DOI:
10.1039/D4AN01357C
(Paper)
Analyst, 2025,
150, 94-102
Prussian blue-doped CaCO3 nanoparticle-labeled secondary antibodies for electrochemical immunoassay of interleukin-6 with migraine patients
Received
19th October 2024
, Accepted 15th November 2024
First published on 15th November 2024
Abstract
The sensitive and accurate identification of interleukin-6 (IL-6) in biological fluids is essential for assessing migraine due to its role in different physiological and pathological processes. In this study, we designed a simple and feasible electrochemical immunosensing method for the voltammetric measurement of IL-6. The electrochemical immunosensor was fabricated through covalent conjugation of anti-IL-6 capture antibodies on the glassy carbon electrode with a typical carbodiimide coupling method. Anti-IL-6 secondary antibodies were labeled on the surface of Prussian blue-doped CaCO3 nanoparticles (PBCaNP) via the epoxy-amino reaction. The assay was carried out with a sandwich-type immunoreaction. In the presence of target IL-6, the analyte was sandwiched between the capture antibody and detection antibody. Thereafter, the carried PBCaNP accompanying the secondary antibody could be detected by using square wave voltammetry (SWV). The voltammetric peak current was dependent on the concentration of target IL-6. Under optimum conditions, the electrochemical immunosensor exhibited good analytical properties, and allowed detection of IL-6 within a wide linear range from 0.1 to 1000 pg mL−1. The limit of detection was estimated to be 0.078 pg mL−1 of IL-6 at the 3sB criterion. An intermediate reproducibility of ≤10.59% was accomplished with batch-to-batch identification, and good anti-interference capacity against other biomolecules was achieved. Importantly, clinical human serum samples obtained from 15 migraine patients were analyzed with the developed electrochemical immunosensors, giving results well-matched with those obtained from the referenced enzyme-linked immunosorbent assay (ELISA) method.
Introduction
Migraine is a common neurological disease experienced by approximately 6% of men and 18% of women in the general population.1,2 Several studies have suggested a role for the immune system in the pathophysiology of migraine.3,4 Interleukin-6 (IL-6) is a pleiotropic cytokine, and plays a significant role in the regulation of pain threshold and trigeminal nerve fiber sensitization in migraine.5,6 In patients with migraine, a significant change of IL-6 plasma concentrations was found after headache attacks. Meanwhile, IL-6 is also thought to facilitate pain signalling from the meninges during the development of headache.7,8 Thus, sensitive and specific detection of IL-6 concentrations in biological fluids is crucial for the effective treatment of migraine patients.
Recently, different methods and strategies have been designed for the detection of IL-6 based on various signal-generation principles.9–11 Immunoassay is a promising approach for selective and sensitive analysis, and has received increasing attention for diagnostics.12,13 Zhao et al. developed a dual fluorescence signal-enhancement immunosensor based on substrate modification for the simultaneous detection of interleukin-6 and procalcitonin.9 Vessella et al. in situ synthesized a V2CTx MXene-based immune tag for the electrochemical detection of interleukin-6 from breast cancer cells.10 The Dai group constructed a sensitive and portable pressure-based immunosensor to assess maternal plasma interleukin-6 levels.11 Despite many advances in this field, it is necessary to find new approaches that could improve the simplicity, selectivity, and sensitivity of clinical immunoassays.
Electrochemical immunosensors or immunoassays on the basis of specific antigen–antibody reactions have been widely applied for this purpose because of their high sensitivity, low power requirements, low cost, and high compatibility with advanced micromachining technologies.14–17 For the successful development of an innovative electrochemical immunosensor, one key concern is to explore high-efficiency signal-transduction labels (tags).18,19 Conventional methods for the design of electrochemical immunosensors utilize enzyme labels, e.g., horseradish peroxidase (HRP) or alkaline phosphatase (ALP).20,21 Typically, the electrochemical measurements are carried out through enzymatic conversion of certain substrates in the presence of electron mediators, e.g., [Fe(CN)6]4−/3−, methylene blue or thionine.22,23 In this case, the bioactivities of the labeled enzymes or antibodies might be affected by the electron mediators.24 Meanwhile, enzyme-based electrochemical immunoassays are generally susceptible to interference and changes under the assay conditions during the signal generation stage.25 To this end, our motivation in this study is to search for a new signal-transduction tag without the participation of natural enzymes.
Favourably, the rapidly emerging research field of nanotechnology opens a new horizon in the design of a new electrochemical immunosensor using nanomaterial labels, specifically electroactive nano labels.26,27 One major advantage of using nanomaterials is that one can control and tailor their properties in a very predictable manner to meet the requirements of specific applications.28 Prussian blue, with the formula FeIII4[FeII(CN)6]3, containing polar FeII–C–N–FeIII units, exhibits many interesting physicochemical functions, making it widely applied in catalysis, lithium-ion batteries, and sodium-ion batteries.29,30 Due to its low energy gap and different valances of iron in its unit cell, Prussian blue can produce electron movement for easy oxidation or reduction through an external potential.31–33 Ma et al. systematically summarized recent progress in Prussian blue-based nanoparticles in biosensor applications.34 To acquire a highly efficient electrochemical immunosensor, another important point is to amplify the detectable signal, because the level of target analyte is relatively low in the early stage of the disease. It might be difficult for Prussian blue alone to produce a strong electrochemical signal. To tackle this issue, it is advantageous for many Prussian blue molecules to be simultaneously encapsulated into one nanoparticle. Nanosized CaCO3 particles have been studied and have proved to be an ideal host candidate.35 During the synthesis, Prussian blue can be readily doped or entrapped into the nanosized CaCO3 particles, thereby resulting in signal amplification.
Based on the above-mentioned motivations, we designed a new electrochemical immunosensor for the voltammetric detection of IL-6 on an anti-IL-6 capture antibody-modified glassy carbon electrode by using Prussian blue-doped CaCO3 nanoparticles (PBCaNP) as the label (Scheme 1). The introduction of electroactive Prussian blue to the inner CaCO3 nanoparticle is expected to amplify the electrochemical signal of the immunosensor after the formation of a sandwiched immunocomplex. This system includes a capture antibody-functionalized electrode and detection antibody-labeled PBCaNP. The assay mainly involves the sandwiched immunoreaction and voltammetric measurement. The aim of this work is to explore a new, enzyme-free, in situ amplified electrochemical immunosensor for the ultrasensitive detection of low-abundance proteins.
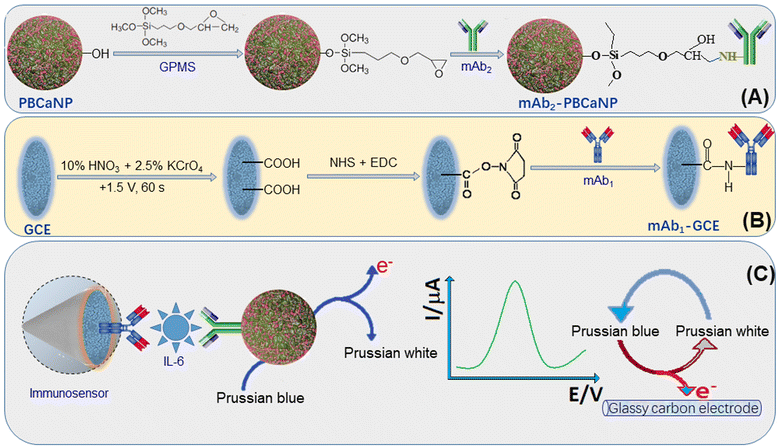 |
| Scheme 1 Preparation processes of (A) monoclonal rabbit anti-human IL-6 detection/secondary antibody (mAb2)-functionalized Prussian blue-doped CaCO3 nanoparticles (PBCaNP); and (B) monoclonal rabbit anti-human IL-6 capture antibody (mAb1)-conjugated glassy carbon electrode (GCE); (C) schematic illustration of the enzyme-free electrochemical immunosensor for the voltammetric measurement of interleukin-6 (IL-6) with a sandwich-type immunoreaction format. | |
Experimental
Materials and chemicals
Monoclonal rabbit anti-human interleukin-6 (IL-6) capture antibody (mAb1; clone number: EPR21711, cat#: ab233706) and monoclonal rabbit anti-human IL-6 secondary/detection antibody (mAb2; clone number: EPR20653, cat#: ab214429) were purchased from Abcam (Shanghai, China). Human IL-6 enzyme-linked immunosorbent assay (ELISA) kits were obtained from Abcam (Shanghai, China) (one-wash 90 min protocol, sensitivity: 1.6 pg mL−1, range: 7.8–500 pg mL−1, detection method: colorimetric, assay type: sandwich/quantitative, react with: human, cat#: ab178013). All the glassy carbon electrodes with a diameter of 4.0 mm were obtained from Chenhua Instrument Co., Ltd (Shanghai, China). N-Hydroxysuccinimide (NHS), N-(3-dimethylaminopropyl)-N′-ethylcarbodiimide hydrochloride (EDC), bovine serum albumin (BSA), γ-glycidoxypropyltrimethoxysilance (GPMS), and bis(2-ethylhexyl) sodium sulfosuccinate (AOT) were purchased from Sigma-Aldrich (Merck KGaA, Darmstadt, Germany). All other reagents were of analytical grade and were used without further purification, unless specified otherwise. Ultrapure water from a Milli-Q water purification system (18.2 MΩ cm−1, Millipore) was used throughout this work. All the buffers including phosphate-buffered saline (PBS) solution were products of Sigma.
Synthesis of Prussian blue-doped CaCO3 nanoparticles (PBCaNPs)
Prussian blue-doped CaCO3 nanoparticles (referred to as PBCaNPs) were synthesized through the reverse-micelle method, with consultation of the literature.36 Prior to synthesis, two solutions were prepared as follows: solution A containing CaCl2 (500 μL, 10 mM) in ultrapure water, K4Fe(CN)6 (500 μL, 10 mM) in ultrapure water and AOT in isooctane (15 mL, 0.1 M); and solution B containing Na2CO3 (500 μL, 10 mM) in ultrapure water, FeCl3 (500 μL, 10 mM) in ultrapure water and AOT in isooctane (15 mL, 0.1 M). After adequate stirring, solution B was added dropwise into solution A under vigorous stirring until the formation of blue PBCaNP colloids occurred. Then, absolute ethanol (3.0 mL) was injected into the mixture for stirring for another 10 min. During this process, the reverse micelle was completely broken down to form two immiscible layers of aqueous ethanol and isooctane. The ethanol was carefully removed through a separating funnel. Finally, the as-prepared PBCaNPs were washed 3–4 times with isooctane and centrifuged to remove residual components. The obtained PBCaNPs were collected for subsequent use.
Covalent labelling of PBCaNPs with mAb2 antibodies
Anti-IL-6 detection/secondary mAb2 antibodies were modified onto the surface of PBCaNPs through the epoxy-amino reaction, similar to previous work.37 Before modification, the above-prepared PBCaNPs were initially dried at 60 °C for 60 min. Then, 100 mg of PBCaNPs were added to 1.0 mL of 5% GPMS in dry toluene, and reacted for 12 h at room temperature under continuous shaking on a shaker. Then, the functionalized nanoparticles were thoroughly washed with toluene and ethanol through centrifugation to remove the physically adsorbed GPMS. In this case, the active epoxy groups on the surface of the PBCaNPs could be readily reacted with the amino groups of the antibody. Next, the resulting PBCaNPs were added to 1.0 mL of 0.1 mg mL−1 mAb2 antibody in PBS (pH 7.4, 10 mM), and reacted for 12 h at 4 °C under slight shaking. After washing with PBS (pH 7.4, 10 mM) and centrifugal separation, the residual active epoxy groups on the surface of the PBCaNPs were blocked with BSA (1.0 mL, 3.0 wt%). Finally, mAb2-functionalized PBCaNPs (designated as mAb2-PBCaNPs) were dispersed into 5.0 mL of pH 7.4 10 mM PBS, and stored at 4 °C for subsequent use (note: the concentration of mAb2-PBCaNPs was about 20 mg mL−1).
Fabrication of mAb1-modified electrochemical immunosensor
Initially, a glassy carbon electrode was polished repeatedly with 1.0 and 0.3 μm alumina slurry, followed by sonication in ultrapure water and ethanol for 5 min, and then dried in air. Thereafter, the cleaned electrode was electrochemically activated by holding it at +1.5 V for 60 s in the mixture containing 10% HNO3 and 2.5% K2CrO7. During this process, many –COOH and –OH groups were formed on the surface of the glassy carbon electrode. After washing with ultrapure water, the resulting electrode was immersed in an aqueous solution containing EDC and NHS, and incubated for 60 min at room temperature. The resultant electrode was washed as before, and reacted again with 0.1 mg mL−1 of mAb1 antibody in PBS (pH 7.4, 10 mM) for 60 min at 4 °C. The residual active sites on the surface of the electrode were blocked with BSA (1.0 mL, 3.0 wt%). Prepared in this way, mAb1 antibodies were covalently conjugated onto the surface of the glassy carbon electrode (GCE) via the typical carbodiimide coupling method. The as-prepared electrochemical immunosensor was referred to as mAb1-GCE (note: the immunosensor was stored at 4 °C when not in use).
Electrochemical measurement of the immunosensor for IL-6
Scheme 1 shows a schematic illustration of the prepared immunosensor for the voltammetric measurement of target IL-6 with a sandwiched immunoreaction between mAb1-GCE and mAb2-PBCaNP. Initially, the as-prepared immunosensor was inverted on a stand. Then, a mixture containing 4.0 μL of various concentrations of IL-6 standard and 4.0 μL of 20 mg mL−1 mAb2-PBCaNPs was dropped on the surface of the mAb1-GCE, and incubated for 35 min at room temperature. During this process, target IL-6 was sandwiched between mAb1-GCE and mAb2-PBCaNP. After washing with PBS (pH 7.4, 10 mM), the resulting immunosensor was electrochemically measured in PBS (pH 5.0, 10 mM) using square wave voltammetry (SWV) from 50 mV to 450 mV (vs. Ag/AgCl) (amplitude: 25 mV; frequency: 15 Hz; increase E: 4.0 mV). The obtained voltammetric peak current (μA) was collected as the signal of the immunosensor for different concentrations of target IL-6. The sigmoidal curve was constructed through mathematically fitting experimental points by using Rodbard's four-parameter function with the Origin 8.0 software. Graphs were plotted in the form of voltammetric current against the logarithm of the IL-6 concentration. All measurements were carried out in triplicate at room temperature (25 ± 1.0 °C). The results are expressed as mean value ± standard deviation (SD) of three determinations and statistical significance was defined at P ≤ 0.05.38,39
Results and discussion
Choice of method and characterization of materials
In this work, the electrochemical immunosensor is designed for the voltammetric detection of target IL-6 on an anti-IL-6 capture antibody-functionalized glassy carbon electrode by using Prussian blue-doped CaCO3 nanoparticles for the labelling of the secondary antibody. Anti-IL-6 mAb1 capture antibodies are covalently conjugated onto the surface of the glassy carbon electrode through typical carbodiimide coupling, whereas anti-IL-6 secondary antibodies are labeled on the surface of the PBCaNPs via a classical epoxy-amino reaction. The preparation of immunosensors is relatively simple without the need for any other matrices. Such a one-step modification is expected to enhance and improve the reproducibility of the electrochemical immunosensor. Meanwhile, the introduction of Prussian blue into the nano labels can be employed to produce a detectable electrochemical signal after the antigen–antibody reaction because of strong redox properties. The use of CaCO3 nanoparticles can provide lots of room for the loading of Prussian blue, thus causing the signal amplification. The immunoassay is carried out with a one-step reaction because these two monoclonal antibodies have different clone numbers. The presence of target IL-6 triggers the formation of a sandwiched immunocomplex on the electrode. By monitoring the voltammetric peak current of the Prussian blue, we can indirectly evaluate the concentration of target IL-6 in the sample.
To realize our design, the successful fabrication and preparation of the electrochemical immunosensor and PBCaNPs are crucial. Prior to measurement, we used scanning electron microscopy (SEM; Hitachi 4800, Japan) and emission transmission electron microscopy (TEM; FEI Talos F200S G2, Thermo Scientific, USA) to characterize the mAb1-coated GCE and PBCaNP, respectively. Fig. 1A shows a typical SEM image of a cleaned glassy carbon electrode, and a smooth surface was observed. After modification with anti-IL-6 capture antibodies, the electrode surface became rough, suggesting a coating of biomolecules (Fig. 1B). Fig. 1C shows a TEM image of the as-synthesized PBCaNPs with an average diameter of 20 nm. To demonstrate the presence of iron element in the nanoparticles, Prussian blue-doped CaCO3 nanoparticles were investigated by using inductively coupled plasma optical emission spectrometry, and the content of iron (Fe) element in the CaCO3 nanoparticles was about 2.17 wt%.
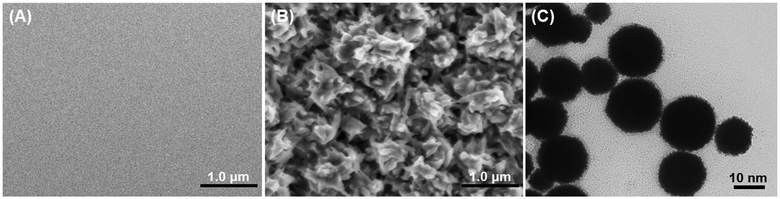 |
| Fig. 1 SEM images of (A) the cleaned glassy carbon electrode and (B) anti-IL-6 mAb1-functionalized GCE, and (C) TEM image of the as-synthesized Prussian blue-doped CaCO3 nanoparticles by using reverse-micelle method. | |
However, a puzzling question is whether anti-IL-6 mAb2 antibodies could be covalently conjugated onto the surface of PBCaNPs. To explore this issue, the preparation of mAb2-PBCaNPs after each step was monitored by calculating the zeta potentials on the basis of dynamic light scattering (DLS; Zetasizer Nano S90, Malvern, London, UK). A negative zeta potential (ζ = −13.2 mV) was achieved toward the newly prepared PBCaNPs, which was mainly derived from the –O− group of the nanoparticle surface. Upon reaction with the GPMS, the zeta potential was close to zero (ζ = −0.72 mV). This is most likely a consequence of the fact that the groups (–OH) on the surface of the PBCaNPs reacted with the silane [–Si(OH)3] of GPMS, and many epoxy groups were exposed on the surface, thus resulting in the decreasing zeta potential. The zeta potential changed to −29.8 mV when anti-IL-6 mAb2 antibodies were conjugated onto the GPMS-modified PBCaNPs, however. Since the conjugated anti-IL-6 mAb2 antibody is a kind of protein, the isoelectric point (Ip) is about 5.9, obtained by isoelectric focusing (IEF) electrophoresis. In pH 7.4 PBS (10 mM), the corresponding antibodies are negatively charged species. Therefore, the zeta potential of mAb2-PBCaNPs exhibited a negative value. On the basis of the above-mentioned results, we can preliminarily judge that anti-IL-6 mAb2 antibodies could be covalently conjugated onto the PBCaNPs.
Feasibility investigation of the electrochemical immunosensor
Using the above-prepared mAb1-GCE and mAb2-PBCaNPs, we first investigated the feasibility of the electrochemical immunosensor for the detection of target IL-6 (with 0.1 ng mL−1 IL-6 used as an example). Before voltammetric measurement, electrochemical impedance spectroscopy (EIS) was employed to verify the fabrication process of the immunosensor after each step in PBS (10 mM, pH 7.4) containing 10 mM Fe(CN)64−/3− and 0.1 M KCl within the range of 10−2 Hz to 105 Hz at an alternating voltage of 5.0 mV, because it could give information accompanying the change in the resistance during the assembly (Fig. 2A). Typically, the semicircle diameter of impedimetric spectroscopy equals the electron transfer resistance, Rre. This resistance controls the electron transfer kinetics of the redox-probe at the electrode interface, and the value varies when different substances are adsorbed onto the electrode surface. Curve ‘a’ represents the EIS of the cleaned GCE, and the resistance was about 503 Ω. When anti-IL-6 mAb1 antibodies were covalently conjugated onto the GCE, a big resistance was acquired (Rre ≈ 946 Ω) (curve ‘b’). This is mainly because of the coating mAb1 biomolecules. Meanwhile, Fe(CN)64−/3− ions were repelled by the negatively charged mAb1 antibody due to its isoelectric point (Ip) of ∼5.3. Furthermore, the corresponding resistances gradually increased when the immobilized mAb1 capture antibodies reacted with target IL-6 (curve ‘c’) and mAb2-PBCaNPs (curve ‘d’) in sequence. The formation of immunocomplexes with the weak conductivity hindered the electron transfer of Fe(CN)64−/3− ions from the solution to the electrode surface. These results indicated that the sandwiched immunoreaction could be executed between mAb1-GCE and mAb2-PBCaNPs in the presence of target IL-6.
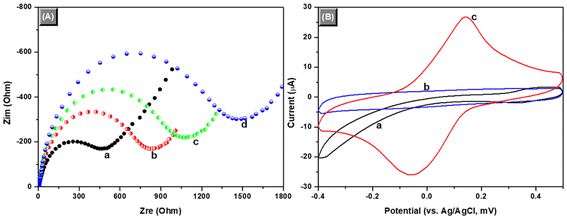 |
| Fig. 2 (A) Nyquist diagrams for (a) GCE, (b) mAb1-GCE, (c) mAb1-GCE after reaction with 0.1 ng mL−1 of IL-6, and (d) immunosensor ‘b’ after reaction with mAb2-PBCaNPs in PBS (10 mM, pH 7.4) containing 10 mM Fe(CN)64−/3− and 0.1 M KCl within the range of 10−2 Hz to 105 Hz at an alternating voltage of 5.0 mV. (B) Cyclic voltammograms for (a) mAb1-GCE, and (b) mAb1-GCE after reaction with 0.1 ng mL−1 of IL-6, and (c) immunosensor ‘b’ after reaction with mAb2-PBCaNPs in PBS (10 mM, pH 5.0) at 50 mV s−1. | |
As mentioned above, the major motivation of this work is to design a simple and sensitive voltammetric immunoassay for the detection of target analyte. To adequately display the advantages of the as-synthesized mAb2-PBCaNPs, we used cyclic voltammetry to study the voltammetric behaviours toward differently modified electrodes in pH 5.0 PBS (10 mM) at a scanning rate of 50 mV s−1 (Fig. 2B). No cyclic voltammetric peaks were observed for either mAb1-GCE (curve ‘a’) or IL-6/mAb1-GCE (curve ‘b’). However, the background current at the IL-6/mAb1-GCE was relatively small, suggesting that the introduction of target IL-6 decreased the electron communication between the solution and the electrode. Favourably, a pair of well-defined redox peaks at −50 mV and +150 mV was observed after the formation of sandwiched immunocomplexes with mAb2-PBCaNPs (curve ‘c’). The result also revealed that the as-synthesized mAb2-PBCaNPs had electrochemical activity, which could be used as an electron indicator for the determination of target analyte.
Optimization of immunosensing conditions
To achieve an optimum analytical result, some external experimental conditions and factors influencing the analytical properties of the immunosensors should be investigated, e.g., immunoreaction time and pH of PBS (note: we did not optimize the external conditions for the fabrication and preparation of mAb1-GCE and mAb2-PBCaNPs because of multiple conditions). In this work, the voltammetric signal was produced by the doped Prussian blue in the CaCO3 nanoparticles. Typically, the pH values of the supporting electrolyte (10 mM PBS used in this system) would greatly affect the peak current of the electron mediator. The peak potential and peak current varied at different pH values of PBS. Fig. 3A displays the effects of various pH values of PBS (10 mM) on the peak currents of the electrochemical immunosensors by using 0.1 ng mL−1 of IL-6 as an example. Clearly, low peak currents were observed at higher or lower pH values. An optimum current was achieved at pH 5.0 PBS. Thereafter, pH 5.0 of PBS (10 mM) was utilized for the supporting electrolyte for voltammetric measurements after the antigen–antibody reaction.
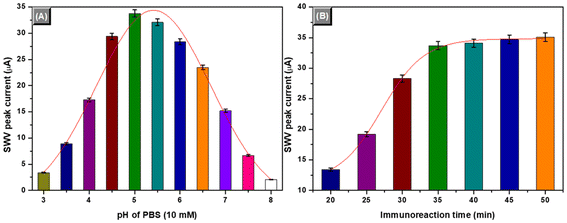 |
| Fig. 3 Effects of (A) pH of PBS (10 mM) as the supporting electrolyte and (B) immunoreaction time between mAb1-GCE and mAb2-PBCaNP for target IL-6 (0.1 ng mL−1 used as an example) on the SWV peak currents of the electrochemical immunosensor. | |
Another factor lies in the immunoreaction time. Generally, the reactions between antibodies and antigens are relatively slow. It takes some time to implement the reaction. Considering the future applications, all the immunoreactions were carried out at room temperature (25 ± 1.0 °C). Under these conditions, we monitored the effect of the immunoreaction time on the voltammetric peak currents of the immunosensors. As shown in Fig. 3B, the peak current increased with increasing immunoreaction time, and tended to level off after 35 min. A longer immunoreaction time did not cause a significant increase in the peak current. To save the assay time, 35 min of immunoreaction time was used for the antigen–antibody reaction.
Calibration plots of the electrochemical immunosensor for IL-6
Under the aforementioned conditions, the as-prepared mAb1-GCE and mAb2-PBCaNPs were employed for quantitative determination of IL-6 standards with various concentrations using a sandwiched assay format. The square wave voltammograms were recorded in PBS (pH 5.0, 10 mM) with different IL-6 levels. Fig. 4A displays the SWV responses of the electrochemical immunosensors toward various concentrations of target IL-6 standards. The SWV peak currents were not obvious at low IL-6 concentrations (from 0.01 to 0.1 pg mL−1) or high levels (from 1.0 to 5.0 ng mL−1), and exhibited a sigmoidal “S” relationship from 0.01 pg mL−1 to 5.0 ng mL−1. A good linear relationship between the SWV peak current and the decimal logarithm of IL-6 concentration (ng mL−1) was achieved within the range of 0.1 to 1000 pg mL−1 (Fig. 4B). The limit of detection (LOD) was estimated to 0.078 pg mL−1 at a signal-to-noise of 3sB (where sB is the standard deviation of zero-dose, n = 13). The regression equation was y (μA) = 11.61 + 11.15 × logC[IL-6] (pg mL−1, R2 = 0.994, n = 6). To highlight the merits of the developed electrochemical immunosensor, the analytical properties including the linear range and LOD were compared with other IL-6 analytical methods (Table 1). A comparable analytical performance could be given with our developed electrochemical immunosensors. Meanwhile, the threshold value of IL-6 in human normal serum was 0.373–0.463 pg mL−1. Thus, our system is highly advantageous for the detection of IL-6 in diagnostics.
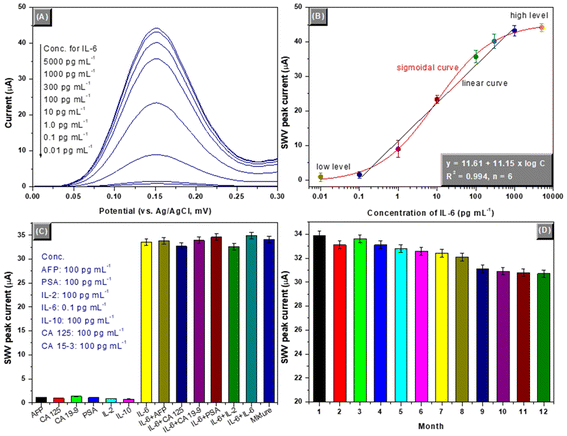 |
| Fig. 4 (A) SWV responses of the electrochemical immunosensors toward different concentrations of IL-6 standards in PBS (pH 5.0, 10 mM) on mAb1-GCE by using mAb2-PBCaNPs as the detection antibodies; (B) calibration plots for the relationship between SWV peak current and decimal logarithm of the IL-6 concentration; (C) the specificity of the electrochemical immunosensor against AFP, CA 125, CA 19-9, PSA, IL-2, IL-10 and target IL-6 (note: the mixture contained the above-mentioned analytes); (D) the storage stability of mAb1-GCE and pAb2-PBCaNPs at 4 °C with intermittent measurement (every month) of 0.1 pg mL−1 of IL-6. | |
Table 1 Comparison of analytical properties including linear range and LOD for the electrochemical immunosensor and other IL-6 detection methods (unit: pg mL−1)
Method |
Linear range |
LOD |
Ref. |
3D spatial amplification |
0.42–24 |
0.27 |
40
|
Electrochemical immunomagnetic assay |
50–1000 |
50 |
41
|
Automated microfluidic immunoassay |
0.7–2652 |
5.0 |
42
|
Fluorescence immunosensor |
50–50 000 |
24 |
9
|
Pressure immunosensor |
0.01–1000 |
0.00333 |
11
|
Electrochemical immunosensor |
0.5–500 |
0.5 |
10
|
Reflectance spectroscopy |
10–10 000 |
10 |
43
|
Electrochemical immunosensor |
30–138 |
5.4 |
44
|
Electrochemical immunosensor |
0.1–1000 |
0.078 |
This work |
Reproducibility, specificity and stability of the immunosensor
In this system, the as-prepared electrochemical immunosensor was disposable, and could not be repeatedly used for IL-6 measurement. Although the immunocomplex can be broken under harsh conditions (e.g., in acidic or alkaline solution), the immobilized mAb1 capture antibodies on the electrode suffer from functional damage. Thus, the reproducibility of the electrochemical immunosensors was investigated on the basis of independently batch-prepared mAb1-GCE and mAb2-PBCaNPs. The reproducibility was evaluated by calculating intra- and inter-batch variation coefficients (CVs, n = 5) toward 3 IL-6 levels. As indicated from Table 2, the CVs were less than 9.58% (n = 5) for intra-assay and 10.59% (n = 5) for inter-assay, respectively, giving good intermediate precision and reproducibility.
Table 2 Intra- and inter-batch variation coefficients (CVs) of mAb1-GCE and mAb2-PBCaNPs for electrochemical immunosensors toward different concentrations of IL-6 standard
Item |
Conc. (pg mL−1) |
Time; conc. (ng mL−1) |
CV (%) |
1 |
2 |
3 |
4 |
5 |
Intra-assay |
1.0 |
0.98 |
1.12 |
1.08 |
0.89 |
0.94 |
9.58 |
50 |
52.3 |
54.1 |
48.2 |
49.1 |
51.2 |
4.68 |
500 |
491 |
509 |
523 |
486 |
514 |
3.09 |
Inter-assay |
1.0 |
1.12 |
0.89 |
0.91 |
1.07 |
1.09 |
10.59 |
50 |
56.2 |
53.1 |
47.2 |
46.8 |
53.4 |
8.07 |
500 |
534 |
512 |
489 |
507 |
481 |
4.12 |
Generally, different proteins or biomarkers are present in human serum. Non-targets might interfere with the results of electrochemical immunosensors. In this case, the as-prepared electrochemical sensor was used against other possible proteins or biomolecules, e.g., alpha-fetoprotein (AFP), cancer antigen 125 (CA 125), cancer antigen 19-9 (CA 19-9), prostate-specific antigen (PSA), interleukin-2 (IL-2) and interleukin-10 (IL-10). The comparison was carried out by assaying the SWV peak currents of the electrochemical immunosensors toward 0.1 ng mL−1 of IL-6 in the presence and absence of non-targets (note: a ratio of 1
:
1000 for target and non-target was used in these cases). Fig. 4C gives the experimental results. Clearly, the electrochemical signals were not fundamentally changed for target IL-6 whether non-targets were present or not. So, the electrochemical immunosensor had high specificity and selectivity for target IL-6.
The storage stabilities of mAb1-GCE and mAb2-PBCaNP were studied at 4 °C for a 12-month period. The prepared immunosensing probes were intermittently used for the detection of 0.1 ng mL−1 of IL-6 (as an example). As indicated from Fig. 4D, the peak currents could remain more than 95% of the initial signal for the first 8 months. After being stored for 12 months, the signals could also preserve ∼90% of the initial current. Such a long-term storage stability was ascribed to the covalent conjugation of antibodies with the electrode and nanoparticles. Thus, the stability of the developed immunosensors is acceptable.
Measurement of human serum specimens
To investigate future applications of electrochemical immunosensors, we collected 15 human serum samples containing target IL-6 from migraine patients according to the ethical standards of our hospital. These samples were first centrifuged (10 min, 5000g) to remove the possible precipitates, and then detected by using the developed immunosensor (note: high-concentration samples were diluted within the linear range of the immunosensors by 10 mM pH 7.4 PBS). For the method comparison study, a commercial human IL-6 ELISA kit was employed to detect human serum specimens. The assayed results are listed in Table 3. Statistical analysis of the experimental results was performed using a t-test between two methods.45–47 As seen from Table 3, the texp values in all samples were less than tcrit (tcrit[4, 0.05] = 2.77). Therefore, the electrochemical immunosensor can be used as an optional method for detection of IL-6 in clinical diagnostics.
Table 3 Analysis of 15 human serum specimens containing target IL-6 from migraine patients using the electrochemical immunosensor and human IL-6 ELISA kit
Sample no. |
Method; conc. (mean ± SD, pg mL−1; n = 3)a |
t
exp
|
Immunosensor |
ELISA kit |
High concentrations were assayed by diluting them with PBS (pH 7.4, 10 mM).
|
1 |
9.6 ± 0.7 |
10.2 ± 0.6 |
1.13 |
2 |
78.2 ± 6.3 |
73.4 ± 5.2 |
1.01 |
3 |
12.3 ± 1.1 |
11.5 ± 0.8 |
1.02 |
4 |
25.5 ± 1.9 |
27.3 ± 2.1 |
1.10 |
5 |
37.8 ± 2.8 |
35.1 ± 2.3 |
1.29 |
6 |
10.7 ± 0.9 |
9.8 ± 0.5 |
1.51 |
7 |
13.2 ± 1.1 |
14.3 ± 0.9 |
1.34 |
8 |
21.6 ± 1.6 |
22.8 ± 1.2 |
1.04 |
9 |
26.7 ± 2.3 |
24.9 ± 1.6 |
1.11 |
10 |
16.4 ± 1.4 |
15.2 ± 1.3 |
1.09 |
11 |
9.2 ± 0.9 |
9.8 ± 0.4 |
1.06 |
12 |
8.3 ± 0.8 |
8.5 ± 0.2 |
0.42 |
13 |
18.3 ± 1.6 |
19.2 ± 1.2 |
0.78 |
14 |
15.4 ± 1.3 |
14.2 ± 1.0 |
1.27 |
15 |
18.3 ± 1.4 |
16.9 ± 1.2 |
1.32 |
Conclusions
In summary, we innovatively fabricated an enzyme-free and sensitive electrochemical immunosensor for the detection of IL-6 by coupling with an electrochemically active nanoparticle label. Experimental results showed that the developed electrochemical immunoassay could exhibit high sensitivity, good reproducibility/specificity, long-term storage stability and acceptable accuracy. Compared with traditional electrochemical immunosensors, several highlights can be simply summarized as follows: (i) the immunoassay can be implemented with a one-step reaction because of two monoclonal antibodies, (ii) this system can be used without the requirements of bioactive enzymes, (iii) the as-synthesized nanoparticles have electrochemical activity, and (iv) the preparation of electrochemical immunosensors is relatively simple using covalent conjugation. These features, as well as the other advantages, such as operational convenience, low cost, and effective analysis at low concentrations, make this system further applicable to other proteins or biomolecules by controlling the target antibody, thus demonstrating the versatility of the proposed assay. Importantly, the developed electrochemical immunosensor can be suitable for use in the mass production of miniaturized devices and open new opportunities for protein diagnostics.
Author contributions
Zhong Chen: conceptualization, investigation, methodology, writing–original draft. Wenhui He: investigation, methodology, writing–review & editing. Renhe Lin: visualization, investigation, methodology, writing–review & editing. Dongzhi Wu: writing–review & editing, methodology, project administration. Xiaoling Jiang: methodology, writing–review & editing, project administration. Yunfan Cheng: visualization, investigation, funding acquisition, supervision, writing–review & editing.
Ethical statement
All the experiments were performed in accordance with the Guidelines of Fujian Medical University, China, and approved by the ethics committee at Fujian Medical University, China. Informed consents were obtained from human participants of this study.
Data availability
Data will be made available on request.
Conflicts of interest
There are no conflicts to declare.
Acknowledgements
This work was supported by the Natural Science Foundation of Fujian Province, China (grant no.: 2023J011548), the Key Clinical Specialty Discipline Construction Program of Fuzhou, Fujian (grant no.: 20220105), and the Grant for In-hospital Scientific Research Projects of Fuzhou Second General Hospital (grant nos: 2024ZY05 & 2024ZY06).
References
- W. Ha and M. Chu, J. Headache Pain, 2024, 25, 95 CrossRef
.
- N. Nathan, A. Ngo and S. Khoromi, J. Clin. Med., 2024, 13, 5380 CrossRef PubMed
.
- T. Kortesi, G. Nagy-Grocz and L. Vecsei, J. Headache Pain, 2024, 25, 129 CrossRef PubMed
.
- L. Biscetti, E. Cresta, L. Cupini, P. Calabresi and P. Sarchielli, Neurobiol. Dis., 2023, 180, 106072 CrossRef PubMed
.
- D. Detchou and U. Barrie, Neurosurg. Rev., 2024, 47, 541 CrossRef PubMed
.
- I. Zaporowaka-Stachowiak, M. Springer, K. Stachowiak, M. Oduah, M. Sopata, K. Wieczorowaka-Tobis and W. Bryl, J. Interferon Cytokine Res., 2024, 44, 45–59 CrossRef PubMed
.
- A. Gholami, N. Dinarvand and M. Hariri, J. Health Popul. Nutr., 2024, 43, 119 CrossRef PubMed
.
- Q. Wang, W. Zheng and S. Pan, Front. Oncol., 2024, 14, 1375362 CrossRef PubMed
.
- M. Zhao, Y. Yang, N. Li, Y. Lv, Q. Jin, L. Wang, Y. Shi, Y. Zhang, H. Shen, L. Li and R. Wu, Langmuir, 2024, 40, 4447–4459 CrossRef PubMed
.
- T. Vessella, H. Zhang, Z. Zhou, F. Cui and H. Zhou, Biosens. Bioelectron., 2023, 237, 115512 CrossRef PubMed
.
- L. Gao, Y. Chen, J. Wei, S. Zhang, J. Yan and H. Dai, Microchem. J., 2023, 195, 109401 CrossRef CAS
.
- Y. Wang, R. Zeng, S. Tian, S. Chen, Z. Bi, D. Tang and D. Knopp, Anal. Chem., 2024, 96, 13663–13671 CrossRef CAS PubMed
.
- D. Wu, J. Tang, Z. Yu, Y. Gao, Y. Zeng, D. Tang and X. Liu, Anal. Chem., 2024, 96, 8740–8746 CrossRef CAS
.
- R. Zeng, M. Qiu, W. Wan, Z. Huang, X. Liu, D. Tang and D. Knopp, Anal. Chem., 2022, 94, 15155–15161 CrossRef CAS PubMed
.
- S. Lv, K. Zhang, L. Zhu, D. Tang, R. Niessner and D. Knopp, Anal. Chem., 2019, 91, 12055–12062 CrossRef CAS PubMed
.
- Z. Yu, H. Gong, Y. Li, J. Xu, J. Zhang, Y. Zeng, X. Liu and D. Tang, Anal. Chem., 2021, 93, 13389–13397 CrossRef PubMed
.
- L. Bai, Y. Shi, X. Zhang, X. Cao, J. Jia, H. Shi and W. Lu, Analyst, 2023, 148, 3359–3370 RSC
.
- J. Shu and D. Tang, Anal. Chem., 2020, 92, 363–377 CrossRef PubMed
.
- J. Shu and D. Tang, Chem. – Asian J., 2017, 12, 2780–2789 CrossRef PubMed
.
- Z. Luo, L. Zhang, R. Zeng, L. Su and D. Tang, Anal. Chem., 2018, 90, 9568–9575 CrossRef PubMed
.
- N. Miyakawa, A. Shinagawa, T. Nakano, S. Ushiba, T. Ono, Y. Kanai, S. Tani, M. Kimura and K. Matsumoto, Microchem. J., 2024, 196, 109594 CrossRef
.
- J. Shin, D. Kim, S. Kim, X. Yang, Y. Kim, E. Kang, J. Song and K. Suh, Microchem. J., 2024, 203, 110919 CrossRef
.
- K. Kunpatee, K. Khantasup, K. Komolpis, A. Yakoh, S. Nuanualsuwan, M. Sain and S. Chaiyo, Biosens. Bioelectron., 2023, 242, 115742 CrossRef
.
- G. Can, B. Perk, B. Citil, Y. Buyuksunetci and U. Anik, Anal. Chem., 2024, 96, 8342–8348 CrossRef
.
- S. Yin and Z. Ma, Expert Rev. Mol. Diagn., 2018, 18, 457–465 CrossRef PubMed
.
- J. Feng, C. Chu and Z. Ma, Electrochem. Commun., 2021, 125, 106970 CrossRef
.
- R. Zeng, K. Lian, B. Su, L. Lu, J. Lin, D. Tang, S. Lin and X. Wang, Angew. Chem., Int. Ed., 2021, 60, 25055–25062 CrossRef PubMed
.
- R. Zeng, Y. Li, X. Hu, W. Wang, Y. Li, H. Gong, J. Xu, L. Huang, L. Lu, Y. Zhang, D. Tang and J. Song, Nano Lett., 2023, 23, 6073–6080 CrossRef CAS
.
- R. Zeng, L. Lin, H. Gong, Y. Li, J. Xu, L. Huang, W. Wang, S. Lin, D. Tang and S. Guo, Chem. Catal., 2023, 3, 100514 CrossRef CAS
.
- H. He, M. Long, Y. Duan and N. Gu, Nanoscale, 2023, 15, 12818–12839 RSC
.
- X. Han, W. He, T. Zhou and S. Ma, Inorg. Chem. Front., 2024, 11, 3707–3730 RSC
.
- Y. Zhang, W. Li, H. Gong, Q. Zhang, L. Yan and H. Wang, Front. Energy, 2024, 18, 160–186 CrossRef
.
- W. Zeng, J. Su, Y. Wang, M. Shou, J. Qian and G. Kai, ChemistrySelect, 2023, 8, e202302655 CrossRef
.
- X. Ma, T. Zhang, X. Wang, T. Zhang, R. Zhang, Z. Xu, M. Ma, Y. Ma and F. Shi, ACS Appl. Nano Mater., 2023, 6, 22568–22593 CrossRef
.
- A. Shan, A. Idrees, W. Zaman, A. Mohsin, Z. Abbas, F. Stadler and S. Lyu, Environ. Res., 2024, 245, 118050 CrossRef
.
- M. Zhang, X. Li and H. Lin, APL Mater., 2024, 12, 091101 CrossRef
.
- D. Tang and J. Ren, Anal. Chem., 2008, 80, 8064–8070 CrossRef PubMed
.
- W. Lu, S. Xue, X. Liu, C. Bao and H. Shi, Microchem. J., 2024, 196, 109606 CrossRef
.
- W. Lu, R. Zhang, X. Zhang, Y. Shi, Y. Wang and H. Shi, Analyst, 2023, 148, 5469–5475 RSC
.
- Z. Fu, C. Hao, X. Fei and H. Ju, J. Immunol. Methods, 2006, 312, 61–67 CrossRef
.
- M. Yamaguchi and V. Shetty, Sens. Actuators Rep., 2024, 8, 100213 CrossRef
.
- G. Buckey, O. Owens, H. Richards and D. Cliffel, Sens. Diagn., 2024, 3, 1039–1043 RSC
.
- J. Yang, M. Barbhuiya, C. Hamilton, K. Robyak and Y. Zhu, Ann. Clin. Lab. Sci., 2024, 54, 288–393 Search PubMed
.
- D. Tsounidi, V. Tsaousis, N. Xenos, C. Kroupis, P. Motsatsou, V. Christianidis, D. Goustouridis, I. Raptis, S. Kakabakos and P. Petrou, Talanta, 2023, 258, 124403 CrossRef
.
- R. Cancelliere, A. Tinno, A. Lellis, G. Contini, L. Micheli and E. Signori, Biosens. Bioelectron., 2022, 213, 114467 CrossRef PubMed
.
- R. Liu, F. Shi, Y. Xia, H. Zhu, J. Cao, K. Peng, C. Ren, J. Li and Z. Yang, Chin. Chem. Lett., 2024, 35, 109664 CrossRef
.
- J. Zhan, F. Shi, J. Li, H. Zeng, M. Chen, X. Hu and Z. Yang, Chin. Chem. Lett., 2023, 34, 108791 CrossRef
.
Footnote |
† These authors have contributed equally to this work. |
|
This journal is © The Royal Society of Chemistry 2025 |
Click here to see how this site uses Cookies. View our privacy policy here.