DOI:
10.1039/D4YA00252K
(Paper)
Energy Adv., 2024,
3, 1672-1677
Construction of a supramolecular light-harvesting system based on pillar[5]arene-mediated nanoparticles in water†
Received
19th April 2024
, Accepted 20th May 2024
First published on 22nd May 2024
Abstract
Light harvesting and energy transfer are ubiquitous processes in natural photosynthesis, significantly advancing the widespread utilization of solar energy. In this study, we engineered a supramolecular light-harvesting system utilizing a pyridinium salt-modified cyanostilbene guest (CPy) and a water-soluble pillar[5]arene host (WP5). Through host–guest complexation between WP5 and CPy, the resultant supra-amphiphile further self-assembled into emissive nanoparticles within aqueous environments. Incorporating the commercially available dye DBT into these nanoparticles yielded an efficient artificial light-harvesting system with a high donor/acceptor ratio (>200). Additionally, this system demonstrated tunable fluorescence emission in the solid state and exhibited potential applications as a color-tunable fluorescent ink for information encryption. Our findings not only delineate a promising approach for fabricating efficient light-harvesting systems via a straightforward supramolecular strategy but also underscore the significant potential of tunable photoluminescent nanomaterials.
Introduction
Inspired by natural light-harvesting systems,1–3 scientists are driven to harness renewable energy resources more effectively. These systems, like chloroplasts found in green plants, excel in capturing, transferring, and storing solar energy within nano-sized devices. Moreover, the light-harvesting systems found in chloroplasts are intricate supramolecular organizations of chlorophyll and protein molecules.4 Taking cues from nature, numerous artificial light-harvesting systems (ALHS) assembled by non-covalent interactions have emerged in recent years for different applications,5–10 such as photocatalysis,11–18 tunable luminescent materials,19–30 chemical sensing,31–33 bioimaging/therapy,34–36 temperature sensing,37–41 information encryption42–44 and latent fingerprint imaging.45,46 There are three key conditions to achieve efficient Förster resonance energy transfer (FRET) from an energy donor (D) to an acceptor (A): firstly, a substantial overlap between the absorption spectrum of the acceptor and the emission spectrum of the donor is a prerequisite; secondly, the donor must be densely packed while circumventing aggregation-induced fluorescence quenching (ACQ); thirdly, maintaining a high donor-to-acceptor ratio (D/A > 200) ensures that multiple donors are associated with a single acceptor, optimizing energy transfer efficiency.47–50
To mitigate the ACQ effect associated with conventional dyes, the incorporation of aggregation-induced emission (AIE) donors becomes essential.51–53 In this context, the synergistic integration of AIE with supramolecular self-assembly offers significant advantages for the fabrication of ALHSs.54–57 Recently, fluorophores based on cyanostilbene (CS) have garnered considerable attention owing to their distinctive fluorescence properties.58–62 CS derivatives often exhibit weak emission in organic solvents but display remarkable fluorescence in aqueous environments. Consequently, the CS group can serve as an effective AIE unit for the synthesis of various luminescent materials.63,64 Previously, our research group reported a multicolor fluorescent nanomaterial based on CS groups, demonstrating promising applications in time-dependent information encryption and dual-color latent fingerprint imaging.65 Furthermore, we integrated CS groups into quadruple hydrogen bonding polymers to engineer an ALHS with an ultralow acceptor content, thereby achieving white-light emission.66 Notably, supramolecular macrocycles such as pillar[5]arene can be readily converted into water-soluble hosts and possess remarkable capabilities for modulating the emission of guest molecules.67–70 Thus, the utilization of water-soluble pillar[5]arene to fine-tune CS-based simple guests represents a highly desirable strategy for achieving efficient FRET.
In this work, we devised and synthesized a straightforward guest molecule, denoted as CPy, incorporating a methyl pyridinium salt group as the host-binding motif and a CS group as the fluorescence-generating component (Fig. 1). Moreover, the synthesized water-soluble pillar[5]arene, designated as WP5, has the capability to form host–guest complexes with CPy, yielding a supra-amphiphile denoted as WP5⊃CPy. Consequently, WP5⊃CPy assemblies give rise to nanoparticles (NPs) in aqueous environments. This assembly not only amplifies the fluorescence intensity of CPy but also provides a nanostructured platform conducive to the development of ALHSs. To harness excitation energy from WP5⊃CPy, a commercially available fluorescent dye, 4,7-di(2-thienyl)-benzo[2,1,3]thiadiazole (DBT), was selected as the acceptor and loaded into the hydrophobic layer of the NPs. This system facilitates an efficient FRET process and exhibits a pronounced antenna effect in aqueous media. Remarkably, this ALHS not only emulates natural light-harvesting systems but also demonstrates significant potential as a fluorescent ink and solid-state luminescent material.
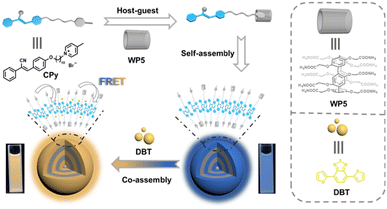 |
| Fig. 1 Schematic representation of the construction of ALHS in water based on WP5⊃CPy and DBT. | |
Experimental
All chemicals and reagents were purchased from commercial suppliers and utilized, unless specifically noted otherwise, without additional purification. Solvents, if required, were subjected to dehydration using established procedures outlined in the literature. Isolated yields were reported for all reactions. Proton nuclear magnetic resonance (1H NMR) spectra were acquired using a Bruker AVANCE III spectrometer operating at either 300 MHz or 400 MHz and calibrated relative to the residual proton signal or the natural abundance carbon resonance of the deuterated solvent, with tetramethylsilane (TMS) employed as the internal standard. Chemical shifts (δ) are expressed in parts per million (ppm) and coupling constants (J) are provided in Hz. Multiplicities are denoted as s (singlet), d (doublet), t (triplet), and m (multiplet). High-resolution electrospray ionization mass spectra (HR-ESI-MS) were recorded using an Agilent Technologies 6540 UHD Accurate-Mass spectrometer. Transmission electron microscopy (TEM) investigations were conducted on a JEM-2100 instrument. Dynamic light scattering (DLS) and zeta-potential (ζ-potential) measurements were performed using a Zetasizer Nano ZS ZEN3600 instrument. UV-vis absorption spectra were obtained using a PerkinElmer Lambda 35 UV-vis spectrometer, while fluorescence measurements were conducted on an agilent cary eclipse spectrofluorometer. Fluorescence lifetimes were determined using time-correlated single photon counting on an FLS980 instrument equipped with a pulsed xenon lamp. The fluorescence decay curves were fitted using a bi-exponential decay model. The instrument response function (IRF) measures the scattering of laser excitation from non-fluorescent control samples to ascertain the fastest possible response of the detectors. Quantum yield measurements were conducted using an FLS980 instrument with an integrating sphere. The CIE (Commission Internationale de l’Eclairage) 1931 coordinates were calculated with the method of color matching functions.
The macrocyclic host, WP5, is a water-soluble pillar[5]arene, synthesized following established procedures outlined in the literature.71 The synthesis of the guest molecule CPy is depicted in Scheme 1. Initially, 4-hydroxyphenylacetonitrile undergoes etherification with 1,10-dibromodecane to yield compound 1. Then, compound 1 undergoes condensation with benzaldehyde via a Knoevenagel reaction, resulting in the formation of compound 2. Finally, compound 2 reacts with 4-methylpyridine through nucleophilic substitution, culminating in the synthesis of the target product, CPy. Synthesis of compound 1 was carried out based on literature methods.72 Compound 2 and CPy were thoroughly characterized using 1H NMR, 13C NMR, and high-resolution electrospray ionization mass spectrometry (ESI-MS) techniques (Fig. S1–S6, ESI†).
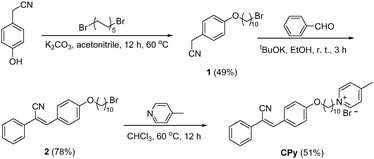 |
| Scheme 1 Synthetic route of CPy. | |
Synthesis of compound 2. Compounds 1 (0.50 g, 1.4 mmol) and tBuOK (0.16 g, 1.4 mmol) were introduced into a 250 mL three-neck flask under a nitrogen atmosphere. Subsequently, anhydrous ethanol (75 mL) was added. Once the solid was completely dissolved, benzaldehyde (0.18 g, 1.7 mmol) was gradually added. The resulting mixture was stirred at room temperature for 3 h. Upon completion of the reaction, the solid was retained following extraction and filtration, yielding white solid compound 2 (0.49 g, 1.1 mmol) with a yield of 78%. 1H NMR (CDCl3, 300 MHz, 298 K) δ (ppm): 7.86 (d, J = 7.2 Hz, 2H, Ar-H), 7.60 (d, J = 8.4 Hz, 2H, Ar-H), 7.35 (m, 4H, Ar-H and –CH
C(CN)–), 6.95 (d, J = 8.4 Hz, 2H, Ar-H), 4.00 (t, J = 6.3 Hz, 2H, –OCH2), 3.41 (t, J = 6.9 Hz, 2H, –BrCH2–), 1.91–1.80 (m, 4H, –CH2–), 1.78–1.33 (m, 12H, –CH2–). 13C NMR (CDCl3, 100 MHz, 298 K) δ (ppm): 160.06, 140.00, 134.03, 130.13, 129.07, 128.93, 127.32, 126.75, 118.21, 114.97, 111.38, 68.21, 34.10, 32.83, 29.73, 29.45, 29.38, 29.19, 28.76, 28.18, 26.01. HR-ESI-MS: m/z [M + Na]+ calcd for [C25H30BrNNaO]+ 462.1403, found 462.1403.
Synthesis of CPy. Compound 2 (0.50 g, 1.1 mmol) and 4-methylpyridine (1.48 g, 15.9 mmol) were combined in a 50 mL flask. Anhydrous chloroform (30 mL) was then added, and the mixture was stirred at 60 °C for 12 h. Upon completion of the reaction, the solvent was evaporated under reduced pressure. The resulting residue underwent column chromatography (eluent: PE
:
EA = 1
:
1, MeOH
:
H2O = 10
:
1, v/v), yielding a pure brown solid compound CPy (0.30 g, 0.56 mmol) with a yield of 51%. 1H NMR (300 MHz, DMSO-d6, 298 K) δ (ppm): 8.93 (d, J = 6.0 Hz, 2H, Ar-H), 7.98 (d, J = 6.0 Hz, 2H, Ar-H), 7.92–7.89 (m, 3H, Ar-H and –CH
C(CN)–), 7.69 (d, J = 8.7 Hz, 2H, Ar-H), 7.55–7.47 (m, 3H, Ar-H), 7.05 (d, J = 8.7 Hz, 2H, Ar-H), 4.51 (t, J = 7.2 Hz, 2H, –NCH2–), 4.02 (t, J = 6.3 Hz, 2H, –OCH2–), 2.60 (s, 3H, –CH3), 1.88 (m, 2H, –CH2–), 1.71 (m, 2H, –CH2–), 1.40–1.22 (m, 12H, –CH2–). 13C NMR δ (100 MHz, DMSO-d6, 298 K) δ (ppm): 159.50, 158.73, 143.69, 140.50, 133.96, 130.21, 128.91, 128.87, 128.32, 127.20, 125.93, 118.03, 115.02, 109.95, 67.66, 59.87, 30.55, 28.83, 28.69 (2C), 28.56, 28.33, 25.44, 25.35, 21.33. HR-ESI-MS: m/z [M–Br]+ calcd for [C31H37N2O]+ 453.2901, found 453.3575.
Results and discussion
The AIE behavior of CPy in mixed solvents was initially explored. In pure water, CPy dissolved completely and exhibited no fluorescence emission. Upon the addition of a poor solvent, glycerol, the fluorescence of CPy at 430 nm gradually increased (Fig. 2a and b). A sharp increase in fluorescence was observed when the glycerol fraction reached 98%. Despite the amphiphilic nature of CPy, attempts to determine its critical aggregation concentration (CAC) in water through optical transmittance experiments (Fig. S7, ESI†) were inconclusive, indicating the absence of a distinct CAC within this concentration range. The aggregation of CPy molecules in mixed solvents appeared to be disordered and challenging to control. Based on these observations, we further endeavored to investigate the aggregation and luminescence of CPy through supramolecular self-assembly to achieve well-ordered nanostructures.
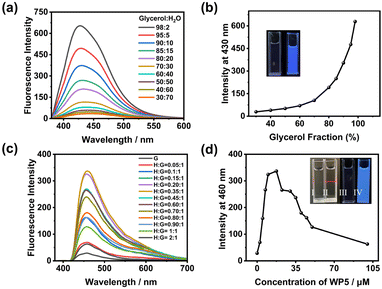 |
| Fig. 2 (a) Fluorescence spectra of CPy (1 × 10−4 M) in a glycerol/water solution with different fractions of glycerol (λex = 360 nm). (b) Fluorescence intensity of CPy at 430 nm in the presence of different factions of glycerol from 30 to 98%; inset: photographs of CPy in water (left) and in glycerol (right, with 2% water) under a turn-on UV lamp. (c) Fluorescence spectra of CPy (as the guest, G, 5 × 10−5 M) in pure water upon the titration of WP5 (as the host, H). (d) Fluorescence intensity of CPy (5 × 10−5 M) at 460 nm versus different concentrations of WP5; insets: Tyndall effects of CPy (I) and WP5⊃CPy (II), and fluorescence photographs of CPy (III) and WP5⊃CPy (IV). | |
WP5 was employed to facilitate the self-assembly of CPy through host–guest interactions in pure water. Upon the addition of WP5 to the CPy solution (5 × 10−5 M), a notable enhancement in the fluorescence intensity of CPy was observed (Fig. 2c), indicating the effective induction of CPy self-assembly by WP5 in water. The fluorescence intensity reached its maximum when the WP5 concentration was 17.5 μM, suggesting an optimal molar ratio of WP5 to CPy of 0.35
:
1 (Fig. 2d). Additionally, a distinct Tyndall effect was observed for WP5⊃CPy, confirming the presence of abundant NPs in the solution (Fig. 2d, inset). Notably, the CAC of WP5⊃CPy was determined to be 2.5 μM (Fig. S8, ESI†). In the presence of WP5, the absolute fluorescence quantum yield of CPy increased significantly to 2.72%, underscoring the pivotal role of WP5 in fluorescence enhancement (Fig. S10a, ESI†).
The morphology and size of the WP5⊃CPy nanostructures were characterized using transmission electron microscopy (TEM) and dynamic light scattering (DLS). DLS analysis revealed a narrow size distribution for WP5⊃CPy, with an average hydrodynamic diameter of 142 nm (Fig. 3a). TEM imaging depicted spherical nanoaggregates with a diameter of approximately 100 nm, consistent with the DLS data (Fig. 3c). As WP5⊃CPy NPs exhibited pronounced fluorescence in pure water, efforts were made to construct a donor–acceptor system by co-assembling hydrophobic ACQ dyes into the NPs. To this end, the commercially available dye DBT was chosen due to its well-matched photophysical spectra (Fig. 4a). The morphology of WP5⊃CPy-DBT NPs was also investigated using DLS and TEM, revealing a spherical structure with an average hydrodynamic diameter of 191 nm (Fig. 3b and d). Zeta potential measurements were further conducted for both WP5⊃CPy (−53 mV) and WP5⊃CPy-DBT (−36 mV) NPs (Fig. S9, ESI†). The relatively high negative values for both nanoparticles indicate their stability in water, as their surfaces are repulsive and resistant to agglomeration.
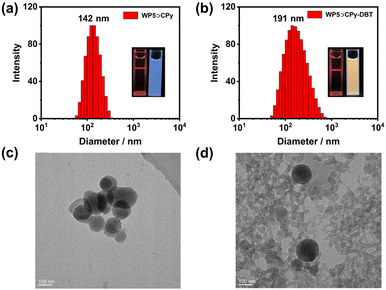 |
| Fig. 3 DLS data of (a) WP5⊃CPy NPs (inset: Tyndall effect and fluorescence photograph of WP5⊃CPy), and (b) WP5⊃CPy-DBT NPs (inset: Tyndall effect and fluorescence photograph of WP5⊃CPy-DBT). TEM images of (c) WP5⊃CPy NPs and (d) WP5⊃CPy-DBT NPs. [CPy] = 5 × 10−5 M, [WP5] = 1.75 × 10−5 M, [DBT] = 2.5 × 10−7 M, respectively. | |
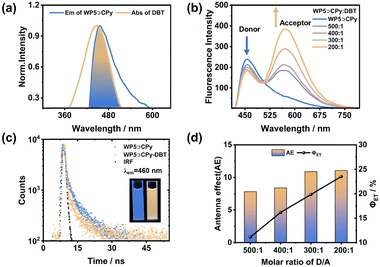 |
| Fig. 4 (a) Normalized absorption and emission spectra of WP5⊃CPy (blue curve) and DBT (orange curve), respectively. (b) Fluorescence spectra of WP5⊃CPy-DBT in water at different donor/acceptor molar ratios. (c) Fluorescence decay profiles of WP5⊃CPy and WP5⊃CPy-DBT; inset: fluorescence photographs of WP5⊃CPy (left) and WP5⊃CPy-DBT (right). (d) Energy-transfer efficiency (ΦET) and antenna effect (AE) of the ALHS with varying acceptor content. [CPy] = 5 × 10−5 M, [WP5] = 1.75 × 10−5 M, [DBT] = 2.5 × 10−7 M, respectively. | |
As mentioned above, DBT served as the energy acceptor to capture excitation energy from WP5⊃CPy on account of their favorable energy-matching characteristics. As shown in Fig. 4a, the absorption spectrum of DBT exhibited significant overlap with the emission spectrum of WP5⊃CPy, facilitating efficient energy transfer between WP5⊃CPy and DBT. Upon the co-assembly of increasing DBT content, the fluorescence intensity of WP5⊃CPy at 460 nm decreased markedly, while the fluorescence peak of DBT at 580 nm continued to rise (Fig. 4b), indicative of the occurrence of energy transfer. Furthermore, a noticeable shift in fluorescence color from blue to orange was observed (Fig. 4c, inset). The FRET process was further corroborated by time-resolved fluorescence analysis. Fluorescence lifetimes were fitted as a double exponential decay for both samples (Fig. 4c). The fluorescence lifetimes of WP5⊃CPy were determined to be τ1 = 0.84 ns and τ2 = 7.11 ns (Table S2, ESI†). In contrast, the fluorescence lifetimes of WP5⊃CPy-DBT decreased to τ1 = 0.46 ns and τ2 = 5.44 ns, providing additional evidence of light harvesting and energy transfer occurring within the nanoparticles.
To quantitatively assess the performance of the ALHS based on WP5⊃CPy-DBT, the energy transfer efficiency (ΦET) and antenna effect (AE) were systematically calculated. ΦET represents the ratio of the excitation energy absorbed by DBT to the total energy of WP5⊃CPy. Additionally, AE measures the emission amplification of DBT within the ALHS compared to its direct excitation. The resulting system exhibited enhanced energy transfer efficiency with increasing acceptor content (D/A = 500/1–200/1) (Fig. 4d). ΦET reaches 23.5% when D/A = 200/1 (Fig. S11 and Table S3, ESI†). Moreover, the system demonstrated considerable antenna effect values (7 to 11) at high D/A ratios (Fig. S12 and Table S4, ESI†). Furthermore, the absolute fluorescence quantum yield of the system increased from 2.72% (WP5⊃CPy) to 13.69% (WP5⊃CPy-DBT), indicative of a significant fluorescence enhancement through light harvesting (Fig. S10 and Table S1, ESI†). These quantitative findings collectively suggest that WP5⊃CPy-DBT represents an efficient supramolecular ALHS.
The dynamic characteristics of the WP5⊃CPy-DBT system confer upon it the ability to exhibit adjustable fluorescence colors. As depicted in the CIE 1931 chromaticity coordinates (Fig. 5a), the fluorescence emission color of the system varies with the molar ratio. Upon increasing the DBT content, the emission color of the system in water transitions gradually from blue to yellow. This transition is also evident in the fluorescence photograph of the samples (Fig. 5a, inset) and in the fluorescence spectra obtained through three-dimensional processing (Fig. 5b). Due to the system's AIE behavior, it displays luminescence not only in aqueous solution but also in the solid state. Upon inscription onto paper, characters rendered with the ALHS material remain unreadable under natural light but become clearly discernible under UV illumination, emitting a golden fluorescence color. This suggests the potential of the WP5⊃CPy-DBT system to serve as a customizable fluorescent encryption ink (Fig. 5c).
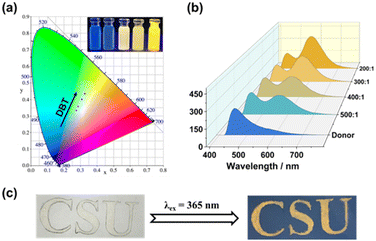 |
| Fig. 5 (a) CIE chromaticity coordinates of WP5⊃CPy at various concentrations of DBT, accompanied by inset photographs displaying WP5⊃CPy NPs at different DBT concentrations under UV irradiation. (b) Fluorescence spectra of WP5⊃CPy in aqueous solution with varying concentrations of DBT. (c) Illustration of characters inscribed using a solution of WP5⊃CPy-DBT (D/A = 200/1), demonstrating its encryption function. Concentrations used in the experiments: [CPy] = 5 × 10−5 M, [WP5] = 1.75 × 10−5 M, [DBT] = 2.5 × 10−7 M, respectively. | |
Conclusions
In summary, we have engineered an artificial light-harvesting system in aqueous solution through the host–guest interaction between a pillar[5]arene host and a CS-based guest. The guest molecule, CPy, comprises a CS moiety and a methylpyridinium unit linked by a ten-carbon alkyl spacer, rendering it AIE active. Consequently, the water-soluble pillar[5]arene WP5 induces the self-assembly of CPy into emissive nanoparticles, serving as an effective nano-platform and energy donor. Through the incorporation of the commercially available dye DBT into the supramolecular nanoparticles, we successfully constructed an efficient ALHS (WP5⊃CPy-DBT) based on FRET. The D/A ratio of the system is dynamically adjustable, leading to tunable fluorescence emission colors. Furthermore, these nanoparticles in aqueous solutions can serve as efficient solid-state information encryption materials due to their AIE properties. This study not only employs a straightforward supramolecular approach to fabricate an artificial light-harvesting system but also presents novel concepts for harnessing light energy and developing tunable luminescent materials.
Conflicts of interest
There are no conflicts to declare.
Acknowledgements
We gratefully acknowledge the National Natural Science Foundation of China (21702020), and The Analysis and Testing Center, NERC Biomass of Changzhou University is also acknowledged.
Notes and references
- R. J. Sension, Nature, 2007, 446, 740–741 CrossRef CAS PubMed.
- X. Qin, M. Suga, T. Kuang and J.-R. Shen, Science, 2015, 348, 989–995 CrossRef CAS PubMed.
- T. Mirkovic, E. E. Ostroumov, J. M. Anna, R. van Grondelle, Govindjee and G. D. Scholes, Chem. Rev., 2017, 117, 249–293 CrossRef CAS PubMed.
- J. Otsuki, J. Mater. Chem. A, 2018, 6, 6710–6753 RSC.
- Z. Wu, H. Qian, X. Li, T. Xiao and L. Wang, Chin. Chem. Lett., 2024, 35, 108829 CrossRef CAS.
- D. Bokotial, K. Acharyya, A. Chowdhury and P. S. Mukherjee, Angew. Chem., Int. Ed., 2024, 63, e202401136 CrossRef CAS PubMed.
- X. M. Chen, X. Chen, X. F. Hou, S. Zhang, D. Chen and Q. Li, Nanoscale Adv., 2023, 5, 1830–1852 RSC.
- K. Wang, K. Velmurugan, B. Li and X. Y. Hu, Chem. Commun., 2021, 57, 13641–13654 RSC.
- T. Xiao, W. Zhong, L. Zhou, L. Xu, X.-Q. Sun, R. B. P. Elmes, X.-Y. Hu and L. Wang, Chin. Chem. Lett., 2019, 30, 31–36 CrossRef CAS.
- X.-Y. Dai, M. Huo and Y. Liu, Nat. Rev. Chem., 2023, 7, 854–874 CrossRef CAS PubMed.
- Z. Zhang, Z. Zhao, Y. Hou, H. Wang, X. Li, G. He and M. Zhang, Angew. Chem., Int. Ed., 2019, 58, 8862–8866 CrossRef CAS PubMed.
- M. Hao, G. Sun, M. Zuo, Z. Xu, Y. Chen, X. Y. Hu and L. Wang, Angew. Chem., Int. Ed., 2020, 59, 10095–10100 CrossRef CAS PubMed.
- P. P. Jia, L. Xu, Y. X. Hu, W. J. Li, X. Q. Wang, Q. H. Ling, X. Shi, G. Q. Yin, X. Li, H. Sun, Y. Jiang and H. B. Yang, J. Am. Chem. Soc., 2021, 143, 399–408 CrossRef CAS PubMed.
- D. Zhang, W. Yu, S. Li, Y. Xia, X. Li, Y. Li and T. Yi, J. Am. Chem. Soc., 2021, 143, 1313–1317 CrossRef CAS PubMed.
- Y. Li, C. Xia, R. Tian, L. Zhao, J. Hou, J. Wang, Q. Luo, J. Xu, L. Wang, C. Hou, B. Yang, H. Sun and J. Liu, ACS Nano, 2022, 16, 8012–8021 CrossRef CAS PubMed.
- G. Sun, M. Li, L. Cai, D. Wang, Y. Cui, Y. Hu, T. Sun, J. Zhu and Y. Tang, J. Colloid Interface Sci., 2023, 641, 803–811 CrossRef CAS PubMed.
- Y. Wang, C. Q. Ma, X. L. Li, R. Z. Dong, H. Liu, R. Z. Wang, S. S. Yu and L. B. Xing, J. Mater. Chem. A, 2023, 11, 2627–2633 RSC.
- T. Xiao, D. Chen, H. Qian, Y. Shen, L. Zhang, Z.-Y. Li and X.-Q. Sun, Dyes Pigm., 2023, 210, 110958 CrossRef CAS.
- Z. Xu, S. Peng, Y. Y. Wang, J. K. Zhang, I. Lazar Alexandra and D. S. Guo, Adv. Mater., 2016, 28, 7666–7671 CrossRef CAS PubMed.
- L. Ji, Y. Sang, G. Ouyang, D. Yang, P. Duan, Y. Jiang and M. Liu, Angew. Chem., Int. Ed., 2019, 58, 844–848 CrossRef CAS PubMed.
- X. H. Wang, N. Song, W. Hou, C. Y. Wang, Y. Wang, J. Tang and Y. W. Yang, Adv. Mater., 2019, 31, e1903962 CrossRef PubMed.
- L. Xu, Z. Wang, R. Wang, L. Wang, X. He, H. Jiang, H. Tang, D. Cao and B. Z. Tang, Angew. Chem., Int. Ed., 2020, 59, 9908–9913 CrossRef CAS PubMed.
- Q. Song, S. Goia, J. Yang, S. C. L. Hall, M. Staniforth, V. G. Stavros and S. Perrier, J. Am. Chem. Soc., 2021, 143, 382–389 CrossRef CAS PubMed.
- Y. Han, X. Zhang, Z. Ge, Z. Gao, R. Liao and F. Wang, Nat. Commun., 2022, 13, 3546 CrossRef CAS PubMed.
- Z. Lian, J. He, L. Liu, Y. Fan, X. Chen and H. Jiang, Nat. Commun., 2023, 14, 2752 CrossRef CAS PubMed.
- B. Shi, P. Qin, W. Li, H. Feng, Y. Zhou, Y. Chai, W.-J. Qu, T.-B. Wei, Y.-M. Zhang and Q. Lin, Inorg. Chem., 2023, 62, 17236–17240 CrossRef CAS PubMed.
- M. D. Tian, Z. Wang, X. Yuan, H. Zhang, Z. X. Liu and Y. Liu, Adv. Funct. Mater., 2023, 33, 2300779 CrossRef CAS.
- T. Xiao, H. Qian, X. Li, Z. Wu, Z.-Y. Li and X.-Q. Sun, Dyes Pigm., 2023, 215, 111289 CrossRef CAS.
- D. Jia, H. Zhong, S. Jiang, R. Yao and F. Wang, Chin. Chem. Lett., 2022, 33, 4900–4903 CrossRef CAS.
- J.-L. Yu, Z. Chen, Y.-Q. Zhu, Y.-L. Jin, X. Wang, M.-X. Wu, X.-H. Wang and Y.-W. Yang, Aggregate, 2024, 5, e562 CrossRef.
- T. Xiao, C. Bao, L. Zhang, K. Diao, D. Ren, C. Wei, Z.-Y. Li and X.-Q. Sun, J. Mater. Chem. A, 2022, 10, 8528–8534 RSC.
- X. Ma, T. Ren, J. Tang, J. Zhang, J. Wei, Y. Liang, J. Zhang, E. Feng and X. Han, ChemistrySelect, 2024, 9, e202304171 CrossRef CAS.
- Z. Wu, Q. Zhang, D. Chen and T. Xiao, Sens. Diagn., 2024, 3, 295–300 RSC.
- X. M. Chen, Q. Cao, H. K. Bisoyi, M. Wang, H. Yang and Q. Li, Angew. Chem., Int. Ed., 2020, 59, 10493–10497 CrossRef CAS PubMed.
- K.-X. Teng, Z.-P. An, L.-Y. Niu and Q.-Z. Yang, ACS Mater. Lett., 2023, 6, 290–297 CrossRef.
- X. Q. Tian, S. K. Li, K. Velmurugan, Z. H. Bai, Q. Liu, K. Y. Wang, M. Z. Zuo and X. Y. Hu, Mater. Chem. Front., 2023, 7, 2484–2492 RSC.
- Z. Wang, X. He, T. Yong, Y. Miao, C. Zhang and B. Zhong Tang, J. Am. Chem. Soc., 2020, 142, 512–519 CrossRef CAS PubMed.
- R. Biswas and S. Banerjee, J. Phys. Chem. B, 2023, 127, 4135–4144 CrossRef CAS PubMed.
- T. Xiao, D. Ren, L. Tang, Z. Wu, Q. Wang, Z.-Y. Li and X.-Q. Sun, J. Mater. Chem. A, 2023, 11, 18419–18425 RSC.
- T. Xiao, L. Tang, D. Ren, K. Diao, Z.-Y. Li and X.-Q. Sun, Chem. – Eur. J., 2023, 29, e202203463 CrossRef CAS PubMed.
- L. Tang, Z. Wu, Q. Zhang, Q. Hu, X. Dang, F. Cui, L. Tang and T. Xiao, Chem. Commun., 2024, 60, 4719–4722 RSC.
- T. Xiao, X. Wei, H. Wu, K. Diao, Z.-Y. Li and X.-Q. Sun, Dyes Pigm., 2021, 188, 109161 CrossRef CAS.
- T. Xiao, H. Qian, Y. Shen, C. Wei, D. Ren, L. Zhang, Z. Y. Li, L. Wang and X. Q. Sun, Mater. Today Chem., 2022, 24, 100833 CrossRef CAS.
- B. Mu, X. Hao, X. Luo, Z. Yang, H. Lu and W. Tian, Nat. Commun., 2024, 15, 903 CrossRef CAS PubMed.
- K. Zhong, S. Lu, W. Guo, J. Su, S. Sun, J. Hai and B. Wang, Chem. Commun., 2021, 57, 9434–9437 RSC.
- T. Xiao, L. Zhang, D. Chen, Q. Zhang, Q. Wang, Z.-Y. Li and X.-Q. Sun, Org. Chem. Front., 2023, 10, 3245–3251 RSC.
- B. Sk and S. Hirata, Chem. Commun., 2023, 59, 6643–6659 RSC.
- L. Wu, C. Huang, B. P. Emery, A. C. Sedgwick, S. D. Bull, X.-P. He, H. Tian, J. Yoon, J. L. Sessler and T. D. James, Chem. Soc. Rev., 2020, 49, 5110–5139 RSC.
- R. M. Clegg, Cur. Opin. Biotech., 1995, 6, 103–110 CrossRef CAS PubMed.
- S. Guo, Y. Song, Y. He, X. Y. Hu and L. Wang, Angew. Chem., Int. Ed., 2018, 57, 3163–3167 CrossRef CAS PubMed.
- F. Würthner, Angew. Chem., Int. Ed., 2020, 59, 14192–14196 CrossRef PubMed.
- Y. Hong, J. W. Y. Lam and B. Z. Tang, Chem. Soc. Rev., 2011, 40, 5361–5388 RSC.
- X.-Y. Lou, G. Zhang, N. Song and Y.-W. Yang, Biomaterials, 2022, 286, 121595 CrossRef CAS PubMed.
- Y.-X. Hu, W.-J. Li, P.-P. Jia, X.-Q. Wang, L. Xu and H.-B. Yang, Adv. Opt. Mater., 2020, 8, 2000265 CrossRef CAS.
- X. Tang, Y. Zhu, W. Guan and C. Lu, Aggregate, 2023, 4, e348 CrossRef CAS.
- X. Y. Lou and Y. W. Yang, Aggregate, 2020, 1, 19–30 CrossRef.
- X. Y. Lou and Y. W. Yang, Adv. Opt. Mater., 2018, 6, 1800668 CrossRef.
- D. Ren, L. Tang, Z. Wu, Q. Zhang, T. Xiao, R. B. P. Elmes and L. Wang, Chin. Chem. Lett., 2023, 34, 108617 CrossRef CAS.
- Y. L. Ding, J. Guo, X. He, W. Tao, Y. D. Shi, J. H. Xu, L. Xu, M. X. Tang, D. K. Shen, H. Bi, Z. Q. Wu, K. Yang, Z. B. Zeng and P. F. Wei, Adv. Funct. Mater., 2023, 33, 2212886 CrossRef CAS.
- H. Wu and T. Xiao, Front. Chem., 2020, 8, 610093 CrossRef CAS PubMed.
- H.-J. Kim, P. C. Nandajan, J. Gierschner and S. Y. Park, Adv. Funct. Mater., 2018, 28, 1705141 CrossRef.
- Y. Zhang, Y. Xue, L. Gao, R. Liao, F. Wang and F. Wang, Chin. Chem. Lett., 2024, 35, 109217 CrossRef CAS.
- T. Xiao, X. Li, L. Zhang, K. Diao, Z.-Y. Li, X.-Q. Sun and L. Wang, Chin. Chem. Lett., 2024, 35, 108618 CrossRef CAS.
- X. H. Wang, X. Y. Lou, T. Lu, C. Wang, J. Tang, F. Liu, Y. Wang and Y. W. Yang, ACS Appl. Mater. Interfaces, 2021, 13, 4593–4604 CrossRef CAS PubMed.
- D. Chen, C. Bao, L. Zhang, Q. Zhang, Z. Wu, Z.-Y. Li, X.-Q. Sun, L. Wang and T. Xiao, Adv. Funct. Mater., 2024, 34, 2314093 CrossRef CAS.
- K. Diao, D. J. Whitaker, Z. Huang, H. Qian, D. Ren, L. Zhang, Z.-Y. Li, X.-Q. Sun, T. Xiao and L. Wang, Chem. Commun., 2022, 58, 2343–2346 RSC.
- T. Ogoshi, T.-a Yamagishi and Y. Nakamoto, Chem. Rev., 2016, 116, 7937–8002 CrossRef CAS PubMed.
- M. Xue, Y. Yang, X. Chi, Z. Zhang and F. Huang, Acc. Chem. Res., 2012, 45, 1294–1308 CrossRef CAS PubMed.
- T. Xiao, L. Zhou, L. Xu, W. Zhong, W. Zhao, X.-Q. Sun and R. B. P. Elmes, Chin. Chem. Lett., 2019, 30, 271–276 CrossRef CAS.
- H. Zheng, L. Fu, R. Wang, J. Jiao, Y. Song, C. Shi, Y. Chen, J. Jiang, C. Lin, J. Ma and L. Wang, Nat. Commun., 2023, 14, 590 CrossRef CAS PubMed.
- C. Ding, Y. Liu, T. Wang and J. Fu, J. Mater. Chem. B, 2016, 4, 2819–2827 RSC.
- G. Sun, W. Qian, J. Jiao, T. Han, Y. Shi, X.-Y. Hu and L. Wang, J. Mater. Chem. A, 2020, 8, 9590–9596 RSC.
|
This journal is © The Royal Society of Chemistry 2024 |
Click here to see how this site uses Cookies. View our privacy policy here.