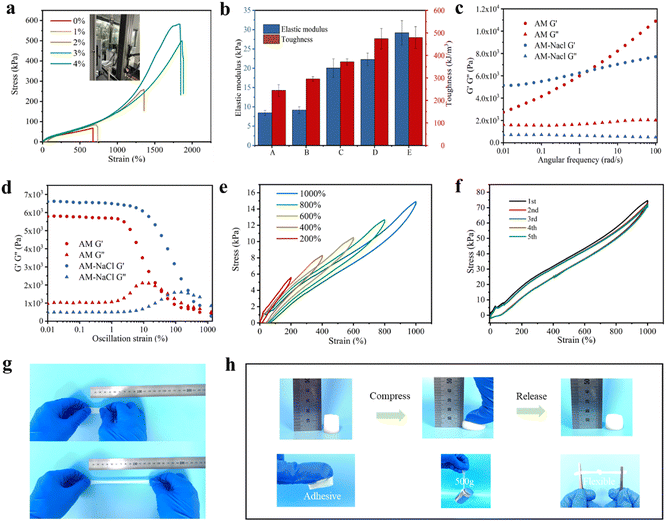 |
| Fig. 3 (a) and (b) Tensile stress–strain curves and corresponding toughness and modulus of elasticity of SN-PAAM hydrogels with different NaCl contents. (c) Dynamic rheological curves of SN-PAAM under different strains. (d) Dynamic rheological curves of SN-PAAM under different frequency scans. (e) Continuous cyclic loading–unloading curves of SN-PAAM hydrogels. (f) Continuous tensile load–unload cycle curves of SN-PAAM hydrogels. (g) Photograph of SN-PAAM hydrogels stretched 18 times. (h) Pictures showing the compressibility, flexibility, and adhesion of organic hydrogels. | |
The main reason for this is that when NaCl is added at a low level, it can have a certain toughening effect, reaching its optimum at 4%. However, as the NaCl content continues to increase to 6.0% (Fig. S1, ESI†), the network structure of the hydrogel and the cross-linked network will be destroyed from these defects during the tensile process due to the high content of NaCl, resulting in a significant decrease in mechanical properties.24,26 As shown in Fig. 3(g) and (h), the SN-PAAM hydrogels can withstand large, twisted, and crossed stretches. Moreover, the SN-PAAM hydrogels can also withstand large compression and loads of 500 g with no apparent damage, indicating that the SN-PAAM hydrogels have superior tensile and compressive properties. In addition, the SN-PAAM hydrogels have a slight adhesive property that allows them to be adhered to a hand for sensing activities.
Further, we have performed dynamic rheological property measurements on SN-PAAM hydrogels with different NaCl contents to better illustrate the effect of NaCl content on the mechanical properties of the hydrogels. The curves of the energy storage modulus G′ and loss modulus G′′ of pure PAAM hydrogels and SN-PAAM hydrogels with angular frequency are shown in Fig. 3(c). It can be seen that the dynamic energy storage modulus (G′) is higher than the loss modulus (G′′) over the entire frequency sweep for each group of hydrogel samples, demonstrating the elastomeric nature of the hydrogel.34 The storage modulus G′ and loss modulus G′′ of the SN-PAAM hydrogels were relatively independent of frequency over the entire range, indicating the formation of gel-like networks.24 Moreover, the storage modulus G′ of the SN-PAAM hydrogels was significantly higher, indicating a denser and stronger network structure after the introduction of SDS and NaCl to construct hydrophobic linkage networks. From a microscopic rheological point of view, the mechanical properties of the hydrophobically linked hydrogels were significantly improved, which corroborated with the results obtained from the tensile tests. Oscillatory strain amplitude scan tests were performed on both PAAM and SN-PAAM hydrogels. As shown in Fig. 3(d), the hydrogels exhibited strain-dependent viscoelastic response behaviour. In the linear region (less than the critical strain), G′ is always higher than G′′, indicating that the hydrogels exhibit an elastic (gel-like) behaviour. However, as the shear strain increases above the critical strain, G′ gradually decreases, while G′′ gradually increases. When the shear strain increased further, G′′ exceeded G′, indicating the transition behaviour of the hydrogel from solid (gel) to liquid (viscous fluid).24 the phase intersection strain of the SN-PAAM hydrogel was 363.36%, while that of the PAAM hydrogel was only 56.03%. This indicates that the stability of the hydrogels was improved with the introduction of the hydrophobic linkage network.35
To further analyse the mechanical properties of the hydrogels, a series of tensile loading–unloading cycles were performed on SN-PAAM hydrogels to analyse the stress changes during the tensile process in order to investigate the toughening mechanism. Fig. 3(e) illustrates the loading–unloading curves of the continuous tensile tests at different strains. As the strain increases from 200% to 1000%, both the tensile stress of the hydrogel and the area of the hysteresis loop increase. This is due to the fact that the reversible conjoining–reconjoining process effectively dissipates energy during the stretching process, resulting in higher tensile toughness.4 Furthermore, Fig. 3(f) shows the hydrogel stretching profile for five consecutive cycles without any residence time. It can be noted that, in the first cycle, there is a hysteresis cycle; while in the subsequent four cycles, the hysteresis effect is smaller than that in the first cycle. This indicates that the hydrogel exhibits good self-recovery through the reversible hydrophobic interaction of the conjoining–reconjoining processes.24
Thermogenic phase change properties of SN-PAAM hydrogels
The transition between two states (opaque–transparent) of the SN-PAAM hydrogels can be completed in less than 10 seconds, which is much faster than many other thermochromic techniques. Fig. 4(a)–(e) shows the light transmission profiles of SN-PAAM at different NaCl contents from 5 °C to 25 °C. At a wavelength of 400 nm, all hydrogels with 0–4% NaCl contents exhibit over 75% light transmission at 25 °C, and the light transmission decreases slightly with increasing NaCl concentration. When the temperature dropped to 20 °C, the hydrogel with a NaCl content of 4% began to become opaque, and the transparency dropped to 10%. At 15 °C, the transparency of the hydrogel with a NaCl content of 4% decreased to 0%, and the hydrogel with a NaCl content of 3% decreased from 80% (25 °C) to 10%. At 10 °C, the hydrogel transparency was 0% at a NaCl content of 2%, 3%, and 4%, while the transparency of the hydrogel with a NaCl content of 1% dropped from 90% (25 °C) to 50%. When the temperature dropped to 5 °C, all SN-PAAM hydrogels became opaque (0% transparency) except for the hydrogel without any NaCl. Fig. 4(f) shows the macroscopic optical transparency of SN-PAAM with different NaCl contents from 5 °C to 25 °C. We can observe with naked eyes that the macroscopic optical transparency of the hydrogels at different temperatures follows the same pattern as that exhibited by the light transmission curves of the hydrogels. As can be seen in Fig. 4(f), the hydrogels without SDS and NaCl remain transparent over the entire temperature range of 0–25 °C. It is clear that due to the addition of SDS and NaCl, the SN-PAAM hydrogel possesses the ability to undergo a phase change with temperature (opaqueness–transparency) capability. As the NaCl content increases, it is clear that the low temperature thermal color change point Tk of the hydrogel slowly increases. The effect of NaCl on the thermotropic phase change ability of SN-PAAM hydrogels can be explained by the common ionic effect.30,31 The self-hydrophobic SDS can be ionized and dissolved in water to form free ion pairs composed of Na+ counter ions and amphipathic anions (hydrophilic sulcate SO42− head groups with long hydrophobic tails). The SO42− group is very sensitive to inorganic salts, and upon addition of inorganic salts it can shield the charge of the head group by electrostatic interactions.22,31,36 After the addition of inorganic salts, the addition of Na+ cations weaken the electrostatic repulsion of SDS, making it easier to assemble and form micelles in the hydrogels (Fig. 2(g)–(i)), which ultimately require higher temperatures for solubilization, i.e., elevated Tk temperatures. We analysed the different states (opaque–translucent–transparent) of SN-PAAM (NaCl content of 4%) using optical microscopy, and the results of the analysis are shown in Fig. 2(g)–(i). In Fig. 2(g), an image of the hydrogel at 20 °C is shown, with many large-sized micelles appearing in the image as shown. However, as the temperature increases, the micelles continue to decrease (Fig. 2(h)) until the micelles disappear completely (Fig. 2(i)). Thus, changes in micelles within the hydrogel will affect the refractive index of light and appear as changes in transparency at the macroscopic level. The dynamic hydrophobic association between LMA and SDS, as well as the precipitated crystals produced by the addition of inorganic salts are important factors in the immediate transformation of thermochromic hydrogels.29
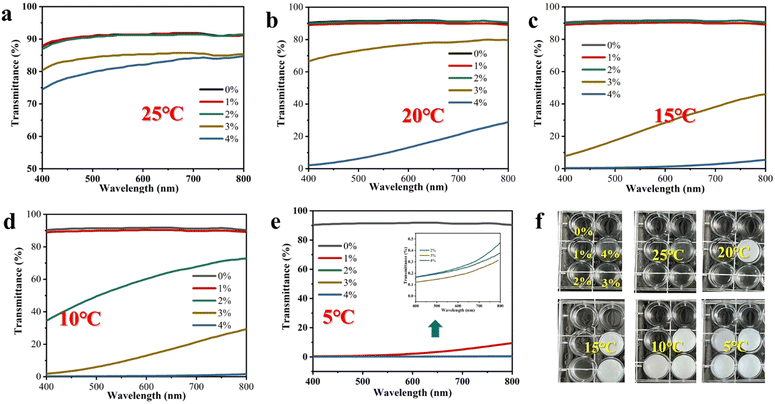 |
| Fig. 4 (a)–(f) Changes in the transparency of SN-PAAM hydrogels with different NaCl contents at 25, 20, 15, 10, and 5 °C. | |
In addition, the stability of temperature regulation is an important feature of stimuli-responsive materials.30 Therefore, the SN-PAAM hydrogel was subjected to 20 cycles of heating–cooling cycles, and the results are given in Fig. 2(e). Remarkably, the resulting hydrogels could withstand 20 repeated cycles, indicating good reliability and durability of the material, which further paves the way for practical applications. By exploiting the excellent thermochromic properties of the SN-PAAM hydrogels, we have applied the hydrogels to camouflage and encryption of a wide range of messages. As shown in Fig. 5(a)–(c), by covering drawings, leaves and text with hydrogel, we can only appreciate the drawings, patterns and text at room temperature (25 °C), and as the temperature decreases, the hydrogel becomes opaque, making the patterns unrecognizable at low temperatures. When the hydrogel sheet is applied to the forehead of a volunteer, as shown in Fig. 5(d), it changes from opaque to transparent, indicating immediate responsiveness to human temperature as well. Thus, the hydrogel anti-counterfeiting label that we designed has obvious dual responsiveness and has promising applications in anti-counterfeiting and information encryption. The SN-PAAM hydrogel has both transparent and opaque states and can be used for information encoding encryption. We define the colorless and transparent PAAM hydrogel block as binary code “0” throughout the process, while the SN-PAAM hydrogel block with thermogenic phase change properties is binary code “1”. The two hydrogel precursor solutions were injected into the designated molds and cured to give two types (2 cm long, 2 cm wide, and 2 cm high) of hydrogels. As shown in Fig. 6(a), the two hydrogels can be programmed and combined to form an ensemble, with each line of the hydrogel representing a letter according to the binary code. Fig. 6(b) and (c) show the thermogenic phase change reaction properties of the combined hydrogels. At 25 °C, the whole hydrogel combination is transparent; while as the temperature decreases, the SN-PAAM hydrogel with thermotropic phase change properties starts to become opaque. We can obtain the “NRI” as well as the “HEL” information. This matrix combination allows for the dynamic storage of cryptographic information in a single storage cell, greatly improving the security of information storage associated with mobile data encryption and validating a programmable encryption and decryption technique through temperature regulation. We believe that our work opens a new path for the development of temperature-stimulated responsive materials that are promising for a wide range of applications in areas such as information encryption and anti-counterfeiting.
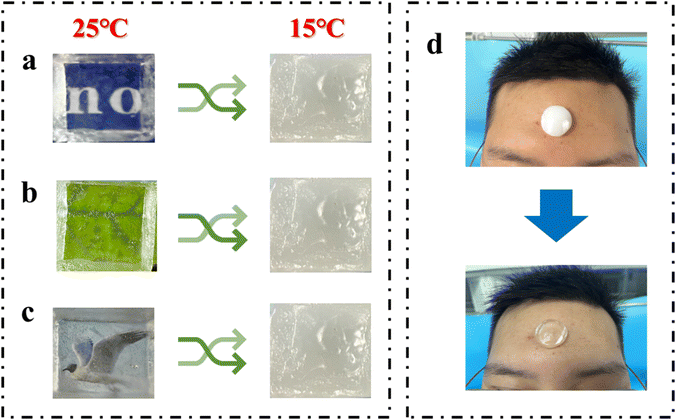 |
| Fig. 5 (a)–(c) SN-PAAM hydrogel for anti-counterfeiting and message encryption. (d) Temperature responsiveness of the SN-PAAM hydrogel. | |
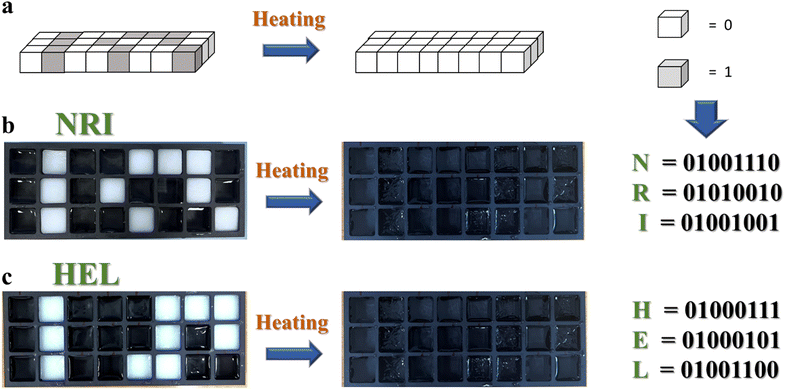 |
| Fig. 6 (a) Schematic diagram of binary encoding of SN-PAAM hydrogels. (b) and (c) Pictures of binary encoding of SN-PAAM hydrogels. | |
Conductivity and temperature/strain sensing properties of SN-PAAM hydrogels
The addition of NaCl not only modulates the mechanical and thermotropic phase change properties of the SN-PAAM hydrogels, but also gives the hydrogels excellent electrical conductivity. The electrical conductivity of the SN-PAAM hydrogels with different NaCl contents and at different temperatures is provided in Fig. 7. Fig. 7(a) shows the conductivity of pure PAAM hydrogels without any NaCl, SN-PAAM hydrogels with 1%, 2%, 3%, and 4% NaCl. It is clear that the PAAM hydrogels exhibit a low electrical conductivity. However, by introducing NaCl into the hydrogel, the SN-PAAM hydrogel showed a significant increase in conductivity, with the NaCl content increasing gradually to a maximum of 5.7 mS cm−1. Since the conductivity of this hydrogel is mainly due to the presence of free Na+ and Cl− in the system, the conductivity gradually increases with the increase in NaCl content. This indicates that the addition of NaCl plays an important role in increasing the conductivity of the hydrogel (Fig. 7(b)). The excellent conductivity of the hydrogel allows the SN-PAAM hydrogels to be used as flexible electronic devices. Since the SN-PAAM hydrogel is a thermotropic phase change material and is sensitive to temperature, we tested the conductivity of the SN-PAAM hydrogel (4% NaCl content) at temperatures of 5, 15, 25, 35, 45, and 55 °C. As shown in Fig. 7(c), the conductivity of the SN-PAAM hydrogel is the lowest at 5 °C (0.18 mS cm−1) and becomes progressively greater as the temperature rises. It can also be noted from Fig. 7(c) that the increase in conductivity of the SN-PAAM hydrogels is the greatest when the temperature increases from 5 °C to 15 °C (Fig. 7(d)). The different conductivity of SN-PAAM at different temperatures allows it to be used in temperature sensors. This greatly broadens the application of the hydrogel as flexible sensors.
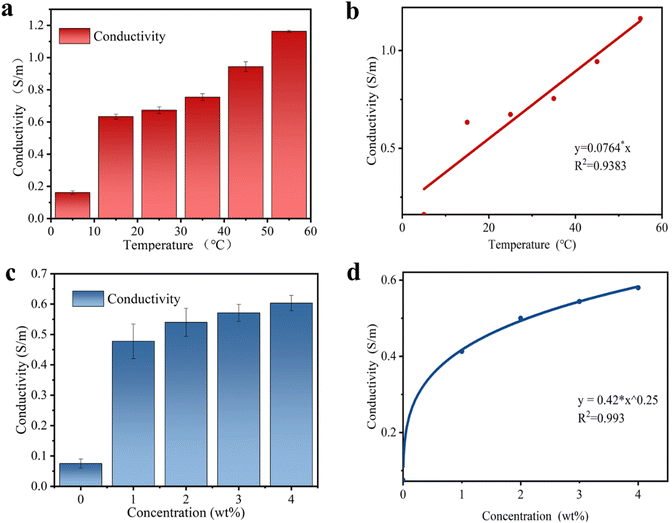 |
| Fig. 7 (a) Conductivity of SN-PAAM hydrogels with 4% NaCl at different temperatures. (b) Fitting curves for the conductivity of hydrogels with 4% NaCl at different temperatures. (c) Conductivity of SN-PAAM hydrogels with different NaCl contents. (d) Fitting curves for conductivity of hydrogels with different NaCl contents. | |
The SN-PAAM hydrogel has very good mechanical stability and electrical conductivity (5.7 mS cm−1), which ensures that the organic hydrogel can be used to assemble flexible strain sensors. The change in resistance of the SN-PAAM hydrogel flexible sensor was recorded by means of a mustimeter. Fig. 8(a)–(c) shows the relative resistance change curves for the SN-PAAM hydrogels with different elongations. During cyclic stretching, the SN-PAAM hydrogel shows excellent sensing repeatability for both small strains (1–5%) or large strains (600–1000%) of relative resistance change. The resistance of the SN-PAAM hydrogel shows a stable smoothing period during strain retention, indicating that the hydrogel has excellent electrical stability. To further investigate stability of the SN-PAAM hydrogels, 200 load–unload tensile cycles were performed at 200% strain, and the change in relative resistance of the SN-PAAM hydrogels was measured (Fig. 8(d)). The resistance signal shows a cyclic variation over time within 200 tensile cycles and does not weaken after multiple repeated cycles, indicating excellent stability of sensing performance of the SN-PAAM hydrogel. The SN-PAAM organic hydrogel strain sensor exhibited excellent sensitivity over a wide operating range. Fig. 8(e) shows that relative resistance change (ΔR/R0) of the SN-PAAM hydrogel increases gradually with the increase of strain in the range of 0–1000%. The measurement factor (GF) is defined as the rate of change in relative resistance of the SN-PAAM organic hydrogel at different tensile strains, indicating the sensitivity of the hydrogel flexible sensor. The GF of the organic hydrogel was stable at 1.62 over the strain range of 0–200%, while it rose to 2.46 at strains greater than 200%. Response time and recovery time are necessary for a flexible hydrogel sensor to respond quickly and effectively to a stimulus in practical applications.6Fig. 8(f) shows response time and recovery time of the hydrogel, and the response/recovery time refers to the time interval between the strain change and the corresponding change in resistance. During loading–unloading, the SN-PAAM organic hydrogels have a fast response time as short as 120 ms and a recovery time of 130 ms, which is similar to the response time of human skins (∼100 ms).6
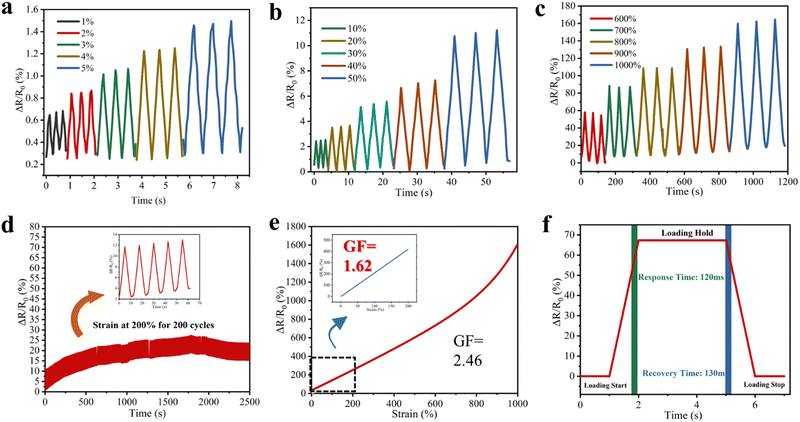 |
| Fig. 8 Electrical sensing performance of SN-PAAM organo-hydrogels. Relative resistance variation of the organo-hydrogel sensor at (a) small strains (1–5%), (b) medium strains (10–50%), and (c) large strains (600–1000%). (d) Stability of the electrical sensing performance of the organo-hydrogel-based sensor over 200 cycles (200%). (e) Dynamic tensile sensitivity of the organo-hydrogel-based sensor. (f) Response time testing of the organic hydrogel-based sensor. | |
The SN-PAAM organic hydrogels have excellent electrical conductivity, excellent mechanical properties, high strain sensitivity, and can be assembled into wearable strain sensors. The SN-PAAM hydrogel samples are applied to various parts of the body (such as fingers, wrists, knees, and neck) to monitor human movement. As shown in Fig. 9(a)–(d), the SN-PAAM hydrogel strain sensor detected and recorded signals from bending of various body parts (such as fingers, wrists, knees, and neck). ΔR/R0 gradually increased as the fingers were bent from 0° to 90° and the resistance signal sensitively increased and periodically recovered, demonstrating the reliable repeatability and stability of the sensor, signifying that the sensor can accurately detect various body parts strain activity for large-amplitude movements. In addition to monitoring large strains in body parts, the hydrogel sensor is also able to accurately sense different angles of finger changes and small vibrations in vocal cords of the throat in real time. The SN-PAAM hydrogel is integrated into the finger for fine angular motion monitoring (Fig. 9(e)). As the finger is bent to different angles (0°, 30°, 60°, and 90°), the resistance of the hydrogel increases differently. Therefore, the SN-PAAM hydrogel is fully capable of accurately reflecting the degree of finger flexion by the degree of change in resistance. As shown in Fig. 9(f), the signal obtained when the volunteer says “Hi” shows consistency and reproducibility. This ability to recognize small strains allows the sensor to be applied to monitoring of smile angles during movement and detection of speech sounds.
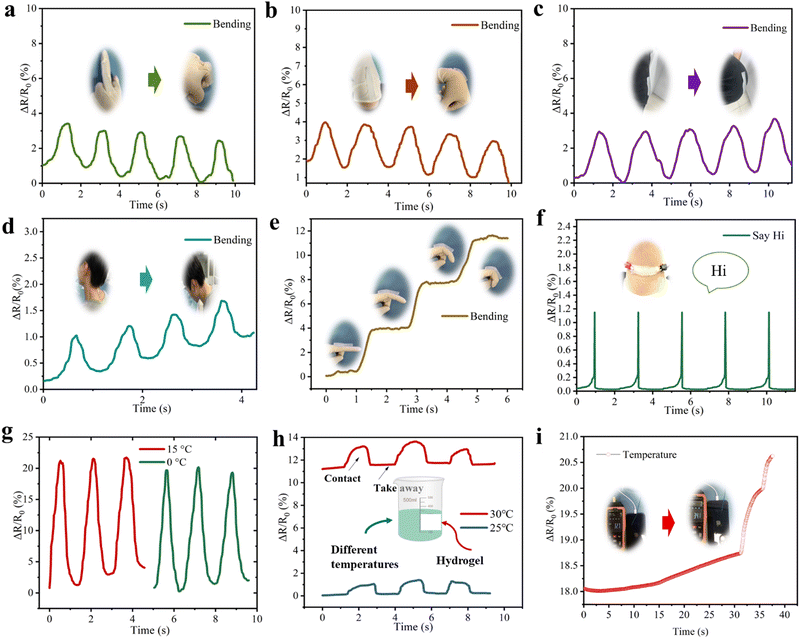 |
| Fig. 9 Real-time relative resistance variations of the SN-PAAM sensors attached on different body joints: (a) finger joint, (b) finesse joint, (c) knee joint, and (d) neck joint. (e) Relative resistance changes of hybrid hydrogels in response to fingers bent at different angles (0°, 30°, 60°, and 90°). (f) Relative resistance changes of hybrid hydrogels in response to tiny human motions: speaking. (g)–(i) Real-time relative resistance changes of SN-PAAM sensors at different temperatures. | |
In addition to responding to external strains, the SN-PAAM hydrogel can also monitor different external temperature changes. As shown in Fig. 9(g), the SN-PAAM hydrogel temperature sensor detects and recognizes the signals of a finger bent at different temperatures (0 °C and 15 °C). It is clear that the values of ΔR/R0 are significantly different when the temperatures are different, and thus can be used to monitor changes in the external environment. As shown in Fig. 9(h), when the cups containing different temperatures were repeatedly replaced on the upper right side of the SN-PAAM sensor, the output resistance signal repeatedly changed accordingly. In addition, the SN-PAAM, as a temperature sensor, can also monitor the charging process of a mobile phone in real time. Fig. 9(i) shows that the ΔR/R0 value of the SN-PAAM sensor adhered to the back of the mobile phone increases with the charging time (32.7–34.1 °C). The SN-PAAM hydrogel can cleverly detect changes in stress and temperature via electrical signals, showing uniquely appealing dual-stimuli responsiveness, which opens up new avenues for the design and fabrication of multisession flexible devices and skin-like sensors.
Sensitivity is an important indicator for temperature sensors, and strain sensitivity of our hydrogel can be affected by temperature since our hydrogel is dual-responsive, which means that monitoring surrounding temperature is required to accurately measure strain. In Fig. S8 (ESI†), to clarify the sensitivity of the hydrogel temperature sensor, we calculated the temperature coefficient of resistance (TCR), i.e., the slope of the fitted curve, for different strains (50–200%) in the temperature range of −5 °C to 45 °C, respectively (ΔR/R0 at 5 °C was set as the initial value). It is clear that ΔR/R0 is positive when the temperature is lower than 5 °C but negative when the temperature is higher than 5 °C, which helps to determine heat/cold sources. As shown in Fig. S8 (ESI†), the TCR values of the hydrogels are between −0.009% °C−1 and −0.11% °C−1 for the strain range of 50–200%, demonstrating good thermal responsiveness of the hydrogels. As shown in Fig. S9 (ESI†), we also illustrated fitting curves of ΔR/R0 at different temperatures for different strains to establish the relationship of temperature sensitivity and strain. The curves show that the TCR of the hydrogel decreases as the strain increases. The reason is that the resistance of the hydrogel continuously becomes larger and the TCR becomes smaller as the strain increases.
Adhesive properties are also important for a device to work as a wearable sensor. In order to quantitatively assess the adhesive strength of our hydrogels on different surfaces, peel force of the hydrogels on different substrates was measured. Four representative substrates including hogskin, plastic, rubber, and glass were selected for 180° peeling test. As shown in Fig. S4 (ESI†), the adhesive strength of the hydrogels on different surfaces was 24.5 N m−1, 30 N m−1, 28.5 N m−1, and 22 N m−1 for hogskin, plastic, rubber, and glass, respectively. In addition, the hydrogels exhibited reproducible adhesion on plastic. As shown in Fig. S5 (ESI†), the peel strength remained consistent throughout five parallel peel tests, indicating excellent and repeatable bonding performance.
Conclusions
In summary, we have successfully synthesized SN-PAAM multifunctional ion-conductive hydrogels by a “one-pot” method with excellent mechanical properties: high tensile strength of 581 kPa, high modulus of elasticity of 29.19 kPa, ultra-long elongation of 1836%, and excellent toughness of 479.67 kJ m−3 and good self-recovery after successive loading–unloading cycles (this is due to the reversible conjoining–reconjoining process which effectively dissipates energy during the stretching process). After introduction of the anionic surfactant SDS and NaCl, the organic hydrogels have the ability to undergo thermotropic phase transitions (the SN-PAAM hydrogels can switch between two states (opaque–transparent) in less than 10 seconds), which is much faster than the thermal colour change techniques used for many other materials. The SN-PAAM ionic hydrogel is also doubly responsive to strain and temperature, allowing it to be attached directly to human skins and used as a strain sensor to detect various body movements such as finger flexion, knee bending, and speech in real time, and as a temperature sensor to monitor ambient temperature in real time. The SN-PAAM hydrogel sensors exhibit excellent sensing capability and reversibility after both 200 load–unload cycles at 200% strain and more than 20 transparent–opaque cycles. In addition, the unique phase change capability of the SN-PAAM hydrogel can be combined with binary coding to form a matrix to dynamically store cryptographic information in a single storage unit, greatly improving the security of information storage associated with mobile data encryption and showing a wide range of applications in areas such as information encryption and anti-counterfeiting. In summary, our SN-PAAM ion-conductive hydrogel design strategy promises to be a new generation of smart and dual stimuli-responsive hydrogels for visualized bionic skins, interactive wearables, anti-counterfeiting, and encryption.
Author contributions
Dongmei Lei: investigation, methodology, formal analysis. Yunchao Xiao: investigation, methodology, formal analysis, funding acquisition. Man Xi: investigation, formal analysis. Yang Jiang: supervision, project administration, writing – review & editing. Yi Li: conceptualization, funding acquisition, writing – original draft.
Conflicts of interest
The authors declare that they have no known competing financial interests or personal relationships that could have appeared to influence the work reported in this paper.
Acknowledgements
This work was supported by Zhejiang Provincial Natural Science Foundation (LY22E030012; LQ22C100002), Public Welfare Project of Jiaxing Municipal Bureau of Science and Technology (2021AY10056), and National Natural Science Foundation of China (NSFC) (no. 81901900).
Notes and references
- J. Chen, G. Zhu, J. Wang, X. Chang and Y. Zhu, Multifunctional Iontronic Sensor Based on Liquid Metal-Filled Hollow Ionogel Fibers in Detecting Pressure, Temperature, and Proximity, ACS Appl. Mater. Interfaces, 2023, 15(5), 7485–7495 CrossRef CAS PubMed.
- M. Peng, X. Li, Y. Liu, J. Chen, X. Chang and Y. Zhu, Flexible multisensory sensor based on hierarchically porous ionic liquids/thermoplastic polyurethane composites, Appl. Surf. Sci., 2023, 610, 155516 CrossRef CAS.
- L. Chen, X. Chang, H. Wang, J. Chen and Y. Zhu, Stretchable and transparent multimodal electronic-skin sensors in detecting strain, temperature, and humidity, Nano Energy, 2022, 96, 107077 CrossRef CAS.
- S. Xia, W. Fu, J. Liu and G. Gao, Recyclable hydrogel for human-machine interface of multi-mode human vital signal acquisition, Sci. China Mater., 2023, 66(7), 2843–2851 CrossRef.
- J. Tie, Z. Mao, L. Zhang, Y. Zhong, X. Sui and H. Xu, Highly sensitive, durable, environmentally tolerant and multimodal composite ionogel-based sensor with an ultrawide response range. Science China, Materials, 2023, 66(5), 1899–1910 CAS.
- L. Zeng and G. Gao, A stretchable organohydrogel with adhesion, self-healing and environment-tolerance for wearable strain sensor, ACS Appl. Mater. Interfaces, 2023, 15(24), 28993–29003 CrossRef CAS PubMed.
- B. Kang, X. Yan, Z. Zhao and S. Song, Dual-Sensing, Stretchable, Fatigue-Resistant, Adhesive, and Conductive Hydrogels Used as Flexible Sensors for Human Motion Monitoring, Langmuir, 2022, 38(22), 7013–7023 CrossRef CAS PubMed.
- R. Zhang, C. Liu, C. Wei, Y. Wang, F. Li, Z. Zhang, J. Qu, N. Qing and L. Tang, Thermoplastic charge-transfer hydrogels for highly sensitive strain and temperature sensors, J. Mater. Chem. A, 2023, 11(15), 8320–8329 RSC.
- X. Chai, J. Tang, Y. Li, Y. Cao, X. Chen, T. Chen and Z. Zhang, Highly Stretchable and Stimulus-Free Self-Healing Hydrogels with Multiple Signal Detection Performance for Self-Powered Wearable Temperature Sensors, ACS Appl. Mater. Interfaces, 2023, 15(14), 18262–18271 CrossRef CAS PubMed.
- A. Abodurexiti and X. Maimaitiyiming, Self-Healing, Anti-Freezing Hydrogels and Its Application in Diversified Skin-Like Electronic Sensors, IEEE Sens. J., 2022, 22(13), 12588–12594 CAS.
- L. Wang, J. Wang, C. Fan, T. Xu and X. Zhang, Skin-like hydrogel-elastomer based electrochemical device for comfortable wearable biofluid monitoring, Chem. Eng. J., 2023, 455, 140609 CrossRef CAS.
- Y. Lu, Y. Yue, Q. Ding, C. Mei, X. Xu, S. Jiang, S. He, Q. Wu, H. Xiao and J. Han, Environment-tolerant ionic hydrogel–elastomer hybrids with robust interfaces, high transparence, and biocompatibility for a mechanical–thermal multimode sensor, InfoMat, 2023, 5(4), e12409 CrossRef CAS.
- Y. Zhao, X. Fu, B. Liu, J. Sun, Z. Zhuang, P. Yang, J. Zhong and K. Liu, Ultra-stretchable hydrogel thermocouples for intelligent wearables. Science China, Materials, 2023, 66(5), 1934–1940 Search PubMed.
- Y. Wu, J. Liu, Z. Chen, Y. Chen, W. Chen, H. Li and H. Liu, High Multi-Environmental Mechanical Stability and Adhesive Transparent Ionic Conductive Hydrogels Used as Smart Wearable Devices, Polymers, 2022, 14(23), 5316 CrossRef CAS PubMed.
- S. Li, H. Pan, Y. Wang and J. Sun, Polyelectrolyte complex-based self-healing, fatigue-resistant and anti-freezing hydrogels as highly sensitive ionic skins, J. Mater. Chem. A, 2020, 8(7), 3667–3675 RSC.
- Y. Xin, J. Liang, L. Ren, W. Gao, W. Qiu, Z. Li, B. Qu, A. Peng, Z. Ye, J. Fu, G. Zeng and X. He, Tough, Healable, and Sensitive Strain Sensor Based on Multiphysically Cross-Linked Hydrogel for Ionic Skin, Biomacromolecules, 2023, 24(3), 1287–1298 CrossRef CAS PubMed.
- W. Zhang, B. Wu, S. Sun and P. Wu, Skin-like mechanoresponsive self-healing ionic elastomer from supramolecular zwitterionic network, Nat. Commun., 2021, 12(1), 4082 CrossRef CAS PubMed.
- Y. Zhou, C. Wan, Y. Yang, H. Yang, S. Wang, Z. Dai, K. Ji, H. Jiang, X. Chen and Y. Long, Highly Stretchable, Elastic, and Ionic Conductive Hydrogel for Artificial Soft Electronics, Adv. Funct. Mater., 2019, 29(1), 1806220 CrossRef.
- X. Zhang, High-sensitivity antidrying hydrogel sensor with interpenetrating network crosslinking structure, J. Mater. Sci.: Mater. Electron., 2023, 34(6), 540 CrossRef CAS.
- D. Wang, J. Zhang, C. Fan, J. Xing, A. Wei, W. Xu, Q. Feng and Q. Wei, A strong, ultrastretchable, antifreezing and high sensitive strain sensor based on ionic conductive fiber reinforced organohydrogel, Composites, Part B, 2022, 243, 110116 CrossRef CAS.
- S. Liu, Y. Chen, J. Feng, J. Peng, Y. Zhou, Y. Zhao, Y. Zhao, Z. Lu, M. Sun, C. Wu, H. Hu, H. Rao, T. Zhou and G. Su, A mechanically soft-tissue-like organohydrogel with multi-functionalities for sensitive soft ionotronics, Chem. Eng. J., 2023, 466, 143087 CrossRef CAS.
- Y. Liu, Y. Han, Z. Huang, P. Qi, A. Song and J. Hao, New focus of the cloud point/Krafft point of nonionic/cationic surfactants as thermochromic materials for smart windows, Chem. Commun., 2022, 58(17), 2814–2817 RSC.
- N. Patra, A. Mal, A. Dey and S. Ghosh, Influence of solvent, electrolytes, β-CD, OTAB on the krafft temperature and aggregation of sodium tetradecyl sulfate, J. Mol. Liq., 2019, 280, 307–313 CrossRef CAS.
- S. Xia, S. Song, F. Jia and G. Gao, A flexible, adhesive and self-healable hydrogel-based wearable strain sensor for human motion and physiological signal monitoring, J. Mater. Chem. B, 2019, 7(30), 4638–4648 RSC.
- J. Yang, Y. Li, X. Yu, X. Sun, L. Zhu, G. Qin, Y. Dai and Q. Chen, Tough and Conductive Dual Physically Cross-Linked Hydrogels for Wearable Sensors, Ind. Eng. Chem. Res., 2019, 58(36), 17001–17009 CrossRef CAS.
- X. Lv, C. Liu, Z. Shao, S. Song and S. Sun, Tailored Ionic Liquids Encapsulation Method Endowing Hydrogels with Excellent Mechanical and Catalytic Activity, ACS Sustainable Chem. Eng., 2020, 8(15), 5975–5984 CrossRef CAS.
- D. C. Tuncaboylu, M. Sari, W. Oppermann and O. Okay, Tough and Self-Healing Hydrogels Formed via Hydrophobic Interactions, Macromolecules, 2011, 44(12), 4997–5005 CrossRef CAS.
- H. Khan, J. M. Seddon, R. V. Law, N. J. Brooks, E. Robles, J. T. Cabral and O. Ces, Effect of glycerol with sodium chloride on the Krafft point of sodium dodecyl sulfate using surface tension, J. Colloid Interface Sci., 2019, 538, 75–82 CrossRef CAS PubMed.
- K. Li, H. Gao, X. Ren and G. Gao, Regulatable Thermochromic Hydrogels via Hydrogen Bonds Driven by Potassium Tartrate Hemihydrate, ACS Sustainable Chem. Eng., 2019, 7(17), 15036–15043 CrossRef CAS.
- L. Bai, Y. Jin, X. Shang, H. Jin, W. Zeng and L. Shi, Dual thermo-responsive multifunctional ionic conductive hydrogel by salt modulation strategy for multilevel encryption and visual monitoring, Chem. Eng. J., 2023, 456, 141082 CrossRef CAS.
- G. Xu, H. Xia, P. Chen, W. She, H. Zhang, J. Ma, Q. Ruan, W. Zhang and Z. Sun, Thermochromic Hydrogels with Dynamic Solar Modulation and Regulatable Critical Response Temperature for Energy-Saving Smart Windows, Adv. Funct. Mater., 2021, 32(5), 2109597 CrossRef.
- K. Li, S. Meng, S. Xia, X. Ren and G. Gao, Durable and Controllable Smart Windows Based on Thermochromic Hydrogels, ACS Appl. Mater. Interfaces, 2020, 12(37), 42193–42201 CrossRef CAS PubMed.
- Y. Ding, Y. Yan, Q. Peng, B. Wang, Y. Xing, Z. Hua and Z. Wang, Multiple Stimuli-Responsive Cellulose Hydrogels with Tunable LCST and UCST as Smart Windows, ACS Appl. Polym. Mater., 2020, 2(8), 3259–3266 CrossRef CAS.
- D. Lei, Y. Xiao, L. Shao, M. Xi, Y. Jiang and Y. Li, Dual-Stimuli-Responsive and Anti-Freezing Conductive Ionic Hydrogels for Smart Wearable Devices and Optical Display Devices, ACS Appl. Mater. Interfaces, 2023, 15(20), 24175–24185 CrossRef CAS PubMed.
- S. Zhang, Y. Zhang, B. Li, P. Zhang, L. Kan, G. Wang, H. Wei, X. Zhang and N. Ma, One-Step Preparation of a Highly Stretchable, Conductive, and Transparent Poly(vinyl alcohol)-Phytic Acid Hydrogel for Casual Writing Circuits, ACS Appl. Mater. Interfaces, 2019, 11(35), 32441–32448 CrossRef CAS PubMed.
- N. Ueno, T. Maruo, N. Nishiyama, Y. Egashira and K. Ueyama, Low-temperature synthesis of ZnO nanorods using a seed layer of zinc acetate/sodium dodecyle sulfate nanocomposite, Mater. Lett., 2010, 64(4), 513–515 CrossRef CAS.
Footnote |
† Electronic supplementary information (ESI) available: These might include comments relevant not central to the matter under discussion, limited experimental and spectral data, and crystallographic data. See DOI: https://doi.org/10.1039/d3tc03359g |
|
This journal is © The Royal Society of Chemistry 2024 |
Click here to see how this site uses Cookies. View our privacy policy here.