DOI:
10.1039/D4VA00121D
(Critical Review)
Environ. Sci.: Adv., 2024,
3, 1679-1697
Di(2-ethylhexyl)phthalate and type 2 diabetes
Received
17th April 2024
, Accepted 27th September 2024
First published on 15th October 2024
Abstract
The prevalence of type 2 diabetes is escalating worldwide and it has been suggested that exposure to endocrine disrupting chemicals, such as phthalates, contributes to the alarming increase. Di(2-ethylhexyl)phthalate (DEHP) is used as a plasticizer in a variety of everyday products; thus humans are constantly exposed to it. Animal studies have associated DEHP with adverse health effects such as reproduction and developmental toxicity, carcinogenicity and metabolic disruption. Concerns over the potential for similar adverse effects in humans are mounting. Recent reviews have reported the link between exposure to a broad set of phthalates and diabetes as well as diabetes-related metabolic conditions. This review evaluates the available information in the literature regarding the association between DEHP exposure and type 2 diabetes and related metabolic conditions, specifically insulin resistance and obesity.
Environmental significance
Phthalates are ubiquitous environmental contaminants and exposure to them has been associated with adverse health effects. This review highlights almost two decades of epidemiological and experimental data associating phthalate exposure, specifically di(2-ethylhexyl)phthalate (DEHP), with diabetes and related adverse metabolic effects. While some associations are clear, some are inconsistent. Nonetheless, as a precaution, DEHP exposure should be minimized. Further studies using models relevant to humans and at environmentally relevant doses could provide a better understanding of the association between DEHP and type 2 diabetes in humans.
|
1 Introduction
Diabetes is a chronic metabolic disease characterized by elevated blood sugar levels.1,2 Type 2 diabetes (T2D) is caused by a combination of genetic and lifestyle factors1 and accounts for about 90% of diabetic cases.2 It is characterized by insulin resistance in target tissues and β cell dysfunction.2 About 537 million adults were living with diabetes in 2021, a number that is estimated to increase to 643 million by 2030.3 The burden due to diabetes on the global health-care system and the wider global economy is unavoidable.1 Some studies have suggested that the increase in environmental exposure to endocrine-disrupting chemicals, such as phthalates, might also contribute to the alarming increase in the prevalence of diabetes.4,5 Di(2-ethylhexyl)phthalate (DEHP) is one of the most widely used high molecular weight phthalates, with a production of several million tons per year worldwide.6 DEHP is mainly used as a plasticizer to make polyvinyl chloride (PVC) more flexible in products such as toys and medical devices. It is also used in food packaging, waterproof clothing, wall coverings and automobile upholstery.7,8 DEHP is non-covalently attached to PVC and can leach from PVC-laden items into the environment.6–8 The widespread use of DEHP gives rise to many possible opportunities for human exposure, which in the general population mainly occurs through ingestion of contaminated food.9,10 A review of seventeen food monitoring surveys conducted in North America, Europe and Asia found that poultry, fats and oils as well as dairy products (cream and cheese) contained high concentrations of DEHP and were likely the main contributors to exposure.11 In another study, DEHP levels ranging from 0.02 to 2685 mg kg−1 were detected in various commercial food samples.12 People may also be exposed to DEHP via ingestion of contaminated indoor dust that has adhered to food or objects9,13 and through medical tubing during medical procedures.8,14–16 DEHP exposure in the general population of the United States is estimated to be 1 to 30 μg per kg of body weight per day.17 However, individuals undergoing chronic treatment such as dialysis and prolonged intensive care treatment16 and neonates in intensive care units14,15 can be exposed to higher levels of DEHP. As a result, some governments have recommended restricting the use of DEHP in medical devices.18 Once it enters the body, DEHP is metabolized by lipases to produce the primary metabolite mono(2-ethylhexyl)phthalate (MEHP) as well as 2-ethylhexanol.19 MEHP is further converted to secondary metabolites which are excreted in urine in their unconjugated form or as glucuronide conjugates, depending on the species.20,21 In humans, 67% of orally administered DEHP is excreted in urine after 24 hours while 75% is eliminated from the body after 2 days.22 Furthermore, MEHP contributes only 5.9% of the excreted urinary DEHP metabolites while the secondary oxidized metabolites mono(2-ethyl-5-hydroxyhexyl)phthalate (5OH-MEHP/MEHHP), mono(2-ethyl-5-oxo-hexyl)phthalate (5oxo-MEHP/MEOHP), mono(2-ethyl-5-carboxypentyl)phthalate (5cx-MEPP/MECPP) and mono(2-carboxymethylhexyl)phthalate (2cx-MMHP/MCMHP) together contribute 61%. Due to their longer elimination half-lives, MECPP and MCMHP are considered suitable indicators of chronic exposure to DEHP while the short elimination half-lives of MEHP, MEHHP and MEOHP are more indicative of short-term exposure.22,23 DEHP metabolites have been detected in more than 90% of urine samples in exposure assessment studies of different populations24–30 and to a lesser extent in breast milk31 and amniotic fluid.32 This level of exposure has raised concerns about its effects on human health, prompting the European Union,33 USA34 and Canada35 to restrict the use of DEHP in certain products. Although the use of DEHP is on the decline in these countries,25,36 DEHP usage is still unregulated in other parts of the world.37 Studies have associated DEHP exposure with adverse health effects such as reproduction and developmental toxicity as well as carcinogenicity.18,38 Moreover, the literature has reviewed the association between exposure to a broad set of phthalates and metabolic conditions such as diabetes,39,40 insulin resistance41,42 and obesity.43,44 This review documents the association between DEHP exposure and T2D and its related metabolic conditions.
2 Epidemiological studies
Most of the epidemiological studies relating DEHP exposure to diabetes and related effects were conducted in countries such as Canada,45 USA46 and Korea47 utilizing biomonitoring data collected from national surveys. Such data, to the best of our knowledge, are lacking in South Africa and Africa as a whole and collecting these data might be beneficial for disease preventative efforts in the African continent, especially because diabetes prevalence has risen faster in middle- and low-income countries than high-income countries.1 Furthermore, the International Diabetes Federation estimates that 3 in 4 adults with diabetes live in low- and middle-income countries.3
2.1 Association between DEHP and diabetes
There is growing evidence that exposure to DEHP may contribute to the development of disorders related to glucose and lipid metabolism. Several epidemiological studies have assessed the relationship between the levels of DEHP metabolite and diabetes. Svensson et al.48 reported a correlation between urinary levels of DEHP metabolites (MEHHP and MEOHP) and the occurrence of T2D in a case–control study involving 221 Mexican women, with 17.4% of the women having self-reported diabetes. A case–control study on USA nurses reported a relationship between MECPP and the sum of DEHP metabolites (ΣDEHPm) and T2D in middle aged women.49 In another study, data from 2350 women participants in the 2001–2008 National Health and Nutrition Examination Survey (NHANES) indicated that ΣDEHPm (MEHP, MEHHP, and MEOHP) was associated with prevalent diabetes.46 Similarly, a positive association was reported between DEHP metabolites (MEHHP, MEOHP and MECPP) and self-reported diabetes in a study conducted in Shanghai involving 2330 adult participants, while MEOHP, MEHHP, MECPP, MCMHP and ΣDEHPox (sum of oxidative metabolites) were associated with self-reported diabetes only in males.29 One study assessed the relationship between serum levels of MEHP and the prevalence of diabetes and found no correlation between the two in an elderly population sample in Sweden,50 which is contrary to the positive relation observed between urinary MEHP concentration and T2D in a Chinese adult population sample.51 In the same case–control study, Duan et al.51 also observed a positive relationship between MEHHP, MEOHP and ΣDEHPm and T2D but an inverse association with MECPP and MCMHP. Studies based on the 2015–2017 Korean National Environmental Health Survey (KoNEHS)52 and 2017–2018 NHANES data53 reported that MEHHP, MEOHP, MECPP and ΣDEHPm levels were related to higher prevalence of diabetes or risk of T2D in adults.
Overall, although some inconsistent findings were reported for associations of MEHP, MECPP and MCMHP with diabetes, most of the studies reported a positive relationship between DEHP exposure and diabetes. Sex-specific associations have been found in some studies29 but not in others52 while age-specific associations were also observed.49,51
2.2 Association between DEHP and insulin resistance
T2D is associated with insulin resistance and hyperglycemia. The data correlating the levels of DEHP metabolites to both these parameters are conflicting. MEHHP and MEOHP were not found to be associated with insulin resistance in the male subgroup of the 1999–2002 NHANES,54 in adolescents and young adults from the young Taiwanese cohort study55 and in a population of Korean women.56 The two oxidative metabolites were also not associated with fasting insulin levels55,56 and glucose levels.55 Dirinck et al.57 observed no correlation between DEHP metabolites (MEHP, MEHHP, MEOHP and MECPP) and glycated hemoglobin (HbA1c), fasting glucose, fasting insulin levels, the homeostasis model assessment of insulin resistance index (HOMA-IR) and the homeostasis model assessment of β-cell function (HOMA-β) in an obese Belgian adult cohort. Kim et al.58 found no association between DEHP metabolites (MEHP, MEHHP, MEOHP and MECPP) and insulin resistance in a population of Korean girls but reported a positive association with MEHHP%, a marker reflecting MEHHP metabolism. A study based on the women subset of the 2001–2008 NHANES also found no association between ΣDEHPm (MEHP, MEHHP and MEOHP) and HbA1c but reported a positive association with HOMA-IR.46 However, another study reported a positive association between MEHP and MEHHP and HbA1c levels in the 2009–2011 Canadian health survey and further observed that ΣDEHPm (MEHP, MEHHP, and MEOHP) was associated with fasting glucose, fasting insulin, HOMA-IR and HOMA-β.45 Duan et al.51 found a positive association between MEHHP and HbA1c levels as well as MEHP and fasting glucose levels in a Chinese adult population. A study based on the 2015–2017 KoNEHS data found positive associations between ΣDEHPm and glycated hemoglobin in males while a negative association was observed with MECPP and ΣDEHPm (MEHHP, MEOHP and MECPP) in females (depending on the urinary adjustment method).47 Positive associations between DEHP metabolites (MEHP, MEHHP, MEOHP and MECPP) and insulin resistance have been recorded in adolescents, based on NHANES data covering the period from 2003 to 2012.59,60 Another study reported a positive relationship between MEHP and increased insulin levels and HOMA-IR in a cohort of young Taiwanese adults but not adolescents.55 However, using the same data, Lin et al.61 found a positive correlation between MEHP levels and higher insulin levels, HOMA-IR and HOMA-β in adolescents and young adults. Huang et al.62 reported a positive association between ΣDEHPm (MEHP, MEHHP and MEOHP) and fasting blood glucose, fasting insulin and insulin resistance in both men and women using NHANES data of 2001–2008. James-Todd et al.63 found associations between ΣDEHPm (MEHP, MEHHP, and MEOHP) and hyperglycemia in a cross-sectional NHANES study (2001–2010) of 2719 adults. A study involving Korean females also reported positive associations between ΣDEHPm (MEHHP, MEOHP, MECPP and MCMHP) and fasting glucose.64 A recent NHANES study (2017–2018) also found that MEHHP, MEOHP, MECPP and their sum are related to fasting insulin levels and HOMA-IR.53
Oxidative stress plays a major role in insulin resistance.65,66 Studies have investigated the link between DEHP exposure and oxidative stress markers, some of which results from the interaction of reactive oxygen species (ROS) with DNA or fatty acids.67 There are reports on the relationship between DEHP metabolites and markers such as serum gamma glutamyltransferase (GGT) and urinary malondialdehyde (MDA), F2-isoprostane, and 8-hydroxydeoxyguanosine (8-OHdG). MEHHP and MEOHP were correlated with MDA and 8-OHdG in a Korean adult population sample; MEHHP also had a positive association with fasting glucose levels.68 Using the 1999–2006 NHANES data, Ferguson et al.69 found a positive association between MEHP and GGT but an inverse association with oxidative DEHP metabolites (MEHHP, MEOHP, and MECPP). DEHP metabolite (MEHP, MEHHP, MEOHP, MECPP and MCMHP) levels were associated with increased levels of F2-isoprostane in a small cohort of adolescents in New York but no associations with insulin resistance were found.70 One study observed an association between DEHP metabolites (MEHP, MEHHP, MEOHP and MECPP) and higher levels of 8-OHdG and 8-isoprostane during pregnancy in a nested case–control study in Boston, USA.67 However, the same DEHP metabolites were not associated with 8-isoprostane during pregnancy in a rural Mexican American population; instead, a marginal association was observed with ΣDEHP.71 DEHP metabolites (MEHHP, MEOHP, MECPP and MCMHP) were correlated with 8-OHdG in a Saudi Arabian population sample72,73 while MEOHP was associated with MDA levels in children.73 Rocha et al.74 observed a positive relationship between MECPP, MCMHP and ΣDEHPox with 8-OHd G levels in a Brazilian children population. A longitudinal study examined the relationship between prenatal DEHP exposure in early and late pregnancy and 8-isoprostane levels in children at 5, 9 and 14 years of age in a population of obese/overweight Mexican American children.75 The study found that maternal MEHP, MECPP and ΣDEHP concentrations measured in early pregnancy were associated with 8-isoprostane in children at 14 years, with borderline associations observed for MEHHP and MEOHP. Kim et al.76 investigated the relationship between DEHP exposure, oxidative stress and insulin resistance in an elderly Korean population. ΣDEHPm (MHEHHP and MEOHP) was associated with fasting glucose, insulin levels, insulin resistance and MDA levels in participants with a previous history of diabetes. The study further established a link between insulin resistance and higher levels of MDA and concluded that DEHP might contribute to insulin resistance by inducing oxidative stress.
Inflammation is another key factor in the development of insulin resistance and it has been suggested that oxidative stress can trigger inflammation response involved in insulin resistance.65,66 One study examined the relationship between DEHP exposure in diabetic patients and oxidative stress, adiponectin, and inflammatory cytokines levels.77 Adiponectin has anti-inflammatory properties and its low levels are associated with insulin resistance and T2D while the expression of the inflammatory cytokine tumour necrosis factor α (TNF-α) is associated with insulin resistance.78 In their analysis, Duan et al.77 reported a positive association between DEHP metabolites (MECPP, MEHHP, MEOHP and MCMHP) and MDA levels in participants of the study. MEHP and ΣDEHPm were also associated with MDA levels in different subgroups of participants, but this association was not observed in the larger BMI subgroup. Oxidative DEHP metabolites were positively associated with serum adiponectin levels while ΣDEHPm (MEHP, MEHHP, MEOHP, MECPP and MCMHP) and MEHP showed inverse associations with adiponectin levels in the larger BMI subgroup. Oxidative DEHP metabolite levels were negatively associated with TNF-α levels while ΣDEHPm and MEHP exhibited a positive relationship with TNF-α in the larger BMI subgroup. The positive association with TNF-α levels was attributed to phthalates contributing to insulin resistance by increasing TNF-α levels while the negative association was attributed to the lower toxicity of oxidative metabolites compared to MEHP. Lee et al.64 also reported a positive association between ΣDEHPm (MEHHP, MEOHP, MECPP and MCMHP) and serum adiponectin levels and surprisingly, they found a similar association with fasting glucose levels in a Korean female study population, leading to the suggestion that DEHP possibly affects fasting blood glucose levels via a mechanism that does not involve adiponectin. A recent study focusing on a different age group to Duan et al.77 reported a negative correlation between urinary levels of MEHP and adiponectin levels along with a positive association between MEHP and insulin resistance in a Taiwanese study involving adolescents and young adults.61 Inconsistencies in epidemiological studies regarding DEHP exposure and adiponectin levels have been observed.61,64
While some studies suggest that DEHP exposure is associated with insulin resistance, there are also data suggesting otherwise. Differences in study populations may account for some of the observed inconsistencies in these associations71 but other sources of variability need to be examined.
2.3 Association between DEHP and obesity
While obesity is a risk factor for diabetes, accounting for about 70% of the risk associated with T2D, the two can exist independently of one another.79 Obesity is associated with the development of insulin resistance which in turn predisposes individuals to T2D.80 Parameters such as body mass index (BMI) and waist circumference are used to predict obesity.81 Several studies have examined the link between DEHP exposure and obesity. A positive correlation was found between MEHHP and MEOHP and waist circumference in men in the 2001–2002 NHANES.54 Similarly, a positive association of MEHHP and MEOHP with BMI and waist circumference was reported in men but an inverse association between MEHP and these parameters in females based on NHANES data from 1999 to 2002.82 Interestingly, using the same NHANES data for the women subset (but up to 2004), another study found that MEHP was positively associated with BMI and additionally reported that the ratio of MEHP
:
MEHHP was positively associated with BMI and waist circumference.83 These contradictory findings regarding MEHP association were attributed to differences in data analysis methods.83 An NHANES study (2001–2010) found associations between higher concentrations of ΣDEHPm and central obesity in male subjects, and further found associations with metabolic syndrome in men as well as in women less than 50 years of age.63 Furthermore, using NHANES data from 2007–2010, positive associations were observed between DEHP metabolites (MEHP MEHHP, MEOHP and MECPP) and an increased risk of obesity in adults.84 Similar associations were observed with BMI in a premenopausal American cohort85 and with central obesity in females less than 45 years of age and adults in a Chinese population (MEHHP and MECPP).86 However, DEHP metabolites (MEHHP, MEOHP, and MECPP) were not correlated with general obesity/BMI and/or abdominal obesity/waist circumference in American women in a nurse's health study87 and in Korean adult cohorts.56,88 A study on obese participants in Belgium reported an inverse association between MEOHP and BMI57 while another study found a positive correlation in an elderly population of Anhui province of China, with a stronger association observed in males.89 Van der Meer et al.90 observed a correlation between MECPP and adiposity-related traits (BMI and waist circumference) in a study population of adults in North Netherlands. A positive relation was observed between MEHHP and ΣDEHPm (MEHHP, MEOHP and MECPP) and obesity in adult participants in the 2015–2017 KoNEHS; however, the association was dependent on the urinary dilution adjustment method.47
Such disparities in the association between DEHP metabolites and obesity have also been documented in children. An NHANES study (1999–2002) found an inverse association between MEHP and BMI and waist circumference in adolescent girls.82 Other studies found no associations between ΣDEHPm/DEHP metabolites (MEHP, MEHHP, MEOHP and MECPP) and body mass outcomes (BMI z-score, overweight and obesity) in children and adolescents based on the NHANES data,84,91 in China92,93 and in Korea58 but reported that MEHHP% was positively associated with obesity.58 On the other hand, Wang et al.94 documented a positive association between MEHP and BMI and waist circumference in a Shanghai children cohort. MEHP, MEHHP and ΣDEHPm were negatively associated with obesity in girls in a Shanghai study95 while Amin et al.96 observed a positive association of MEHP and MEHHP with obesity as well as a low to moderate positive association between DEHP metabolites (MEHP, MEHHP and MEOHP) and BMI in an Iranian children and adolescent population. Recently, Seo et al.97 found a positive association between MECPP and obesity in children using KoNEHS data (2015–2017). Similarly, a prospective case–control study reported a correlation between higher levels of DEHP metabolites (MEHP, MEHHP and MEOHP) and BMI z-scores and higher risk of obesity in a Chinese children population.98
Longitudinal studies have mostly found no associations between ΣDEHPm/DEHP metabolite (MEHP, MEHHP, MEOHP and MECPP) levels and obesity parameters determined in a follow-up period of a year in New York children,99 up to six years in girl participants from three centers in USA100 and up to 24 years in preschool children from a Swedish birth cohort.101 One study assessed the relationship between serum MEHP levels and different obesity indices as determined by dual-energy X-ray absorptiometry and abdominal magnetic resonance imaging in a Swedish elderly population and found no correlation in a two year follow-up period.50
Different DEHP exposure patterns in different study populations may account for some of the disparities observed in the studies above.90 Additionally, studies found that associations between concentrations of urinary DEHP metabolites and obesity vary based on sex,63,82,86,89,94 age,63,82,84,86 race/ethnicity91 and differences in the rate of DEHP metabolism due to genetic variations.61 However, other studies did not find sex88 or age-related differences83 in the associations. Other sources of inconsistencies in the studies are the use of different exposure metrics/exposure assessments; these include urinary dilution adjustment/corrections for dilution and whether individual or summed metabolites are presented.43,102 Recently Lee et al.47 demonstrated that the method used to correct urinary phthalate concentrations for urinary dilution could influence the associations between DEHP metabolites and obesity. Differences in adjustment for confounding factors,83 varying statistical analysis86 and different standards/parameters being used to determine obesity (such as BMI and percentage body fat)95 may also contribute to the inconsistencies.
2.4 Variability of DEHP metabolite levels over time
The use of cross-sectional data to conclude on associations between DEHP metabolites with short half-lives and chronic diseases has been highlighted as a shortfall of epidemiological observations.103 Furthermore, most of the cross-sectional studies involve single urine measurements of phthalates which are thought to reflect recent/acute exposure levels.104,105 Thus, it is suggested that these levels do not reflect long-term exposure or exposure throughout disease development.63,64,80 As such, several studies have assessed whether the DEHP metabolite levels recorded in single urine samples represent an individual's DEHP exposure over time, primarily based on assessment of the intraclass correlation coefficient of single metabolites or ΣDEHPm. Assessment of DEHP metabolite levels (MEHP, MEHHP, MEOHP and MECPP) in spot urine samples obtained during varying periods of pregnancy in various pregnant women populations showed low within-person reproducibility105–114 and fair reproducibility of MEHP and MECPP levels.115 Analysis of DEHP metabolites levels (MEHHP, MEOHP, MECPP and MCMHP) in spot, first morning and 24 hour urine samples collected from men and women for a period of days up to several months showed high intraday and interday within-person variability.104,116–121 A similar trend was observed in children for urine samples collected over periods ranging from six months to years.122,123 Other studies have however suggested that single metabolite measurements are representative/predictive of DEHP exposure over time and have reported moderate reproducibility124 and good correlation76 of DEHP secondary metabolite (MEOHP, MEHHP and MECPP) levels from multiple urine samples obtained over 3 years. Another study reported fair reproducibility in samples collected from children over periods ranging from days to a year, based on pooled samples.115
Reports regarding the consistency of MEHP levels in first morning/spot urine samples collected over time are inconsistent. One study found consistencies in MEHP levels from urine samples provided over 2 days.125 Another study found that the participant's MEHP levels were more consistent in morning spot urine samples collected 2 to 8 days apart compared to first morning and 24-hour samples.117 Casas et al.115 found good reliability of MEHP levels in pooled urine samples collected over a period ranging from days to a year. Hauser et al.126 reported within-subject variability in MEHP levels but moderate sensitivity of a single sample to predict a 3-month average exposure. Another study found that MEHP levels measured in a single sample were predictive of an individual's 6-month average concentration.122 Other studies have however recorded within-person variability in samples collected over several days,118,123 1 to 4 months104,120 and even years.123,124
The discrepancies in the findings above may be due to differences in study design, phthalate exposure patterns and study populations,104,106,118 variations in an individual's food consumption patterns116,126 and changes in phthalate metabolism due to physiological changes during/after pregnancy.106,107 On the other hand, it is suggested that the small variability in phthalate levels over time may be a result of an individual's consistent daily time–activity patterns and stable microenvironmental phthalate concentrations106 or consistent long-term diet patterns.124
The above studies were conducted on specific populations, with only a few studies reporting large and diverse cohorts; therefore studies that are representative of the general population are necessary. The intraday variability of metabolite levels has also highlighted the need to factor in the time of sample collection during the day when carrying out such studies.116,119 Moreover, according to Preau et al.116 the short elimination time associated with phthalates implies that determining exposure to phthalates over weeks or months may require multiple urine measurements. Therefore, longitudinal studies, with multiple urine measurements of DEHP metabolites at different time points, are warranted to confirm the exposure–health outcome associations from cross-sectional studies. This would be especially relevant for diseases that take time to manifest, such as T2D. Although multiple sample measurements are associated with logistical and analysis cost implications,116,127 it appears that they are critical for reliable results in epidemiological studies involving nonpersistent chemicals.128
3 Experimental studies
Epidemiological studies have a limitation of failing to establish a cause-and-effect relationship.61,69 To address this shortcoming, experimental studies have been conducted and a growing body of experimental data suggests a link between DEHP exposure and diabetes and its risk factors.129–135 Several modes of action have been proposed for DEHP and these can assist in explaining the association between DEHP/DEHP metabolites and diabetes. These include activation of peroxisome proliferator-activated receptors (PPARs), induction of oxidative stress, impairment of β-cell function and impairment of adiponectin function.40
3.1 Peroxisome proliferator-activated receptor activation
PPARs are nuclear receptors which form heterodimers with the retinoid X receptor (RXR) and bind to peroxisomal proliferator response element in the promoter regions of their target genes.136 The PPAR–RXR heterodimer can function as a transcriptional factor, activating or repressing gene expression, depending on the presence of co-activators or co-repressors.137 The PPAR family of transcription factors comprises isoforms PPARα, PPARγ and PPARβ/δ. PPARα is predominantly found in the liver and plays a crucial role in fatty acid catabolism.138 PPARγ is predominantly found in adipose tissue; it regulates adipogenesis and plays a role in insulin sensitivity.137 Studies have alluded that DEHP/MEHP exerts its effects through a mechanism that is mediated by PPARγ134,136 and that MEHP binds to the ligand binding domain of PPARγ.139,140 While there are inconsistent findings on the ability of the parent compound DEHP to activate PPARs in vitro,136,141–145 trans-activation assays have shown that MEHP is able to activate the three PPAR isoforms and further studies in intact cells show that MEHP activates biological processes that are mediated by PPARs. MEHP activated both mouse136,141,142,146 and human PPARα,141,142 with mouse PPARα being activated at lower MEHP concentrations than human PPARα.142,146 Interestingly, MEHP was found to be a more potent activator of PPARα than more prevalent monoesters such as monobenzyl phthalate and monobutyl phthalate whose urinary levels were reported to be higher than that of MEHP.146–150 With regard to the stimulation of PPARα target genes, promoter–reporter gene assays showed that MEHP (250 μM) activated PPARα-dependent transcription of the rat acyl-CoA oxidase (ACOX) gene with both mouse and human PPARα. Human ACOX was not responsive with either PPARα, prompting the authors to conclude that DEHP does not pose a health risk to humans.147 Similarly, MEHP concentrations of 10–100 μM stimulated the expression of PPARα target genes ACOX and cytochrome P450 4A in rat liver FAO cells but not in the human HepG2 cells.146 This is contrary to another study where the FAO cell line was not responsive to similar concentrations of MEHP in inducing ACOX gene expression.142 It was also highlighted that MEHP activation of human PPARα would possibly be relevant in exceptional cases of high DEHP exposure since the concentration of MEHP required for PPARα activation in in vitro studies is higher than the maximum serum levels of MEHP detected in the general population.146
In vitro studies have reported that MEHP can also activate mouse and human PPARγ with no species differences in PPAR responsiveness to MEHP.136,141,142,146 MEHP (1 to 50 μM) further induced PPARγ dependent adipogenesis in 3T3-L1 cells,136,142,146,148 with significant upregulation of PPARγ adipogenic target genes at concentrations ranging from 10 to 100 μM.148
Another study found that during differentiation, 3T3-L1 cells treated with MEHP at concentrations of 10–300 μM had reduced gene expression of PPARγ, enhanced expression of some adipogenic markers while the expression of other markers was not affected.145 In addition, the cells accumulated MEHP and had enhanced lipolysis and glucose uptake. Treatment of murine mesenchymal stem cells with 100 μM DEHP induced adipogenesis and upregulated gene transcription of adipogenic markers (PPARγ2, adiponectin, fatty acid binding protein fatty 4 (FABP4) and lipoprotein lipase).143 While a human adipocyte cell line was not included in these studies for comparison, few studies assessed the ability of DEHP/MEHP to activate PPAR and further induce target genes in human cell models. Human subcutaneous pre-adipocytes were differentiated in vitro, and on day 11, the cells (30% undifferentiated and 70% differentiated) were treated with 100 μM MEHP for 4 to 48 h149 MEHP induced differentiation of the remaining preadipocytes and upregulated transcription of genes involved in the PPARγ signaling pathway, triglyceride synthesis and storage as well as glyceroneogenesis in mature adipocytes. It is thus possible that by inducing adipogenesis and lipid accumulation, MEHP could promote obesity150 which could possibly lead to insulin resistance. Schaedlich et al.151 assessed the effect of DEHP, at a concentration similar to that detected in blood bags (50 μg ml−1 or 128 μM), on the differentiation of Simpson–Golabi–Behmel syndrome human preadipocytes. They however reported downregulation of certain adipogenic marker expressions, reduced triacylglyceride content and decreased accumulation of lipid droplets. On the other hand, a study on human liposarcoma SW 872 preadipocytes found that treatment with 10 μM MEHP from day 5 to 11 of adipogenesis increased mRNA levels of PPAR-α, decreased PPAR-γ mRNA levels, but had no effect on triglyceride content. The lack of MEHP effect on triglyceride content was however attributed to the low lipid content of the cell line used.152 Schaedlich et al.151 highlighted how differences in cell cultures and study designs in in vitro studies on human cell models can complicate comparisons.
In vivo, DEHP is converted to MEHP and animal studies investigating exposure to DEHP have reported contradictory data pertaining to the induction of adipogenic PPARγ target genes. Feige et al.150 reported that DEHP (500 mg per kg per day) does not activate PPARγ and adipogenesis, based on the inability of DEHP to induce the expression of adipogenic PPARγ target genes in adipose tissue in mice. Instead, DEHP exposure induced PPARα mediated fatty acid catabolism in the liver which protected the mice against diet-induced obesity. Interestingly, DEHP did not protect engineered mouse models expressing the human PPARα from diet-induced obesity, highlighting species differences in DEHP activated biological processes. However it has been suggested that the differences in PPARα between mice and humans are responsible for the differences in response to DEHP in the two species,150 others have alluded to differences in the PPARα target genes promoters and co-activators as the source of these differences since some PPARα target genes are not activated by either mouse or human PPARα in mouse models.147 From their findings, Feige et al.150 suggested that exposure to DEHP in humans may result in weight gain/obesity possibly via decreased PPARα mediated fatty acid oxidation in the liver. This effect could in turn possibly lead to insulin resistance. However, the authors cautioned that this scenario may apply to individuals exposed to high concentrations of DEHP such as those undergoing dialysis or frequent blood transfusions. The lack of adipogenesis activation by DEHP/MEHP was attributed to insufficient MEHP uptake by the adipocytes and MEHP being a selective activator of PPARγ; they observed that agonists of PPARγ promote adipogenesis and weight gain136,150 and display different coregulator recruitment patterns to target gene promoters.136 Lv et al.153 also observed upregulated mRNA levels of β-oxidation, lipid uptake and lipolysis proteins in the white adipose tissue of male C3H/He mice exposed to 50 and 200 mg per kg body weight per day DEHP for 5 weeks while lower DEHP levels (0.5 mg per kg body weight per day) downregulated the expression of these genes. However, the authors not only reported increased adipogenesis but also found a decline in mRNA levels of key proteins involved in lipogenesis.153
In contrast, adult mice administered 0.5 mg kg−1 of body weight of DEHP or MEHP for 24 hours had increased levels of epididymal adipose and increased expression of PPARγ target genes in epididymal adipose and liver tissues.144,148 DEHP also upregulated the expression of adipogenic transcriptional factors PPARγ, CCAAT/enhancer-binding protein (C/EBP) alpha and sterol regulatory element binding factor 1 (Srebf1) in the liver.144 Schmidt et al.154 observed that mice exposed to 0.05 mg per kg body weight per day DEHP had increased levels of FABP4 gene expression; FABP4 is involved in fatty acid uptake, transport, and metabolism.151 In another study, DEHP exposure (50 mg per kg per day DEHP) in rats upregulated and activated the Janus-activated kinase (JAK)/signal transducer and activator of transcription (STAT) pathway and upregulated fatty acid metabolism-related genes (FABP4, Acox, and fatty acid synthetase) and leptin expression in adipose tissue.155 Interestingly, the expression of JAK and STAT in the liver was upregulated but the pathway was inhibited. Furthermore, expression of the same fatty acid metabolism-related genes was downregulated in the liver, prompting the authors to conclude that lipid synthesis and lipolysis in the liver were reduced by DEHP. The authors suggested that the increased fatty acid synthesis and leptin levels are associated with DEHP exposure promoting lipid accumulation; however they also pointed out that this association was based on very high DEHP levels to which humans may not be exposed.155
Consequently, due to the discrepancies in PPARγ activation in vivo, there are inconsistent reports regarding the effects of DEHP exposure on fat and body mass and whether there are sex differences in these effects. In one study, DEHP exposure (1000 mg per kg body mass per day) in mice resulted in a decrease in body mass and fat mass.150 Hayashi et al.156 found that exposure of adult mice to DEHP at 10 to 140 mg per kg body weight per day for four weeks did not affect body weight. Other studies have however found an increase in body weight and fat mass in female mice while male mice were unaffected following feeding supplemented with 0.05 mg per kg body weight per day.157 Schmidt et al.154 also reported an increase in body weight and visceral fat tissue in mice exposed to 0.05 to 500 mg per kg per day DEHP, although the weight gain was associated with increased food intake. Male mice exposed to DEHP at 0.5, 5, 50 and 200 mg per kg body weight per day for 5 weeks experienced increased body weight and fat mass, as well as increased food intake.154 Rats administered DEHP (5, 50 or 500 mg per kg per day) for four weeks displayed increased body weight155 but in another study, the same DEHP levels had no effect on body weight in adolescent rats following a 28 day exposure period despite increased food intake.158 Long-term exposure (29 weeks) of adult female mice on a high fat diet to 0.05 mg per kg body weight per day DEHP increased body weight and visceral white adipose tissue159 whereas exposure of male rats to 600 mg per kg per day for 12 weeks had no effect on body weight.160 Investigations into perinatal DEHP/MEHP exposure have observed an increase in body mass and fat mass in male mouse offsprings but not in females perinatally exposed only to low levels of MEHP (0.05 mg per kg per day) until postnatal day 7 while these parameters were unaffected by exposure to 0.25 and 0.5 mg per kg body weight per day MEHP.148 The same authors reported an increase in body weight in both male and female mice perinatally exposed to DEHP at 0.25 mg per kg body weight, but not at 0.05 and 0.5 mg per kg body weight until postnatal day 7.144 Perinatal exposure of mice to DEHP at 0.05 and 5 mg per kg body weight per day until weaning (postnatal day 21) resulted in increased body weight in both male and female offsprings. The offsprings' body weight increased 9 weeks after weaning, with female mice having more fat storage.154 Rajesh and Balasubramanian161 found that offsprings of mice treated with DEHP (10 and 100 mg per kg per day) from gestation day 9 to 21 had increased fat mass at postnatal day 60 while the authors reported reduced body weight in a separate study.132 DEHP exposure during gestation and lactation at 1.25 and 6.25 mg per kg per day129 and 1, 10 and 100 mg per kg per day162 also reduced body weight in offsprings but increased body weight at puberty at a dosage of 700 mg per kg per day.163 Adult male rats exposed to 7 and 75 mg per kg per day DEHP during lactation or puberty did not display changes in body weight.164 Others have attributed the differences in DEHP/MEHP effects at low and high concentrations on bodyweight/fat mass to the inverted U-shaped dose–response curve for DEHP,148 however, some studies involving similar concentrations of DEHP/MEHP have yielded contradictory findings.153–156,158 Some inconsistencies reported here may be due to the differences in the study design,157,159 DEHP/MEHP dosage and timing of exposure as well as the mode of DEHP/MEHP administration and exposure duration.148 Nonetheless, there seem to be discrepancies regarding whether DEHP exposure promotes body weight gain in vivo.
Inconsistencies between in vitro and in vivo data reported in the abovementioned studies regarding DEHP exposure and adipogenesis require further investigations. These studies also highlight the importance of in vivo studies, where all tissues expressing the different PPAR isoforms are present, especially for metabolites such as MEHP that can activate all PPAR isoforms. In addition, the ability of compounds to elicit species specific biological effects necessitates the need for additional experiments in suitable human cell models.
3.2 DEHP and β-cell dysfunction
In T2D, pancreatic β-cells accelerate the production and secretion of insulin in an attempt to compensate for insulin resistance in target cells.54 While this effect initially results in an increase in insulin levels, it eventually places a burden on the β-cells, ultimately leading to reduced insulin secretion.165 Oxidative stress and endoplasmic reticulum (ER) stress have been associated with β-cell dysfunction that eventually leads to diabetes.166 Oxidative damage in β-cells can impair insulin production/secretion or induce apoptosis.66
DEHP exposure during pregnancy can be a risk factor for the development of diabetes in offsprings. For instance, administration of DEHP at 1.25 and 6.25 mg per kg per day129 or 1, 10 and 100 mg per kg per day132 during pregnancy and lactation impaired the expression of genes involved in β cell development and function in the offsprings. DEHP downregulated the expression of transcription factors critical for insulin synthesis, reduced insulin content and upregulated genes involved in ER stress in pancreatic cells in the offsprings at weaning129 and adulthood.132 Furthermore, adult offsprings displayed elevated fasting blood glucose and reduced serum insulin levels, reduced expression of glucose sensors, impaired insulin signaling, and glucose and insulin intolerance along with reduced ex vivo insulin secretion upon glucose stimulation.132 Additionally, DEHP exposure increased the level of global hypermethylation, prompting the authors to suggest that DEHP-induced hypermethylation is a possible mechanism for β-cell dysfunction. Global DNA methylation has been associated with increased risk of insulin resistance, although this observation was made in peripheral blood leukocytes and not diabetes-affected organs.167 Lin et al.129 made similar observations of fasting hyperglycemia, hypoinsulinemia and glucose intolerance but only in adult female offsprings and further noted reduced β-cell mass and insulin content as well as reduced in vivo and ex vivo insulin secretion upon glucose stimulation. The male adult offsprings displayed fasting glucose levels comparable to the control group and increased fasting serum insulin levels along with increased in vivo and ex vivo insulin secretion. However, another study found lower glucose stimulated insulin secretion ex vivo in isolated islets of adult male offsprings exposed to DEHP during lactation, with discrepancies in findings attributed to the dose and timing of DEHP exposure.164 When comparing the effects of DEHP on glucose homeostasis at different stages of development, adult offsprings exposed to DEHP during lactation had reduced insulin sensitivity and were more sensitive to DEHP than those exposed at puberty.168 DEHP exposure during lactation and puberty resulted in elevated fasting blood glucose; however no effect on serum insulin levels was observed. Unlike Lin et al.129 and Rajesh and Balasubramanian,132 another study found a non-significant reduction in pancreatic and duodenal homeobox 1 (PDX-1) protein levels in male and female offsprings upon exposure to 7, 70 and 700 mg per kg DEHP during utero and lactation periods.163 The offsprings in the high DEHP group displayed higher fasting blood glucose levels and reduced insulin sensitivity, with decreased ex vivo glucose stimulated insulin secretion in male offsprings. In another study, 3-week-old male rats administered DEHP (750 mg kg−1) for 8 weeks had elevated fasting glucose levels, reduced HOMA-β, damaged pancreatic tissue and increased MDA levels while superoxide dismutase (SOD) activity remained unaffected.168 Inhibition of the phosphatidylinositol 3-kinase/protein kinase B (PI3K/Akt) signaling pathway was also observed, along with pancreatic cell apoptosis.
In vitro studies assessing the direct effects of DEHP and its metabolites on pancreatic β-cells have shown that DEHP exposure affects the ability of these cells to produce and secrete insulin. In evaluating the suitability of the rat pancreatic β-cell line INS-1 (subclone 832/13) as a screening system for the assessment of DEHP effects on β cell function, one study found that exposure of cells to 100 μM DEHP for 2 hours increased basal insulin secretion but did not affect glucose stimulated insulin secretion.169 Sun et al.133 reported that INS-1 cells exposed to DEHP (5–625 μM) displayed an increase in ROS and a decrease in the cells' nuclear factor erythroid 2-related factor 2 (Nrf2)-dependent antioxidant response at higher DEHP doses; however lower doses of DEHP (5 μM) activated the antioxidant response. This effect was coupled to decreased insulin mRNA and protein levels at higher DEHP doses, thus affecting the amount of insulin released. In addition, DEHP exposure induced ER stress-mediated apoptosis by activating the protein kinase RNA-like endoplasmic reticulum kinase/activating transcription factor 4/C/EBP homologous protein (PERK-ATF4-CHOP) ER signaling pathway.133 A more recent study found that DEHP (30 μM) induced ROS and caused apoptosis in INS-1 cells via inhibition of the PI3K/Akt/Bcl-2 signaling pathway, which resulted in reduced insulin secretion due to a reduction in the number of cells.170 RIN-5F cells treated with 625 μM DEHP displayed elevated ROS production and lipid peroxidation and reduced enzymatic antioxidant levels.171 DEHP also reduced insulin secretion and the protein levels of insulin receptor (IR), insulin receptor substrate-1 (IRS-1) and glucose transporter 2 (GLUT2) and induced apoptotic changes in the cells. Although She et al.172 did not assess the effects of DEHP exposure on insulin-related parameters, they observed that DEHP (200 and 400 μM) caused INS-1 cell dysfunction via oxidative stress marked by elevated ROS generation, reduced glutathione levels and SOD activity and increased MDA levels; these effects were reduced in the presence of the antioxidant compound pyrroloquinoline quinone. DEHP also increased lysosomal membrane permeability, decreased mitochondrial membrane potential and induced DNA damage. Contrary to She et al.,172 a recent study reported that MEHP (0.001–1000 μM) did not affect ROS and MDA levels or mitochondrial membrane potential and lysosomal membrane permeability in 1.1B4 pancreatic cells.173 Instead, MEHP induced ER stress and inflammation (at low MEHP levels), downregulating PPARα and PPARγ expression. Low levels of MEHP decreased insulin secretion at low glucose concentrations but increased the levels of proteins involved in β cell function (GLUT1, musculoaponeurotic fibrosarcoma homolog A (MafA), PDX-1 and glucokinase), while lower glucokinase and GLUT1 protein levels were observed at high DEHP levels (1000 μM).173 While the authors attributed inconsistent findings to the use of DEHP instead of MEHP, a different study observed increased ROS generation and decreased antioxidants in INS-1 cells treated with MEHP (0.1 to 10 μM) for 24 to 72 hours.135 Additionally, there was downregulation of the expression of insulin genes 1 and 2 and PDX-1 genes, along with decreased insulin secretion at normal glucose stimulation (5.5 mM). Another study found that a 72 hour treatment of INS-1E cells with MEHP (500 μM) resulted in reduced insulin secretion following glucose stimulation, an effect that was attributed to cell death due to MEHP-induced toxicity.174 Interestingly, an increase in insulin secretion, was observed following simultaneous exposure of the cells to 100 μM MEHP and normal levels of glucose (6.7 mM) for 2 hours.
A recent study found that MEHP (400 μM) caused nucleotide-binding oligomerization domain-like receptor protein 3 (NLRP3)-dependent pyroptosis, an inflammatory programmed cell death, and affected autophagy in INS-1 cells.175 In other cell lines, treatment of mouse MIN6 cells with DEHP (100 pM to 10 μM) for 24 hours impaired glucose stimulated insulin release, with decreased insulin content only observed at 1 μM DEHP. The expression of the GLUT2 gene was upregulated at 10 μM DEHP while that of PDX-1 and MafA was unaffected by all DEHP concentrations.176 Given the limited studies on human β cell models, one study evaluated the use of the human pancreatic β-cell line Endo C-βH1 as a model for the assessment of DEHP exposure.176 Decreased insulin secretion by the cells was only observed after 7 days of DEHP treatment (1–100 nM) and was associated with a decrease in insulin content at 10 and 100 nM DEHP. Interestingly, a concentration of 1 μM DEHP increased insulin secretion by the cells, prompting the authors to conclude that the cells exhibit a non-monotonic dose response to DEHP exposure.
The studies above show that DEHP/MEHP increases generation of ROS in β cells in vitro, weakens the β-cell antioxidant system and impairs insulin production/secretion. In some cases, the oxidative stress was associated with apoptosis (Fig. 1 top panel). Furthermore, these studies suggest that in utero DEHP exposure causes disturbances in β-cell development and function as well as glucose homeostasis in the offsprings in early life or in the long term. As evidenced by the reviewed studies, most of the data on the effect of DEHP on β-cell function were gathered using mouse cell lines while in vivo studies were conducted in animals such as rats. Since it has been argued that findings from animal studies are not easily extrapolated to humans due to species differences,38 the recent findings by Al-Abdulla et al.176 which demonstrate that DEHP concentrations relevant to human exposure impair insulin secretion in the human pancreatic β-cell line is a step in the right direction in advancing our understanding of the association between DEHP exposure and diabetes.
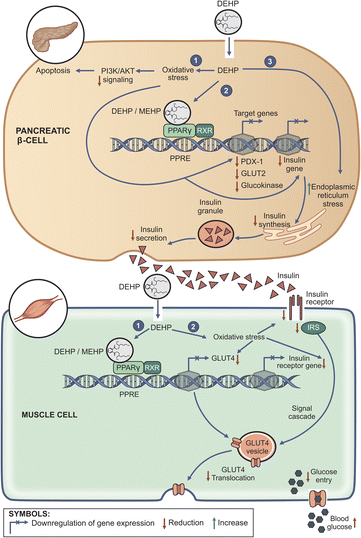 |
| Fig. 1 Overview of some of the proposed mechanisms for the DEHP-induced metabolic disruption. In the pancreatic β cells (top panel), DEHP induces oxidative stress-mediated apoptosis (1) and endoplasmic reticulum stress (3). Oxidative stress also downregulates PDX-1 (a transcription factor involved in insulin synthesis) and insulin gene expression. DEHP/MEHP also binds to PPARγ which together with RXR binds to PPAR response elements (PPRE) in the promoter regions of genes involved in insulin synthesis (PDX-1) and glucose sensing (GLUT2 and glucokinase genes) and downregulates their expression (2). A combination of these factors ultimately impairs insulin synthesis and secretion. In the muscle cells (bottom panel) DEHP binds to PPARγ and the PPARγ–RXR heterodimer binds to PPRE in the promoter region of the GLUT4 gene and downregulates its expression (1). DEHP induces oxidative stress, downregulating the expression of GLUT4 and insulin receptor genes (2). Oxidative stress also reduces the levels of key molecules in the insulin signaling process (2), ultimately reducing translocation of GLUT4 to the cell membrane. A combination of these factors results in a decrease in glucose uptake, hence hyperglycemia. | |
3.3 DEHP and insulin resistance
Some studies investigating the effects of developmental DEHP exposure on rat offsprings found that DEHP impairs insulin signaling in the muscles and liver.161,162 Pregnant rats were administered DEHP (1, 10 and 100 mg per kg body weight per day) and analysis of adult offsprings showed high fasting blood glucose levels, lower glycogen levels, impaired glucose and insulin tolerance in the muscles161 and liver.162 Further investigations revealed downregulation in the expression of key genes involved in insulin signaling, along with altered phosphorylation of key insulin signaling molecules.161,162 In addition, increased methylation in the GLUT4 promoter region contributed to the downregulation of this gene in DEHP-exposed offsprings, resulting in a decline in GLUT4 cytosolic protein levels.161 Furthermore, the offsprings had reduced testosterone and estradiol levels and displayed liver and kidney damage.162 DEHP had opposing effects on fasting serum insulin levels in the two studies.161,162 In a separate study, DEHP exposure in pregnant rats resulted in hypermethylation of the liver IR and GLUT2 gene promoters and reduced transcription of the corresponding genes, along with reduced glucose uptake and oxidation in adult offsprings.177 Adolescent rats administered 50 and 500 mg per kg per day DEHP for 28 days displayed higher fasting blood glucose, serum leptin and serum insulin levels as well as insulin resistance.158 These observations were associated with reduced leptin receptor and IR protein levels (500 mg per kg per day DEHP group) in the liver. DEHP further interfered with the Janus-activated kinase 2/signal transducer and activator of transcription 3/suppressor of cytokine signaling 3 (JAK2/STAT3/SOCS3) pathway. Exposure of 3-week-old male rats to 750 mg per kg DEHP for 8 weeks elevated fasting glucose levels, reduced muscle GLUT4 mRNA and protein levels, reduced insulin sensitivity but had no effect on HOMA-IR.168
Some of the earliest in vitro studies documenting the effect of DEHP on glucose metabolism in a liver cell line originated from a study in which Chang liver cells treated with 200 and 400 μM DEHP for 24 hour showed reduced IR levels and glucose oxidation.178 Moreover, experimental data support the proposed mechanism of DEHP inducing insulin resistance via oxidative stress in insulin target cells. Treatment of L6 myotubes with 100 μM DEHP179 or buffalo rat liver cells with 5, 500 and 50
000 nM DEHP180 for 24 hours induced oxidative stress and apoptosis, along with reduced IR and GLUT4 gene expression and protein content, leading to impaired signaling and reduced glucose uptake and oxidation. Treatment of a human preadipocyte cell line with 50 μg per ml DEHP (128 μM) increased ROS levels but had no effect on the protein levels of enzyme antioxidants and GLUT 4 expression.151
In vivo studies found that treatment of adolescent male mice with 180 mg per kg per day DEHP for 3 weeks elevated fasting blood glucose levels and increased levels of HbA1c but did not change HOMA-IR levels.181 Reduced liver IR, IRS-1 and GLUT4 protein levels, decreased levels of proteins that regulate lipid metabolism homeostasis and increased serum triacylglycerol were also observed. In a similar study, female mice exhibited decreased liver antioxidant enzyme activity and increased MDA levels.182 These observations were associated with increased levels of HbA1c and GLUT4 protein, decreased IR and IRS-1 protein levels, while having no effect on fasting glucose, fasting insulin and HOMA-IR levels. Hepatic cellular self-repair was the suggested reason for the increased liver GLUT4 protein levels. In both studies, the DEHP effects were aggravated in adolescent T2D mice compared to normal rats, with the female mice being more sensitive to DEHP that the male mice.181,182 Rats treated with DEHP (10 and 100 mg per kg body weight) for 30 days displayed increased ROS production in adipose131 and muscles tissue,130 resulting in impaired insulin signaling and GLUT4 translocation to the plasma membrane, subsequently leading to decreased glucose uptake and oxidation along with elevated blood glucose levels. Reduced glycogen concentration was also observed.130 Vitamin E and C were able to prevent most of the DEHP-induced effects, confirming the involvement of DEHP in inducing oxidative stress.130,131 In another study, Balb/c mice given 50 and 250 mg per kg per day DEHP for 56 days had increased liver ROS and MDA levels; however, the authors did not further determine whether the oxidative damage was related to insulin resistance.183 A study on metabolomics and transcriptomics analysis of the long-term exposure (12 weeks) exposure effects of 600 mg per kg per day DEHP on the rat liver reported oxidative stress induction, mitochondrial dysfunction, increased gluconeogenesis but inhibited glycolysis, disturbed insulin signaling and insulin resistance pathways, increased expression of apoptotic genes and decreased expression of proliferative genes.160
Zhang et al.134 studied the effect of DEHP on the rat liver (0.05, 5 and 500 mg kg−1) and human hepatocytes cell line L02 (5–100 μmol l−1). In both the rat liver (15 week examination) and L02 cells, DEHP induced oxidative stress (via increasing MDA levels and decreasing SOD activity), increased PPARγ protein expression and reduced IR and GLUT4 protein levels. The rats also exhibited elevated blood glucose, insulin resistance and liver damage while L02 cells showed reduced glucose uptake and translocation of the GLUT4 transporter to the cell membrane. The PPARγ antagonist GW9662 was effective in reducing some of the DEHP-induced effects in L02 cells and the authors concluded that the DEHP-induced oxidative stress and GLUT4-associated changes occurred via a PPARγ-mediated mechanism. Transfections studies conducted in isolated rat adipocytes and CHO-K1 fibroblasts showed that ligand-free PPARγ (in complex with RXR) represses the activity of the GLUT4 gene by binding to its promoter and further suggested that exogenous ligands alleviate this effect while endogenous ligands enhance it.184 However, since the DEHP metabolite MEHP activates PPARγ142,146 it would seem that DEHP exposure in the above studies further enhanced PPARγ's ability to downregulate GLUT4 expression. Thus Zhang et al.134 suggested the decline in the levels of GLUT4 protein following DEHP treatment in their study to be due to an increase in PPARγ expression, since DEHP and MEHP upregulates the expression of PPARγ at both mRNA and protein levels in vitro.185 Conflicting results have since been reported regarding the effect of DEHP/MEHP on PPARγ expression;145,151,152,154,157 however, most of the studies did not examine GLUT4 expression. The same DEHP dose exerted similar144,148 or differential patterns155 of PPARγ gene expression in different tissues, or exerted different effects on PPARγ gene and protein expression in the same tissue.134,154,157
Disturbances in insulin signaling and reduction in the expression of GLUT4 are some of the proposed mechanisms through which oxidative stress leads to insulin resistance in peripheral tissues.66 It would seem that DEHP could downregulate GLUT4 expression via PPARγ-mediated binding to its promoter region, thus leading to insulin resistance.134 While it is generally accepted that PPARγ ligands induce cellular changes via binding to PPARγ, it has been proposed that the ligands may also act via mechanisms that are receptor independent.166 Oxidative stress seems to be a common feature through which DEHP induces its effects in insulin target cells. Therefore, based on the experimental data, it is possible that the induction of oxidative stress and subsequent impaired insulin signaling134 and reduction in the expression of GLUT4 may represent some of mechanisms through which DEHP could contribute to insulin resistance and subsequently, the development of T2D (Fig. 1 bottom panel). While some of the details of the mechanisms involved in DEHP-induced insulin resistance have been elucidated, further studies are warranted for a full comprehension of these mechanisms.
3.4 DEHP and impaired adiponectin expression
Adiponectin is synthesized by adipose tissue and it modulates glucose levels, lipid metabolism and insulin sensitivity in insulin target organs.186,187 Low levels of adiponectin are associated with insulin resistance, obesity and T2D.187–189 Downregulation of adiponectin gene expression, along with increased leptin expression and an increase in body weight and visceral fat tissue, was observed in pregnant mice that were exposed to DEHP.158 Jia et al.155 found that higher leptin and lower adiponectin serum levels in rats exposed to 500 mg per kg per day DEHP were associated with an increase in the body mass index and insulin insensitivity. In another study, DEHP exposure to male and female mice for 10 weeks did not affect glucose tolerance, fasting glucose and insulin levels or cause insulin resistance (based on the HOMA-IR parameter).157 However, impaired insulin tolerance in female mice, based on insulin tolerance test, was reported along with a decrease in circulating adiponectin levels and reduced levels of adiponectin protein in subcutaneous adipose tissue. The authors suggested that DEHP induces adipose tissue dysfunction, which was reflected by the lowered adiponectin levels, and subsequently impaired insulin sensitivity.157 Mice exposed to DEHP in combination with a high fat diet displayed increased fasting insulin levels and low adiponectin levels, which were suggested to contribute to glucose intolerance. The increased levels of PPARγ phosphorylation at serine 273 in adipose tissue observed in the study were suggested to be the reason for the downregulated adiponectin expression.159
In vitro studies showed that 3T3-L1 cells treated with 0.01% DEHP for 48 hours had decreased adiponectin mRNA and protein levels which were associated with reduced lipid content and impaired insulin stimulated glucose uptake.157 Exposure of a human preadipocyte cell line to environmentally relevant levels of DEHP resulted in reduced adiponectin and increased leptin secretion but did not affect GLUT4 mRNA levels151,190 and GLUT4 translocation to the cell membrane.151 This effect was also associated with reduced triacylglyceride content and reduced accumulation of lipid droplets.151 The lack of DEHP's effect on GLUT4 expression and translocation somewhat contradicts the observations that adiponectin protects against insulin resistance and the findings in rat muscle cells where adiponectin enhanced GLUT4 translocation.191 It would also seem that a decline in adiponectin levels is associated with reduced lipid content in these in vitro studies but an increased body weight/mass in the in vivo studies.
In contrast to epidemiological findings, it seems that experimental studies agree that DEHP reduces adiponectin levels. The observation that lowered adiponectin levels did not affect GLUT4 gene expression and GLUT4 translocation to the cell membrane in human preadipocytes is surprising since one would expect an associated decline in these processes. This is an aspect that can perhaps be clarified with more studies in human models. According to a recent study, the mechanism through which DEHP exposure reduced adiponectin concentrations is still being elucidated.61
4 Conclusions
DEHP and its metabolites have relatively short half-lives in the body and thus it is suggested that they do not bioaccumulate. However, DEHP is ubiquitous in the environment and thus prolonged constant exposure, through the use of everyday products, warrants concern about its effects to human health. Experimental data in animals suggest that exposure to DEHP may be an added risk factor for developing diabetes. Furthermore, animal studies on developmental DEHP exposure suggest that DEHP may cause long term imbalances in glucose metabolism that could potentially lead to the development of diabetes. The question of whether DEHP exposure results in adverse effects in humans remains controversial in the scientific community. With that being said, it seems that the approach should be to err on the side of caution and minimize exposure to this chemical. This could involve the development of biomonitoring surveys in developing countries for environmental chemical exposure monitoring, which could then possibly inform regulatory restrictions regarding the use of DEHP. In addition, the reasons for disparities in the associations of DEHP metabolites with insulin resistance and obesity need to be investigated. There is also a need to strengthen efforts in DEHP/DEHP metabolites and diabetes-related research in areas such as longitudinal epidemiological studies and experimental studies in cell models relevant to humans, at environmentally relevant doses.
Data availability
No primary research results have been included and no new data were generated or analysed as part of this review.
Author contributions
Sebolaishi Doris Makhubela: conceptualization, writing – original draft. Ananias Hodi Kgopa: writing – review and editing. Matlou Phineas Mokgotho: writing – review and editing. Leshweni Jerry Shai: conceptualization, writing – review and editing.
Conflicts of interest
There are no conflicts to declare.
Acknowledgements
This work was funded by the Department of Higher Education and Training (South Africa) through the University Capacity Development Grant (UCDG-D428) and the National Research Foundation of South Africa (grant 129079). We would like to thank Marinda Pretorius from the Medical Illustrations and Audio-visual Services Department (Sefako Makgatho Health Sciences University) for her assistance with the illustrations.
References
-
World Health Organization, WHO Global Report on Diabetes, 2016, available from, https://www.who.int/publications/i/item/9789241565257, accessed 26 March 2020 Search PubMed.
- A. T. Kharroubi and H. M. Darwish, Diabetes mellitus: the epidemic of the century, World J. Diabetes, 2015, 6(6), 850–867 CrossRef PubMed.
-
Federation I, IDF Diabetes Atlas, Tenth, International Diabetes, 2021, available at, https://diabetesatlas.org/, accessed 23 January 2024 Search PubMed.
- S. M. Regnier and R. M. Sargis, Adipocytes under assault: environmental disruption of adipose physiology, Biochim. Biophys. Acta, 2014, 1842(3), 520–533 CrossRef CAS PubMed.
- N. Chevalier and P. Fénichel, Endocrine disruptors: new players in the pathophysiology of type 2 diabetes?, Diabetes Metab., 2015, 41(2), 107–115 CrossRef CAS PubMed.
- C. Casals-Casas and B. Desvergne, Endocrine disruptors: from endocrine to metabolic disruption, Annu. Rev. Physiol., 2011, 73, 135–162 CrossRef CAS PubMed.
-
Agency for Toxic Substances and Disease Registry (ATSDR), Toxicological profile for di-(2-ethylhexyl)phthalate, U.S. Department of Health and Human Services, 2002, available from, https://www.atsdr.cdc.gov/ToxProfiles/tp9.pdf, accessed 6 September 2020 Search PubMed.
- R. Kavlock, K. Boekelheide, R. Chapin, M. Cunningham, E. Faustman, P. Foster, M. Golub, R. Henderson, I. Hinberg, R. Little, J. Seed, K. Shea, S. Tabacova, R. Tyl, P. Williams and T. Zacharewski, NTP Center for the Evaluation of Risks to Human Reproduction: phthalates expert panel report on the reproductive and developmental toxicity of di(2-ethylhexyl) phthalate, Reprod. Toxicol., 2002, 16(5), 529–653 CrossRef CAS.
- M. Wormuth, M. Scheringer, M. Vollenweider and K. Hungerbühler, What are the sources of exposure to eight frequently used phthalic acid esters in Europeans?, Risk Anal., 2006, 26(3), 803–824 CrossRef PubMed.
- H. M. Koch, M. Lorber, K. L. Christensen, C. Pälmke, S. Koslitz and T. Brüning, Identifying sources of phthalate exposure with human biomonitoring: results of a 48h fasting study with urine collection and personal activity patterns, Int. J. Hyg Environ. Health, 2013, 216(6), 672–681 CrossRef CAS PubMed.
- S. E. Serrano, J. Braun, L. Trasande, R. Dills and S. Sathyanarayana, Phthalates and diet: a review of the food monitoring and epidemiology data, Environ. Health, 2014, 13(1), 43 CrossRef PubMed.
- D. Xu, X. Deng, E. Fang, X. Zheng, Y. Zhou, L. Lin, L. Chen, M. Wu and Z. Huang, Determination of 23 phthalic acid esters in food by liquid chromatography tandem mass spectrometry, J. Chromatogr. A, 2014, 1324, 49–56 CrossRef CAS.
- C. Bi, J. P. Maestre, H. Li, G. Zhang, R. Givehchi, A. Mahdavi, K. A. Kinney, J. Siegel, S. D. Horner and Y. Xu, Phthalates and organophosphates in settled dust and HVAC filter dust of U.S. low-income homes: association with season, building characteristics, and childhood asthma, Environ. Int., 2018, 121(pt 1), 916–930 CrossRef CAS.
- A. M. Calafat, L. L. Needham, M. J. Silva and G. Lambert, Exposure to di-(2-ethylhexyl) phthalate among premature neonates in a neonatal intensive care unit, Pediatrics, 2004, 113(5), e429–e434 CrossRef.
- J. Weuve, B. N. Sánchez, A. M. Calafat, T. Schettler, R. A. Green, H. Hu and R. Hauser, Exposure to phthalates in neonatal intensive care unit infants: urinary concentrations of monoesters and oxidative metabolites, Environ. Health Perspect., 2006, 114(9), 1424–1431 CrossRef CAS PubMed.
- E. Testai, P. Hartemann, S. C. Rastogi, U. Bernauer, A. Piersma, W. De Jong, H. Gulliksson, R. Sharpe, D. Schubert, E. Rodríguez-Farre and Ms Scientific Committee SCENIHR, The safety of medical devices containing DEHP plasticized PVC or other plasticizers on neonates and other groups possibly at risk (2015 update), Regul. Toxicol. Pharmacol., 2016, 76, 209–210 CrossRef CAS PubMed.
-
M. D. Shelby, NTP-CERHR monograph on the potential human reproductive and developmental effects of di(2-ethylhexyl)phthalate (DEHP), NTP CERHR Mon., 2006, vol. 18 Search PubMed.
- Y. Ito, M. Kamijima and T. Nakajima, Di(2-ethylhexyl) phthalate-induced toxicity and peroxisome proliferator-activated receptor alpha: a review, Environ. Health Prev. Med., 2019, 24(1), 47 CrossRef PubMed.
- Y. Ito, H. Yokota, R. Wang, O. Yamanoshita, G. Ichihara, H. Wang, Y. Kurata, K. Takagi and T. Nakajima, Species differences in the metabolism of di(2-ethylhexyl) phthalate (DEHP) in several organs of mice, rats, and marmosets, Arch. Toxicol., 2005, 79(3), 147–154 CrossRef CAS PubMed.
- K. Kato, M. J. Silva, J. A. Reidy, D. Hurtz 3rd, N. A. Malek, L. L. Needham, H. Nakazawa, D. B. Barr and A. M. Calafat, Mono(2-ethyl-5-hydroxyhexyl) phthalate and mono-(2-ethyl-5-oxohexyl) phthalate as biomarkers for human exposure assessment to di-(2-ethylhexyl) phthalate, Environ. Health Perspect., 2004, 112(3), 327–330 CrossRef CAS PubMed.
- Y. Kurata, F. Makinodan, N. Shimamura and M. Katoh, Metabolism of di (2-ethylhexyl) phthalate (DEHP): comparative study in juvenile and fetal marmosets and rats, J. Toxicol. Sci., 2012, 37(1), 33–49 CrossRef CAS.
- H. M. Koch, H. M. Bolt, R. Preuss and J. Angerer, New metabolites of di(2-ethylhexyl)phthalate (DEHP) in human urine and serum after single oral doses of deuterium-labelled DEHP, Arch. Toxicol., 2005, 79(7), 367–376 CrossRef CAS.
- H. M. Koch, R. Preuss and J. Angerer, Di(2-ethylhexyl)phthalate (DEHP): human metabolism and internal exposure- an update and latest results, Int. J. Androl., 2006, 29(1), 155–165 CrossRef CAS PubMed.
- G. Saravanabhavan, M. Guay, É. Langlois, S. Giroux, J. Murray and D. Haines, Biomonitoring of phthalate metabolites in the Canadian population through the Canadian Health Measures Survey (2007-2009), Int. J. Hyg Environ. Health, 2013, 216(6), 652–661 CrossRef CAS.
- A. R. Zota, A. M. Calafat and T. J. Woodruff, Temporal trends in phthalate exposures: findings from the National Health and Nutrition Examination Survey, 2001-2010, Environ. Health Perspect., 2014, 122(3), 235–241 CrossRef CAS.
- W. Choi, S. Kim, Y. W. Baek, K. Choi, K. Lee, S. Kim, S. D. Yu and K. Choi, Exposure to environmental chemicals among Korean adults-updates from the second Korean National Environmental Health Survey (2012-2014), Int. J. Hyg Environ. Health, 2017, 220(2 pt A), 29–35 CrossRef CAS PubMed.
- A. T. Mannetje, J. Coakley and J. Douwes, Levels and determinants of urinary phthalate metabolites in New Zealand children and adults, Int. J. Hyg Environ. Health, 2021, 238, 113853 CrossRef PubMed.
- G. Schwedler, E. Rucic, R. Lange, A. Conrad, H. M. Koch, C. Pälmke, T. Brüning, C. Schulz, M. I. H. Schmied-Tobies, A. Daniels and M. Kolossa-Gehring, Phthalate metabolites in urine of children and adolescents in Germany. Human biomonitoring results of the German Environmental Survey GerES V, 2014-2017, Int. J. Hyg Environ. Health, 2020, 225, 113444 CrossRef CAS.
- R. Dong, S. Zhao, H. Zhang, J. Chen, M. Zhang, M. Wang, M. Wu, S. Li and B. Chen, Sex Differences in the Association of Urinary Concentrations of Phthalates Metabolites with Self-Reported Diabetes and Cardiovascular Diseases in Shanghai Adults, Int. J. Environ. Res. Public Health, 2017, 14(6), 598 CrossRef PubMed.
- I. Lee, Y. J. Park, M. J. Kim, S. Kim, S. Choi, J. Park, Y. H. Cho, S. Hong, J. Yoo, H. Park, G. J. Cheon, K. Choi and M. K. Moon, Associations of urinary concentrations of phthalate metabolites, bisphenol A, and parabens with obesity and diabetes mellitus in a Korean adult population: Korean National Environmental Health Survey (KoNEHS) 2015-2017, Environ. Int., 2021, 146, 106227 CrossRef CAS.
- K. M. Main, G. K. Mortensen, M. M. Kaleva, K. A. Boisen, I. N. Damgaard, M. Chellakooty, I. M. Schmidt, A. M. Suomi, H. E. Virtanen, D. V. Petersen, A. M. Andersson, J. Toppari and N. E. Skakkebaek, Human breast milk contamination with phthalates and alterations of endogenous reproductive hormones in infants three months of age, Environ. Health Perspect., 2006, 114(2), 270–276 CrossRef CAS PubMed.
- M. J. Silva, J. A. Reidy, A. R. Herbert, J. L. Preau Jr, L. L. Needham and A. M. Calafat, Detection of phthalate metabolites in human amniotic fluid, Bull. Environ. Contam. Toxicol., 2004, 72(6), 1226–1231 CrossRef CAS.
-
European Commission, 1999/815/EC: Commission Decision of 7 December 1999 adopting measures prohibiting the placing on the market of toys and childcare articles intended to be placed in the mouth by children under three years of age made of soft PVC containing one or more of the substances di-iso-nonyl phthalate (DINP), di(2-ethylhexyl)phthalate (DEHP), dibutyl phthalate (DBP), di-iso-decyl phthalate (DIDP), di-n-octyl phthalate (DNOP), and butylbenzyl phthalate (BBP) (notified under document number C (1999) 4436) (text with EEA relevance), available from, https://data.europa.eu/eli/dec/1999/815/oj, accessed 25 May, 2021 Search PubMed.
- Consumer Product Safety Improvement Act (CPSIA) Public Law 110–314, 122 STAT 3016–3077, 2008, available from, https://www.cpsc.gov/s3fs-public/cpsia.pdf, accessed 25 May 2021.
-
Canadian Consumer Product Safety Act, Phthalates Regulations SOR/2010-298, 2010, available from, https://laws-lois.justice.gc.ca/eng/regulations/SOR-2010-298/20110620/P1TT3xt3.html, accessed 10 June 2021 Search PubMed.
- G. Tranfo, L. Caporossi, D. Pigini, S. Capanna, B. Papaleo and E. Paci, Temporal Trends of Urinary Phthalate Concentrations in Two Populations: Effects of REACH Authorization after Five Years, Int. J. Environ. Res. Public Health, 2018, 15(9), 1950 CrossRef.
- Y. Wang, H. Zhu and K. Kannan, A Review of Biomonitoring of Phthalate Exposures, Toxics, 2019, 7(2), 21 CrossRef CAS PubMed.
- S. S. S. Rowdhwal and J. Chen, Toxic Effects of Di-2-ethylhexyl Phthalate: An Overview, BioMed Res. Int., 2018, 2018, 1750368 Search PubMed.
- H. Zhang, Y. Ben, Y. Han, Y. Zhang, Y. Li and X. Chen, Phthalate exposure and risk of diabetes mellitus: implications from a systematic review and meta-analysis, Environ. Res., 2022, 204(pt B), 112109 CrossRef CAS.
- M. Mariana and E. Cairrao, The Relationship between Phthalates and Diabetes: A Review, Metabolites, 2023, 13(6), 746 CrossRef CAS PubMed.
- E. G. Radke, A. Galizia, K. A. Thayer and G. S. Cooper, Phthalate exposure and metabolic effects: a systematic review of the human epidemiological evidence, Environ. Int., 2019, 132, 104768 CrossRef CAS PubMed.
- H. Gao, D. Chen and M. Zang, Association between phthalate exposure and insulin resistance: a systematic review and meta-analysis update, Environ. Sci. Pollut. Res. Int., 2021, 28(40), 55967–55980 CrossRef CAS PubMed.
- M. Goodman, J. S. Lakind and D. R. Mattison, Do phthalates act as obesogens in humans? a systematic review of the epidemiological literature, Crit. Rev. Toxicol., 2014, 44(2), 151–175 CrossRef CAS PubMed.
- C. Ribeiro, V. Mendes, B. Peleteiro, I. Delgado, J. Araújo, M. Aggerbeck, I. Annesi-Maesano, D. Sarigiannis and E. Ramos, Association between the exposure to phthalates and adiposity: a meta-analysis in children and adults, Environ. Res., 2019, 179(pt A), 108780 CrossRef CAS PubMed.
- R. E. Dales, L. M. Kauri and S. Cakmak, The associations between phthalate exposure and insulin resistance, β-cell function and blood glucose control in a population-based sample, Sci. Total Environ., 2018, 612, 1287–1292 CrossRef CAS.
- T. James-Todd, R. Stahlhut, J. D. Meeker, S. G. Powell, R. Hauser, T. Huang and J. Rich-Edwards, Urinary phthalate
metabolite concentrations and diabetes among women in the National Health and Nutrition Examination Survey (NHANES) 2001-2008, Environ. Health Perspect., 2012, 120(9), 1307–1313 CrossRef PubMed.
- I. Lee, Y. J. Park, M. J. Kim, S. Kim, S. Choi, J. Park, Y. H. Cho, S. Hong, J. Yoo, H. Park, G. J. Cheon, K. Choi and M. K. Moon, Associations of urinary concentrations of phthalate metabolites, bisphenol A, and parabens with obesity and diabetes mellitus in a Korean adult population: Korean National Environmental Health Survey (KoNEHS) 2015-2017, Environ. Int., 2021, 146, 106227 CrossRef CAS PubMed.
- K. Svensson, R. U. Hernández-Ramírez, A. Burguete-García, M. E. Cebrián, A. M. Calafat, L. L. Needham, L. Claudio and L. López-Carrillo, Phthalate exposure associated with self-reported diabetes among Mexican women, Environ. Res., 2011, 111(6), 792–796 CrossRef CAS PubMed.
- Q. Sun, M. C. Cornelis, M. K. Townsend, D. K. Tobias, A. H. Eliassen, A. A. Franke, R. Hauser and F. B. Hu, Association of urinary concentrations of bisphenol A and phthalate metabolites with risk of type 2 diabetes: a prospective investigation in the Nurses' Health Study (NHS) and NHSII cohorts, Environ. Health Perspect., 2014, 122(6), 616–623 CrossRef PubMed.
- P. M. Lind, B. Zethelius and L. Lind, Circulating levels of phthalate metabolites are associated with prevalent diabetes in the elderly, Diabetes Care, 2012, 35(7), 1519–1524 CrossRef CAS PubMed.
- Y. Duan, H. Sun, L. Han and L. Chen, Association between phthalate exposure and glycosylated hemoglobin, fasting glucose, and type 2 diabetes mellitus: a case-control study in China, Sci. Total Environ., 2019, 670, 41–49 CrossRef CAS PubMed.
- D. J. Nam, Y. Kim, E. H. Yang, H. C. Lee and J. H. Ryoo, Relationship between urinary phthalate metabolites and diabetes: Korean National Environmental Health Survey (KoNEHS) cycle 3 (2015-2017), Ann. Occup. Environ. Med., 2020, 32, e34 CrossRef PubMed.
- X. K. Liu, S. W. Si, Y. Ye, J. Y. Li, H. H. Lyu, Y. M. Ma, C. Y. Zou, H. J. Sun, L. Xue, W. Xu, H. F. Geng and J. Liang, The Link between Exposure to Phthalates and Type 2 Diabetes Mellitus: A Study Based on NHANES Data and Bioinformatic Analysis, Biomed. Environ. Sci., 2023, 36(9), 892–896 Search PubMed.
- R. W. Stahlhut, E. van Wijngaarden, T. D. Dye, S. Cook and S. H. Swan, Concentrations of urinary phthalate metabolites are associated with increased waist circumference and insulin resistance in adult U.S. males, Environ. Health Perspect., 2007, 115(6), 876–882 CrossRef CAS PubMed.
- S. Y. Chen, J. S. Hwang, F. C. Sung, C. Y. Lin, C. J. Hsieh, P. C. Chen and T. C. Su, Mono-2-ethylhexyl phthalate associated with insulin resistance and lower testosterone levels in a young population, Environ. Pollut., 2017, 225, 112–117 CrossRef CAS PubMed.
- S. H. Hong, Y. A. Sung, Y. S. Hong, E. Ha, K. Jeong, H. Chung and H. Lee, Urinary bisphenol A is associated with insulin resistance and obesity in reproductive-aged women, Clin. Endocrinol., 2017, 86(4), 506–512 CrossRef CAS PubMed.
- E. Dirinck, A. C. Dirtu, T. Geens, A. Covaci, L. Van Gaal and P. G. Jorens, Urinary phthalate metabolites are associated with insulin resistance in obese subjects, Environ. Res., 2015, 137, 419–423 CrossRef CAS PubMed.
- S. H. Kim, J. W. On, H. Pyo, K. S. Ko, J. C. Won, J. Yang and M. J. Park, Percentage fractions of urinary di(2-ethylhexyl) phthalate metabolites: association with obesity and insulin resistance in Korean girls, PLoS One, 2018, 13(11), e0208081 CrossRef PubMed.
- L. Trasande, A. J. Spanier, S. Sathyanarayana, T. M. Attina and J. Blustein, Urinary phthalates and increased insulin resistance in adolescents, Pediatrics, 2013, 132(3), e646–e655 CrossRef PubMed.
- T. M. Attina and L. Trasande, Association of Exposure to Di-2-Ethylhexylphthalate Replacements With Increased Insulin Resistance in Adolescents From NHANES 2009-2012, J. Clin. Endocrinol. Metabol., 2015, 100(7), 2640–2650 CrossRef CAS PubMed.
- C. Y. Lin, H. L. Lee, C. W. Chen, C. Wang, F. C. Sung and T. C. Su, The role of angiotensin I-converting enzyme gene polymorphism and global DNA methylation in the negative associations between urine di-(2-ethylhexyl) phthalate metabolites and serum adiponectin in a young Taiwanese population, Clin. Epigenet., 2023, 15(1), 87 CrossRef CAS PubMed.
- T. Huang, A. R. Saxena, E. Isganaitis and T. James-Todd, Gender and racial/ethnic differences in the associations of urinary phthalate metabolites with markers of diabetes risk: National Health and Nutrition Examination Survey 2001-2008, Environ. Health, 2014, 13(1), 6 CrossRef PubMed.
- T. M. James-Todd, T. Huang, E. W. Seely and A. R. Saxena, The association between phthalates and metabolic syndrome: the National Health and Nutrition Examination Survey 2001-2010, Environ. Health, 2016, 15, 52 CrossRef PubMed.
- I. Lee, S. Kim, S. Park, S. Mok, Y. Jeong, H. B. Moon, J. Lee, S. Kim, H. J. Kim, G. Choi, S. Choi, S. Y. Kim, A. Lee, J. Park and K. Choi, Association of urinary phthalate metabolites and phenolics with adipokines and insulin resistance related markers among women of reproductive age, Sci. Total Environ., 2019, 688, 1319–1326 CrossRef CAS PubMed.
- K. N. Keane, V. F. Cruzat, R. Carlessi, P. I. de Bittencourt Jr and P. Newsholme, Molecular Events Linking Oxidative Stress and Inflammation to Insulin Resistance and β-Cell Dysfunction, Oxid. Med. Cell. Longev., 2015, 2015, 181643 Search PubMed.
- H. Yaribeygi, T. Sathyapalan, S. L. Atkin and A. Sahebkar, Molecular Mechanisms Linking Oxidative Stress and Diabetes Mellitus, Oxid. Med. Cell. Longev., 2020, 2020, 8609213 Search PubMed.
- K. K. Ferguson, T. F. McElrath, Y. H. Chen, B. Mukherjee and J. D. Meeker, Urinary phthalate metabolites and biomarkers of oxidative stress in pregnant women: a repeated measures analysis, Environ. Health Perspect., 2015, 123(3), 210–216 CrossRef.
- Y. C. Hong, E. Y. Park, M. S. Park, J. A. Ko, S. Y. Oh, H. Kim, K. H. Lee, J. H. Leem and E. H. Ha, Community level exposure to chemicals and oxidative stress in adult population, Toxicol. Lett., 2009, 184(2), 139–144 CrossRef CAS PubMed.
- K. K. Ferguson, R. Loch-Caruso and J. D. Meeker, Urinary phthalate metabolites in relation to biomarkers of inflammation and oxidative stress: NHANES 1999-2006, Environ. Res., 2011, 111(5), 718–726 CrossRef CAS.
- A. Kataria, D. Levine, S. Wertenteil, S. Vento, J. Xue, K. Rajendiran, K. Kannan, J. M. Thurman, D. Morrison, R. Brody, E. Urbina, T. Attina, L. Trasande and H. Trachtman, Exposure to bisphenols and phthalates and association with oxidant stress, insulin resistance, and endothelial dysfunction in children, Pediatr. Res., 2017, 81(6), 857–864 CrossRef CAS PubMed.
- N. Holland, K. Huen, V. Tran, K. Street, B. Nguyen, A. Bradman and B. Eskenazi, Urinary Phthalate Metabolites and Biomarkers of Oxidative Stress in a Mexican-American Cohort: Variability in Early and Late Pregnancy, Toxics, 2016, 4(1), 7 CrossRef.
- A. G. Asimakopoulos, J. Xue, B. P. De Carvalho, A. Iyer, K. O. Abualnaja, S. S. Yaghmoor, T. A. Kumosani and K. Kannan, Urinary biomarkers of exposure to 57 xenobiotics and its association with oxidative stress in a population in Jeddah, Saudi Arabia, Environ. Res., 2016, 150, 573–581 CrossRef CAS PubMed.
- I. Lee, R. Alakeel, S. Kim, Y. A. Al-Sheikh, H. Al-Mandeel, A. A. Alyousef, Y. Kho and K. Choi, Urinary phthalate metabolites among children in Saudi Arabia: occurrences, risks, and their association with oxidative stress markers, Sci. Total Environ., 2019, 654, 1350–1357 CrossRef CAS PubMed.
- B. A. Rocha, A. G. Asimakopoulos, F. Barbosa Jr and K. Kannan, Urinary concentrations of 25 phthalate metabolites in Brazilian children and their association with oxidative DNA damage, Sci. Total Environ., 2017, 586, 152–162 CrossRef CAS PubMed.
- V. Tran, G. Tindula, K. Huen, A. Bradman, K. Harley, K. Kogut, A. M. Calafat, B. Nguyen, K. Parra, X. Ye, B. Eskenazi and N. Holland, Prenatal phthalate exposure and 8-isoprostane among Mexican-American children with high prevalence of obesity, J. Dev. Origins Health Dis., 2017, 8(2), 196–205 CrossRef CAS.
- J. H. Kim, H. Y. Park, S. Bae, Y. H. Lim and Y. C. Hong, Diethylhexyl phthalates is associated with insulin resistance via oxidative stress in the elderly: a panel study, PLoS One, 2013, 8(8), e71392 CrossRef.
- Y. Duan, L. Wang, L. Han, B. Wang, H. Sun, L. Chen, L. Zhu and Y. Luo, Exposure to phthalates in patients with diabetes and its association with oxidative stress, adiponectin, and inflammatory cytokines, Environ. Int., 2017, 109, 53–63 CrossRef CAS PubMed.
- T. Kadowaki, T. Yamauchi, N. Kubota, K. Hara, K. Ueki and K. Tobe, Adiponectin and adiponectin receptors in insulin resistance, diabetes, and the metabolic syndrome, J. Clin. Invest., 2006, 116(7), 1784–1792 CrossRef CAS.
- J. J. Heindel, B. Blumberg, M. Cave, R. Machtinger, A. Mantovani, M. A. Mendez, A. Nadal, P. Palanza, G. Panzica, R. Sargis, L. N. Vandenberg and F. Vom Saal, Metabolism disrupting chemicals and metabolic disorders, Reprod. Toxicol., 2017, 68, 3–33 CrossRef CAS PubMed.
- B. A. Neel and R. M. Sargis, The paradox of progress: environmental disruption of metabolism and the diabetes epidemic, Diabetes, 2011, 60(7), 1838–1848 CrossRef CAS PubMed.
- J. W. Hou, C. L. Lin, Y. A. Tsai, C. H. Chang, K. W. Liao, C. J. Yu, W. Yang, M. J. Lee, P. C. Huang, C. W. Sun, Y. H. Wang, F. R. Lin, W. C. Wu, M. C. Lee, W. H. Pan, B. H. Chen, M. T. Wu, C. C. Chen, S. L. Wang, C. C. Lee, C. A. Hsiung and M. L. Chen, The effects of phthalate and nonylphenol exposure on body size and secondary sexual characteristics during puberty, Int. J. Hyg Environ. Health, 2015, 218(7), 603–615 CrossRef CAS PubMed.
- E. E. Hatch, J. W. Nelson, M. M. Qureshi, J. Weinberg, L. L. Moore, M. Singer and T. F. Webster, Association of urinary phthalate metabolite concentrations with body mass index and waist circumference: a cross-sectional study of NHANES data, 1999-2002, Environ. Health, 2008, 7, 27 CrossRef.
- L. Yaghjyan, S. Sites, Y. Ruan, S.-H. Chang, L. Yaghjyan, S. Sites, Y. Ruan and S. H. Chang, Associations of urinary phthalates with body mass index, waist circumference and serum lipids among females: National Health and Nutrition Examination Survey 1999-2004, Int. J. Obes., 2015, 39(6), 994–1000 CrossRef CAS PubMed.
- M. C. Buser, H. E. Murray and F. Scinicariello, Age and sex differences in childhood and adulthood obesity association with phthalates: analyses of NHANES 2007-2010, Int. J. Hyg Environ. Health, 2014, 217(6), 687–694 CrossRef PubMed.
- M. V. D. Santana, S. E. Hankinson, C. Bigelow, S. R. Sturgeon, R. T. Zoeller, L. Tinker, J. A. E. Manson, A. M. Calafat, J. R. Meliker and K. W. Reeves, Urinary concentrations of phthalate biomarkers and weight change among postmenopausal women: a prospective cohort study, Environ. Health, 2019, 18(1), 20 CrossRef PubMed.
- R. Dong, T. Zhou, J. Chen, M. Zhang, H. Zhang, M. Wu, S. Li, L. Zhang and B. Chen, Gender- and Age-Specific Relationships Between Phthalate Exposures and Obesity in Shanghai Adults, Arch. Environ. Contam. Toxicol., 2017, 73(3), 431–441 CrossRef CAS.
- Y. Song, R. Hauser, F. B. Hu, A. A. Franke, S. Liu and Q. Sun, Urinary concentrations of bisphenol A and phthalate metabolites and weight change: a prospective investigation in US women, Int. J. Obes., 2014, 38(12), 1532–1537 CrossRef CAS PubMed.
- J. E. Lim, B. Choi and S. H. Jee, Urinary bisphenol A, phthalate metabolites, and obesity: do gender and menopausal status matter?, Environ. Sci. Pollut. Res. Int., 2020, 27(27), 34300–34310 CrossRef CAS PubMed.
- Y. L. Li, J. Lv, Z. P. Du, S. Feng, J. Sheng, Z. X. Jin, K. Y. Liu, H. Gao, X. D. Li, H. J. Cao, L. S. Yang, D. X. Xu, F. B. Tao and Q. N. Wang, The levels of phthalate exposure and associations with obesity in an elderly population in China, Ecotoxicol. Environ. Saf., 2020, 201, 110749 CrossRef CAS PubMed.
- T. P. van der Meer, M. van Faassen, A. P. van Beek, H. Snieder, I. P. Kema, B. H. R. Wolffenbuttel and J. V. van Vliet-Ostaptchouk, Exposure to Endocrine Disrupting Chemicals in the Dutch general population is associated with adiposity-related traits, Sci. Rep., 2020, 10(1), 9311 CrossRef CAS PubMed.
- L. Trasande, T. M. Attina, S. Sathyanarayana, A. J. Spanier and J. Blustein, Race/ethnicity-specific associations of urinary phthalates with childhood body mass in a nationally representative sample, Environ. Health Perspect., 2013, 121(4), 501–506 CrossRef PubMed.
- Z. H. Wang, D. Gao and Z. Y. Zou, The association of phthalate metabolites with childhood waist circumference and abdominal obesity, Eur. J. Pediatr., 2023, 182(2), 803–812 CrossRef CAS.
- B. Xia, Q. Zhu, Y. Zhao, W. Ge, Y. Zhao, Q. Song, Y. Zhou, H. Shi and Y. Zhang, Phthalate exposure and childhood overweight and obesity: urinary metabolomic evidence, Environ. Int., 2018, 121(pt 1), 159–168 CrossRef CAS.
- H. Wang, Y. Zhou, C. Tang, Y. He, J. Wu, Y. Chen and Q. Jiang, Urinary phthalate metabolites are associated with body mass index and waist circumference in Chinese school children, PLoS One, 2013, 8(2), e56800 CrossRef CAS.
- Y. Zhang, X. Meng, L. Chen, D. Li, L. Zhao, Y. Zhao, L. Li and H. Shi, Age and sex-specific relationships between phthalate exposures and obesity in Chinese children at puberty, PLoS One, 2014, 9(8), e104852 CrossRef.
- M. M. Amin, S. Parastar, K. Ebrahimpour, B. Shoshtari-Yeganeh, M. Hashemi, M. Mansourian and R. Kelishadi, Association of urinary phthalate metabolites concentrations with body mass index and waist circumference, Environ. Sci. Pollut. Res. Int., 2018, 25(11), 11143–11151 CrossRef CAS PubMed.
- M. Y. Seo, S. Moon, S. H. Kim and M. J. Park, Associations of Phthalate Metabolites and Bisphenol A Levels with Obesity in Children: The Korean National Environmental Health Survey (KoNEHS) 2015 to 2017, Endocrinol. Metabol., 2022, 37(2), 249–260 CrossRef CAS.
- Y. Dong, D. Gao, Y. Li, Z. Yang, X. Wang, M. Chen, Z. Wang, Y. Song, Z. Zou and J. Ma, Effect of childhood phthalates exposure on the risk of overweight and obesity: a nested case-control study in China, Environ. Int., 2022, 158, 106886 CrossRef CAS PubMed.
- S. L. Teitelbaum, N. Mervish, E. L. Moshier, N. Vangeepuram, M. P. Galvez, A. M. Calafat, M. J. Silva, B. L. Brenner and M. S. Wolff, Associations between phthalate metabolite urinary concentrations and body size measures in New York City children, Environ. Res., 2012, 112, 186–193 CrossRef CAS PubMed.
- A. L. Deierlein, M. S. Wolff, A. Pajak, S. M. Pinney, G. C. Windham, M. P. Galvez, M. J. Silva, A. M. Calafat, L. H. Kushi, F. M. Biro, S. L. Teitelbaum and Breast Cancer and Environment Research Program, Longitudinal Associations of Phthalate Exposures During Childhood and Body Size Measurements in Young Girls, Epidemiology, 2016, 27(4), 492–499 CrossRef PubMed.
- A. Zettergren, N. Andersson, K. Larsson, I. Kull, E. Melén, A. Georgelis, M. Berglund, C. Lindh and A. Bergström, Exposure to environmental phthalates during preschool age and obesity from childhood to young adulthood, Environ. Res., 2021, 192, 110249 CrossRef CAS PubMed.
- K. Christensen, J. Sobus, M. Phillips, T. Blessinger, M. Lorber and Y. M. Tan, Changes in epidemiologic associations with different exposure metrics: a case study of phthalate exposure associations with body mass index and waist circumference, Environ. Int., 2014, 73, 66–76 CrossRef CAS PubMed.
- J. S. LaKind, M. Goodman and D. Q. Naiman, Use of NHANES data to link chemical exposures to chronic diseases: a cautionary tale, PLoS One, 2012, 7(12), e51086 CrossRef CAS PubMed.
- J. D. Peck, A. M. Sweeney, E. Symanski, J. Gardiner, M. J. Silva, A. M. Calafat and S. L. Schantz, Intra- and inter-individual variability of urinary phthalate metabolite concentrations in Hmong women of reproductive age, J. Expo. Sci. Environ. Epidemiol., 2010, 20(1), 90–100 CrossRef CAS PubMed.
- J. Li, W. Xia, C. Wu, H. Zhao, Y. Zhou, J. Wei, F. Ji, H. Luan, S. Xu and Z. Cai, Variations of phthalate exposure and metabolism over three trimesters, Environ. Pollut., 2019, 251, 137–145 CrossRef CAS PubMed.
- J. D. Meeker, S. Sathyanarayana and S. H. Swan, Phthalates and other additives in plastics: human exposure and associated health outcomes, Philos. Trans. R. Soc. London, Ser. B, 2009, 364(1526), 2097–2113 CrossRef CAS PubMed.
- J. J. Adibi, R. M. Whyatt, P. L. Williams, A. M. Calafat, D. Camann, R. Herrick, H. Nelson, H. K. Bhat, F. P. Perera, M. J. Silva and R. Hauser, Characterization of phthalate exposure among pregnant women assessed by repeat air and urine samples, Environ. Health Perspect., 2008, 116(4), 467–473 CrossRef CAS PubMed.
- J. M. Braun, K. W. Smith, P. L. Williams, A. M. Calafat, K. Berry, S. Ehrlich and R. Hauser, Variability of urinary phthalate metabolite and bisphenol A concentrations before and during pregnancy, Environ. Health Perspect., 2012, 120(5), 739–745 CrossRef CAS PubMed.
- D. E. Cantonwine, J. F. Cordero, L. O. Rivera-González, L. V. Anzalota Del Toro, K. K. Ferguson, B. Mukherjee, A. M. Calafat, N. Crespo, B. Jiménez-Vélez, I. Y. Padilla, A. N. Alshawabkeh and J. D. Meeker, Urinary phthalate metabolite concentrations among pregnant women in Northern Puerto Rico: distribution, temporal variability, and predictors, Environ. Int., 2014, 62, 1–11 CrossRef CAS PubMed.
- K. K. Ferguson, T. F. McElrath, Y. A. Ko, B. Mukherjee and J. D. Meeker, Variability in urinary phthalate metabolite levels across pregnancy and sensitive windows of exposure for the risk of preterm birth, Environ. Int., 2014, 70, 118–124 CrossRef PubMed.
- M. Fisher, T. E. Arbuckle, R. Mallick, A. LeBlanc, R. Hauser, M. Feeley, D. Koniecki, T. Ramsay, G. Provencher, R. Bérubé and M. Walker, Bisphenol A and phthalate metabolite urinary concentrations: daily and across pregnancy variability, J. Expo. Sci. Environ. Epidemiol., 2015, 25(3), 231–239 CrossRef CAS.
- D. Valvi, N. Monfort, R. Ventura, M. Casas, L. Casas, J. Sunyer and M. Vrijheid, Variability and predictors of urinary phthalate metabolites in Spanish pregnant women, Int. J. Hyg Environ. Health, 2015, 218(2), 220–231 CrossRef CAS.
- T. M. James-Todd, J. D. Meeker, T. Huang, R. Hauser, E. W. Seely, K. K. Ferguson, J. W. Rich-Edwards and T. F. McElrath, Racial and ethnic variations in phthalate metabolite concentration changes across full-term pregnancies, J. Expo. Sci. Environ. Epidemiol., 2017, 27(2), 160–166 CrossRef CAS PubMed.
- S. Siddiq, A. M. Clemons, J. D. Meeker, C. Gennings, V. Rauh, S. H. Leisher, A. A. M. Llanos, J. A. McDonald, B. J. Wylie and P. Factor-Litvak, Predictors of Phthalate Metabolites Exposure among Healthy Pregnant Women in the United States, 2010-2015, Int. J. Environ. Res. Public Health, 2023, 20(23), 7104 CrossRef CAS.
- M. Casas, X. Basagaña, A. K. Sakhi, L. S. Haug, C. Philippat, B. Granum, C. B. Manzano-Salgado, C. Brochot, F. Zeman, J. de Bont, S. Andrusaityte, L. Chatzi, D. Donaire-Gonzalez, L. Giorgis-Allemand, J. R. Gonzalez, E. Gracia-Lavedan, R. Grazuleviciene, M. Kampouri, S. Lyon-Caen, P. Pañella, I. Petraviciene, O. Robinson, J. Urquiza, M. Vafeiadi, C. Vernet, D. Waiblinger, J. Wright, C. Thomsen, R. Slama and M. Vrijheid, Variability of urinary concentrations of non-persistent chemicals in pregnant women and school-aged children, Environ. Int., 2018, 121(pt 1), 561–573 CrossRef CAS.
- J. L. Preau Jr, L. Y. Wong, M. J. Silva, L. L. Needham and A. M. Calafat, Variability over 1 week in the urinary concentrations of metabolites of diethyl phthalate and di(2-ethylhexyl) phthalate among eight adults: an observational study, Environ. Health Perspect., 2010, 118(12), 1748–1754 CrossRef.
- H. Frederiksen, S. K. Kranich, N. Jørgensen, O. Taboureau, J. H. Petersen and A. M. Andersson, Temporal variability in urinary phthalate metabolite excretion based on spot, morning, and 24-h urine samples: considerations for epidemiological studies, Environ. Sci. Technol., 2013, 47(2), 958–967 CrossRef CAS.
- H. Fromme, G. Bolte, H. M. Koch, J. Angerer, S. Boehmer, H. Drexler, R. Mayer and B. Liebl, Occurrence and daily variation of phthalate metabolites in the urine of an adult population, Int. J. Hyg Environ. Health, 2007, 210(1), 21–33 CrossRef CAS PubMed.
- J. D. Meeker, A. M. Calafat and R. Hauser, Urinary phthalate metabolites and their biotransformation products: predictors and temporal variability among men and women, J. Expo. Sci. Environ. Epidemiol., 2012, 22(4), 376–385 CrossRef CAS PubMed.
- L. Dewalque, C. Pirard, S. Vandepaer and C. Charlier, Temporal variability of urinary concentrations of phthalate metabolites, parabens and benzophenone-3 in a Belgian adult population, Environ. Res., 2015, 142, 414–423 CrossRef CAS PubMed.
- M. Bastiaensen, G. Malarvannan, C. Gys, Y. A. Bamai, A. Araki and A. Covaci, Between- and within-individual variability of urinary phthalate and alternative plasticizer metabolites in spot, morning void and 24-h pooled urine samples, Environ. Res., 2020, 191, 110248 CrossRef CAS PubMed.
- S. L. Teitelbaum, J. A. Britton, A. M. Calafat, X. Ye, M. J. Silva, J. A. Reidy, M. P. Galvez, B. L. Brenner and M. S. Wolff, Temporal variability in urinary concentrations of phthalate metabolites, phytoestrogens and phenols among minority children in the United States, Environ. Res., 2008, 106(2), 257–269 CrossRef CAS PubMed.
- D. J. Watkins, M. Eliot, S. Sathyanarayana, A. M. Calafat, K. Yolton, B. P. Lanphear and J. M. Braun, Variability and predictors of urinary concentrations of phthalate metabolites during early childhood, Environ. Sci. Technol., 2014, 48(15), 8881–8890 CrossRef CAS PubMed.
- M. K. Townsend, A. A. Franke, X. Li, F. B. Hu and A. H. Eliassen, Within-person reproducibility of urinary bisphenol A and phthalate metabolites over a 1 to 3 year period among women in the Nurses' Health Studies: a prospective cohort study, Environ. Health, 2013, 12(1), 80 CrossRef PubMed.
- J. A. Hoppin, J. W. Brock, B. J. Davis and D. D. Baird, Reproducibility of urinary phthalate metabolites in first morning urine samples, Environ. Health Perspect., 2002, 110(5), 515–518 CrossRef CAS PubMed.
- R. Hauser, J. D. Meeker, S. Park, M. J. Silva and A. M. Calafat, Temporal variability of urinary phthalate metabolite levels in men of reproductive age, Environ. Health Perspect., 2004, 112(17), 1734–1740 CrossRef CAS.
- M. M. Yazdy, B. A. Coull, J. C. Gardiner, A. Aguiar, A. M. Calafat, X. Ye, S. L. Schantz and S. A. Korrick, A possible approach to improving the reproducibility of urinary concentrations of phthalate metabolites and phenols during pregnancy, J. Expo. Sci. Environ. Epidemiol., 2018, 28(5), 448–460 CrossRef.
- J. S. LaKind, F. Idri, D. Q. Naiman and M. A. Verner, Biomonitoring and Nonpersistent Chemicals-Understanding and Addressing Variability and Exposure Misclassification, Curr. Environ. Health Rep., 20193, 6(1), 16–21 CrossRef CAS.
- Y. Lin, J. Wei, Y. Li, J. Chen, Z. Zhou, L. Song, Z. Wei, Z. Lv, X. Chen, W. Xia and S. Xu, Developmental exposure to di(2-ethylhexyl) phthalate impairs endocrine pancreas and leads to long-term adverse effects on glucose homeostasis in the rat, Am. J. Physiol. Endocrinol. Metab., 2011, 301(3), E527–E538 CrossRef CAS.
- C. Srinivasan, A. I. Khan, V. Balaji, J. Selvaraj and K. Balasubramanian, Diethyl hexyl phthalate-induced changes in insulin signaling molecules and the protective role of antioxidant vitamins in gastrocnemius muscle of adult male rat, Toxicol. Appl. Pharmacol., 2011, 257(2), 155–164 CrossRef CAS.
- P. Rajesh, S. Sathish, C. Srinivasan, J. Selvaraj and K. Balasubramanian, Phthalate is associated with insulin resistance in adipose tissue of male rat: role of antioxidant vitamins, J. Cell. Biochem., 2013, 114(3), 558–569 CrossRef CAS PubMed.
- P. Rajesh and K. Balasubramanian, Gestational exposure to di(2-ethylhexyl) phthalate (DEHP) impairs pancreatic β-cell function in F1 rat offspring, Toxicol. Lett., 2015, 232(1), 46–57 CrossRef CAS PubMed.
- X. Sun, Y. Lin, Q. Huang, J. Shi, L. Qiu, M. Kang, Y. Chen, C. Fang, T. Ye and S. Dong, Di(2-ethylhexyl) phthalate-induced apoptosis in rat INS-1 cells is dependent on activation of endoplasmic reticulum stress and suppression of antioxidant protection, J. Cell Mol. Med., 2015, 19(3), 581–594 CrossRef CAS PubMed.
- W. Zhang, X. Y. Shen, W. W. Zhang, H. Chen, W. P. Xu and W. Wei, Di-(2-ethylhexyl) phthalate could disrupt the insulin signaling pathway in liver of SD rats and L02 cells via PPARγ, Toxicol. Appl. Pharmacol., 2017, 316, 17–26 CrossRef CAS PubMed.
- G. Karabulut and N. Barlas, The possible effects of mono butyl phthalate (MBP) and mono (2-ethylhexyl) phthalate (MEHP) on INS-1 pancreatic beta cells, Toxicol. Res., 2021, 10(3), 601–612 CrossRef PubMed.
- J. N. Feige, L. Gelman, D. Rossi, V. Zoete, R. Métivier, C. Tudor, S. I. Anghel, A. Grosdidier, C. Lathion, Y. Engelborghs, O. Michielin, W. Wahli and B. Desvergne, The endocrine disruptor monoethyl-hexyl-phthalate is a selective peroxisome proliferator-activated receptor gamma modulator that promotes adipogenesis, J. Biol. Chem., 2007, 282(26), 19152–19166 CrossRef CAS PubMed.
- M. Lehrke and M. A. Lazar, The many faces of PPARgamma, Cell, 2005, 123(6), 993–999 CrossRef CAS PubMed.
- J. N. Feige, L. Gelman, L. Michalik, B. Desvergne and W. Wahli, From molecular action to physiological outputs: peroxisome proliferator-activated receptors are nuclear receptors at the crossroads of key cellular functions, Prog. Lipid Res., 2006, 45(2), 120–159 CrossRef CAS PubMed.
- I. Kratochvil, T. Hofmann, S. Rother, R. Schlichting, R. Moretti, D. Scharnweber, V. Hintze, B. I. Escher, J. Meiler, S. Kalkhof and M. von Bergen, Mono(2-ethylhexyl) phthalate (MEHP) and mono(2-ethyl-5-oxohexyl) phthalate (MEOHP) but not di(2-ethylhexyl) phthalate (DEHP) bind productively to the peroxisome proliferator-activated receptor γ, Rapid Commun. Mass Spectrom., 2019, 33(suppl. 1), 75–85 CrossRef CAS PubMed.
- A. Useini, F. Engelberger, G. Künze and N. Sträter, Structural basis of the activation of PPARγ by the plasticizer metabolites MEHP and MINCH, Environ. Int., 2023, 173, 107822 CrossRef CAS PubMed.
- A. Lampen, S. Zimnik and H. Nau, Teratogenic phthalate esters and metabolites activate the nuclear receptors PPARs and induce differentiation of F9 cells, Toxicol. Appl. Pharmacol., 2003, 188(1), 14–23 CrossRef CAS PubMed.
- C. H. Hurst and D. J. Waxman, Activation of PPARalpha and PPARgamma by environmental phthalate monoesters, Toxicol. Sci., 2003, 74(2), 297–308 CrossRef CAS.
- R. Biemann, A. Navarrete Santos, A. Navarrete Santos, D. Riemann, J. Knelangen, M. Blüher, H. Koch and B. Fischer, Endocrine disrupting chemicals affect the adipogenic differentiation of mesenchymal stem cells in distinct ontogenetic windows, Biochem. Biophys. Res. Commun., 2012, 417(2), 747–752 CrossRef CAS.
- C. Hao, X. Cheng, J. Guo, H. Xia and X. Ma, Perinatal exposure to diethyl-hexyl-phthalate induces obesity in mice, Front. Biosci., 2013, 5(2), 725–733 CrossRef PubMed.
- H. C. Chiang, Y. T. Kuo, C. C. Shen, Y. H. Lin, S. L. Wang and T. C. Tsou, Mono(2-ethylhexyl) phthalate accumulation disturbs energy metabolism of fat cells, Arch. Toxicol., 2016, 90(3), 589–601 CrossRef CAS PubMed.
- M. T. Bility, J. T. Thompson, R. H. McKee, R. M. David, J. H. Butala, J. P. Vanden Heuvel and J. M. Peters, Activation of mouse and human peroxisome proliferator-activated receptors (PPARs) by phthalate monoesters, Toxicol. Sci., 2004, 82(1), 170–182 CrossRef CAS PubMed.
- S. C. Hasmall, N. H. James, N. Macdonald, A. R. Soames and R. A. Roberts, Species differences in response to diethylhexylphthalate: suppression of apoptosis, induction of DNA synthesis and peroxisome proliferator activated receptor alpha-mediated gene expression, Arch. Toxicol., 2000, 74(2), 85–91 CrossRef CAS PubMed.
- C. Hao, X. Cheng, H. Xia and X. Ma, The endocrine disruptor mono-(2-ethylhexyl) phthalate promotes adipocyte differentiation and induces obesity in mice, Biosci. Rep., 2012, 32(6), 619–629 CrossRef CAS.
- S. Ellero-Simatos, S. P. Claus, C. Benelli, C. Forest, F. Letourneur, N. Cagnard, P. H. Beaune and I. de Waziers, Combined transcriptomic-(1)H NMR metabonomic study reveals that monoethylhexyl phthalate stimulates adipogenesis and glyceroneogenesis in human adipocytes, J. Proteome Res., 2011, 10(12), 5493–5502 CrossRef CAS PubMed.
- J. N. Feige, A. Gerber, C. Casals-Casas, Q. Yang, C. Winkler, E. Bedu, M. Bueno, L. Gelman, J. Auwerx, F. J. Gonzalez and B. Desvergne, The pollutant diethylhexyl phthalate regulates hepatic energy metabolism via species-specific PPARalpha-dependent mechanisms, Environ. Health Perspect., 2010, 118(2), 234–241 CrossRef CAS PubMed.
- K. Schaedlich, S. Gebauer, L. Hunger, L. S. Beier, H. M. Koch, M. Wabitsch, B. Fischer and J. Ernst, DEHP deregulates adipokine levels and impairs fatty acid storage in human SGBS-adipocytes, Sci. Rep., 2018, 8(1), 3447 CrossRef PubMed.
- E. Campioli, A. Batarseh, J. Li and V. Papadopoulos, The endocrine disruptor mono-(2-ethylhexyl) phthalate affects the differentiation of human liposarcoma cells (SW 872), PLoS One, 2011, 6(12), e28750 CrossRef CAS PubMed.
- Z. Lv, J. Cheng, S. Huang, Y. Zhang, S. Wu, Y. Qiu, Y. Geng, Q. Zhang, G. Huang, Q. Ma, X. Xie, S. Zhou, T. Wu and Y. Ke, DEHP induces obesity and hypothyroidism through both central and peripheral pathways in C3H/He mice, Obesity, 2016, 24(2), 368–378 CrossRef CAS PubMed.
- J. S. Schmidt, K. Schaedlich, N. Fiandanese, P. Pocar and B. Fischer, Effects of di(2-ethylhexyl) phthalate (DEHP) on female fertility and adipogenesis in C3H/N mice, Environ. Health Perspect., 2012, 120(8), 1123–1129 CrossRef CAS PubMed.
- Y. Jia, T. Liu, L. Zhou, J. Zhu, J. Wu, D. Sun, J. Xu, Q. Wang, H. Chen, F. Xu, Y. Zhang, T. Zhang, H. Liu and L. Ye, Effects of Di-(2-ethylhexyl) Phthalate on Lipid Metabolism by the JAK/STAT Pathway in Rats, Int. J. Environ. Res. Public Health, 2016, 13(11), 1085 CrossRef PubMed.
- Y. Hayashi, Y. Ito, N. Yamagishi, Y. Yanagiba, H. Tamada, D. Wang, D. H. Ramdhan, H. Naito, Y. Harada, M. Kamijima, F. J. Gonzales and T. Nakajima, Hepatic peroxisome proliferator-activated receptor α may have an important role in the toxic effects of di(2-ethylhexyl) phthalate on offspring of mice, Toxicology, 2011, 289(1), 1–10 CrossRef CAS PubMed.
- N. Klöting, N. Hesselbarth, M. Gericke, A. Kunath, R. Biemann, R. Chakaroun, J. Kosacka, P. Kovacs, M. Kern, M. Stumvoll, B. Fischer, U. Rolle-Kampczyk, R. Feltens, W. Otto, D. K. Wissenbach, M. von Bergen and M. Blüher, Di-(2-Ethylhexyl)-Phthalate (DEHP) Causes Impaired Adipocyte Function and Alters Serum Metabolites, PLoS One, 2015, 10(12), e0143190 CrossRef PubMed.
- J. Xu, L. Zhou, S. Wang, J. Zhu, T. Liu, Y. Jia, D. Sun, H. Chen, Q. Wang, F. Xu, Y. Zhang, H. Liu, T. Zhang and L. Ye, Di-(2-ethylhexyl)-phthalate induces glucose metabolic disorder in adolescent rats, Environ. Sci. Pollut. Res. Int., 2018, 25(4), 3596–3607 CrossRef CAS PubMed.
- Y. Zhang, H. Feng, A. Tian, C. Zhang, F. Song, T. Zeng and X. Zhao, Long-term exposure to low-dose Di(2-ethylhexyl)
phthalate aggravated high fat diet-induced obesity in female mice, Ecotoxicol. Environ. Saf., 2023, 253, 114679 CrossRef CAS PubMed.
- G. Li, C. Y. Zhao, Q. Wu, S. Y. Guan, H. W. Jin, X. L. Na and Y. B. Zhang, Integrated metabolomics and transcriptomics reveal di(2-ethylhexyl) phthalate-induced mitochondrial dysfunction and glucose metabolism disorder through oxidative stress in rat liver, Ecotoxicol. Environ. Saf., 2021, 228, 112988 CrossRef CAS PubMed.
- P. Rajesh and K. Balasubramanian, Phthalate exposure in utero causes epigenetic changes and impairs insulin signalling, J. Endocrinol., 2014, 223(1), 47–66 CAS.
- G. Rajagopal, R. S. Bhaskaran and B. Karundevi, Maternal di-(2-ethylhexyl) phthalate exposure alters hepatic insulin signal transduction and glucoregulatory events in rat F1 male offspring, J. Appl. Toxicol., 2019, 39(5), 751–763 CrossRef CAS PubMed.
- A. C. Venturelli, K. B. Meyer, S. V. Fischer, D. H. Kita, R. A. Philipsen, R. N. Morais and A. J. M. Andrade, Effects of in utero and lactational exposure to phthalates on reproductive development and glycemic homeostasis in rats, Toxicology, 2019, 421, 30–40 CrossRef CAS PubMed.
- A. C. Venturelli, S. V. Fischer, R. N. de Morais, S. Grassiolli and A. J. M. Andrade, Effects of exposure to Di-(2-ethylhexyl) phthalate (DEHP) during lactation and puberty on sexual maturation and glycemic homeostasis in male rats, Clin. Nutr. ESPEN, 2015, 10(1), e5–e12 CrossRef PubMed.
- K. Aston-Mourney, J. Proietto, G. Morahan and S. Andrikopoulos, Too much of a good thing: why it is bad to stimulate the beta cell to secrete insulin, Diabetologia, 2008, 51(4), 540–545 CrossRef CAS PubMed.
- D. Gupta, T. Kono and C. Evans-Molina, The role of peroxisome proliferator-activated receptor γ in pancreatic β cell function and survival: therapeutic implications for the treatment of type 2 diabetes mellitus, Diabetes Obes. Metabol., 2010, 12(12), 1036–1047 CrossRef CAS PubMed.
- J. Zhao, J. Goldberg, J. D. Bremner and V. Vaccarino, Global DNA methylation is associated with insulin resistance: a monozygotic twin study, Diabetes, 2012, 61(2), 542–546 CrossRef CAS.
- L. Li, L. Huang, R. Lei, P. Zhang, Y. Yang, H. Liu and Y. Zhang, DEHP and DBP, common phthalates, induce glucose metabolism disorders in rats via oxidative damage of PI3K/Akt/GLUT4 signaling, Environ. Pollut., 2024, 341, 122948 CrossRef CAS PubMed.
- T. L. Hectors, C. Vanparys, A. Pereira-Fernandes, G. A. Martens and R. Blust, Evaluation of the INS-1 832/13 cell line as a beta-cell based screening system to assess pollutant effects on beta-cell function, PLoS One, 2013, 8(3), e60030 CrossRef CAS PubMed.
- L. Li, F. Wang, J. Zhang, K. Wang, X. De, L. Li and Y. Zhang, Typical phthalic acid esters induce apoptosis by regulating the PI3K/Akt/Bcl-2 signaling pathway in rat insulinoma cells, Ecotoxicol. Environ. Saf., 2021, 208, 111461 CrossRef CAS PubMed.
- V. Ramachandran, M. A. V. Anand, E. David, K. Venkatachalam, S. Vijayakumar, V. Sankaran, A. Balupillai, C. C. Sangeetha, K. M. Gothandam, V. S. Kotakadi, A. Ghidan, T. Al Antary and B. Xu, Antidiabetic Activity of Gold Nanoparticles Synthesized Using Wedelolactone in RIN-5F Cell Line, Antioxidants, 2019, 9(1), 8 CrossRef PubMed.
- Y. She, L. Jiang, L. Zheng, H. Zuo, M. Chen, X. Sun, Q. Li, C. Geng, G. Yang, L. Jiang and X. Liu, The role of oxidative stress in DNA damage in pancreatic β cells induced by di-(2-ethylhexyl) phthalate, Chem. Biol. Interact., 2017, 265, 8–15 CrossRef CAS PubMed.
- F. D. Yöntem, S. Ayaz, Ş. Bulut, E. H. Aldoğan and M. A. Ahbab, Endoplasmic reticulum stress and pro-inflammatory responses induced by phthalate metabolites monoethylhexyl phthalate and monobutyl phthalate in 1.1B4 pancreatic beta cells, Toxicology, 2024, 501, 153695 CrossRef PubMed.
- N. M. Weldingh, L. Jørgensen-Kaur, R. Becher, J. A. Holme, J. Bodin, U. C. Nygaard and A. K. Bølling, Bisphenol A is More Potent than Phthalate Metabolites in Reducing Pancreatic β-Cell Function, BioMed Res. Int., 2017, 2017, 4614379 Search PubMed.
- L. Jiang, T. Qiu, X. Yao, H. Chen, K. Yao, X. Sun, G. Yang, L. Jiang, C. Zhang, N. Wang, H. Zhang and X. Liu, MEHP induces pyroptosis and autophagy alternation by cathepsin B activation in INS-1 cells, Environ. Sci. Pollut. Res. Int., 2021, 28(47), 66628–66642 CrossRef CAS PubMed.
- R. Al-Abdulla, H. Ferrero, S. Soriano, T. Boronat-Belda and P. Alonso-Magdalena, Screening of Relevant Metabolism-Disrupting Chemicals on Pancreatic β-Cells: Evaluation of Murine and Human In Vitro Models, Int. J. Mol. Sci., 2022, 23(8), 4182 CrossRef CAS PubMed.
- G. Rajagopal, R. S. Bhaskaran and B. Karundevi, Developmental exposure to DEHP alters hepatic glucose uptake and transcriptional regulation of GLUT2 in rat male offspring, Toxicology, 2019, 413, 56–64 CrossRef CAS PubMed.
- S. Rengarajan, C. Parthasarathy, M. Anitha and K. Balasubramanian, Diethylhexyl phthalate impairs insulin binding and glucose oxidation in Chang liver cells, Toxicol. In Vitro, 2007, 21(1), 99–102 CrossRef CAS PubMed.
- P. Rajesh and K. Balasubramanian, Di(2-ethylhexyl)phthalate exposure impairs insulin receptor and glucose transporter 4 gene expression in L6 myotubes, Hum. Exp. Toxicol., 2014, 33(7), 685–700 CrossRef CAS PubMed.
- Y. Ding, Y. Liu, F. Fei, L. Yang, G. Mao, T. Zhao, Z. Zhang, M. Yan, W. Feng and X. Wu, Study on the metabolism toxicity, susceptibility and mechanism of di-(2-ethylhexyl) phthalate on rat liver BRL cells with insulin resistance in vitro, Toxicology, 2019, 422, 102–120 CrossRef CAS PubMed.
- Y. Ding, K. Gao, Y. Liu, G. Mao, K. Chen, X. Qiu, T. Zhao, L. Yang, W. Feng and X. Wu, Transcriptome analysis revealed the mechanism of the metabolic toxicity and susceptibility of di-(2-ethylhexyl)phthalate on adolescent male ICR mice with type 2 diabetes mellitus, Arch. Toxicol., 2019, 93(11), 3183–3206 CrossRef CAS PubMed.
- Y. Ding, T. Xu, G. Mao, Y. Chen, X. Qiu, L. Yang, T. Zhao, X. Xu, W. Feng and X. Wu, Di-(2-ethylhexyl) phthalate-induced hepatotoxicity exacerbated type 2 diabetes mellitus (T2DM) in female pubertal T2DM mice, Food Chem. Toxicol., 2021, 149, 112003 CrossRef CAS PubMed.
- J. Wang, J. Li, K. R. Zahid, K. Wang, Y. Qian, P. Ma, S. Ding, X. Yang and X. Wang, Adverse effect of DEHP exposure on the serum insulin level of Balb/c mice, Mol. Cell. Toxicol., 2016, 12, 83–91 CrossRef CAS.
- M. Armoni, N. Kritz, C. Harel, F. Bar-Yoseph, H. Chen, M. J. Quon and E. Karnieli, Peroxisome proliferator-activated receptor-gamma represses GLUT4 promoter activity in primary adipocytes, and rosiglitazone alleviates this effect, J. Biol. Chem., 2003, 278(33), 30614–30623 CrossRef CAS PubMed.
- Y. Xu, T. J. Cook and G. T. Knipp, Effects of di-(2-ethylhexyl)- phthalate (DEHP) and its metabolites on fatty acid homeostasis regulating proteins in rat placental HRP-1 trophoblast cells, Toxicol. Sci., 2005, 84(2), 287–300 CrossRef CAS PubMed.
- K. N. Keane, V. F. Cruzat, R. Carlessi, P. I. de Bittencourt Jr and P. Newsholme, Molecular Events Linking Oxidative Stress and Inflammation to Insulin Resistance and β-Cell Dysfunction, Oxid. Med. Cell. Longev., 2015, 2015, 181643 Search PubMed.
- T. M. D. Nguyen, Adiponectin: Role in Physiology and Pathophysiology, Int. J. Prev. Med., 2020, 11, 136 CrossRef PubMed.
- E. E. Kershaw and J. S. Flier, Adipose tissue as an endocrine organ, J. Clin. Endocrinol. Metab., 2004, 89(6), 2548–2556 CrossRef CAS PubMed.
- M. E. Trujillo and P. E. Scherer, Adiponectin–journey from an adipocyte secretory protein to biomarker of the metabolic syndrome, J. Intern. Med., 2005, 257(2), 167–175 CrossRef CAS.
- J. Ernst, U. Grabiec, K. Falk, F. Dehghani and K. Schaedlich, The endocrine disruptor DEHP and the ECS: analysis of a possible crosstalk, Endocr. Connect., 2020, 9(2), 101–110 CAS.
- R. B. Ceddia, R. Somwar, A. Maida, X. Fang, G. Bikopoulos and G. Sweeney, Globular adiponectin increases GLUT4 translocation and glucose uptake but reduces glycogen synthesis in rat skeletal muscle cells, Diabetologia, 2005, 48(1), 132–139 CrossRef CAS PubMed.
|
This journal is © The Royal Society of Chemistry 2024 |
Click here to see how this site uses Cookies. View our privacy policy here.