DOI:
10.1039/D4AN01341G
(Paper)
Analyst, 2025,
150, 87-93
A novel ratiometric fluorescent probe based on an internal reference of lanthanide/nucleotide for alkaline phosphatase detection†
Received
15th October 2024
, Accepted 18th November 2024
First published on 22nd November 2024
Abstract
Based on the specific hydrolytic ability of alkaline phosphatase (ALP), a novel biocompatible ratiometric lanthanide fluorescent probe based on an internal reference (CIP@SiO2-Ce/ATP-Tris) was constructed with Ce3+ as the central ion, adenosine triphosphate (ATP) as the ligand, Tris as the auxiliary ligand and ciprofloxacin (CIP) encapsulated into SiO2 nanoparticles as the reference signal. The fluorescent probe emits characteristic fluorescence at 363 nm belonging to Ce3+ as the working signal and at 435 nm belonging to CIP as the reference signal. Dephosphorylation disrupted the coordination of Ce/ATP-Tris with the enzymatic reaction of ALP, which resulted in fluorescence quenching of Ce3+. The reference fluorescence was kept stable because of the protective effect of encapsulation by SiO2. The biosensor analysis method was achieved by comparing the relationship between I435/I363 and ALP concentration. The detection limit is 0.0025 U L−1, and the linear range of detection is 0.1–20 U L−1. It was subsequently used to detect ALP in samples of fetal bovine serum and human serum, and promising results were obtained.
Introduction
The hydrolase alkaline phosphatase (ALP) is found in many biological tissues and cells. It has excellent catalytic ability in alkaline environments and can dephosphorylate monophosphate in substrates such as proteins, phosphoric acid, and small molecules.1,2 ALP is a group of isoenzymes, six of which have been identified from the liver, bone cells, placenta, and cancerous cells. The alkaline phosphatase used in this work is extracted from the bovine small intestine. As an essential biomarker, its normal level in the human body is 45–125 U L−1, and it can be an important diagnostic indicator for many diseases and cancers, such as osteoporosis, biliary obstruction, cirrhosis, diabetes, bone cancer, liver cancer, breast cancer and prostate cancer.3–7 In addition, ALP is often selected as an enzyme tool to generate or amplify the assay signal in molecular biology and enzyme-linked immunosorbent assays.8 Therefore, it is of great significance to establish an ultrasensitive method to detect ALP activity for enzyme activity assay and biosensing research.
The methods developed to determine ALP activity include chromatography, the SERS method, electrochemical analysis, colorimetry, and fluorescence analysis.9–14 For example, Sun and co-workers reported an ALP activity assay based on the unique chromogenic interaction of diethanolamine (DEA) with 4-aminophenol (AP) that came from the dephosphorylation of 4-aminophenyl phosphate (APP).15 Ding et al. reported ultrasmall Ru nanoparticles on carbon nitride with high peroxidase-mimicking activity for the colorimetric detection of alkaline phosphatase.16 However, a chromatographic approach requires sophisticated instruments and complicated pre-treatment as an early detection method. The pretreatment in the SERS method is complicated. The signal of the electrochemical process is weak, and may require the participation of various enzymes. In contrast, fluorescence analysis is convenient, sensitive, and selective, has low background interference and can be monitored in real time. It is a more suitable ALP detection method because of its potential application in endogenous ALP monitoring. Since it is not fluorescent by itself, it is necessary to introduce appropriate fluorescent substances into the analytical system as probes. Organic fluorescent molecules, quantum dots, metal nanoclusters, and polydopamine nanoparticles have been applied to the qualitative and quantitative detection of ALP.17–20 For example, Huang et al. designed a dual-mode strategy for ALP detection based on the electronic effect of PNP.17 Xiao et al. designed the reversible quenching effect of manganese dioxide (MnO2) nanosheets on the fluorescence of F-PDA nanoparticles to detect ALP through the conversion of substrate ascorbic acid 2-phosphate to ascorbic acid, and the AA-triggered reduction of MnO2 nanosheets to Mn2+.19 However, the utilization of these fluorescent materials frequently entails complex synthesis procedures, the use of expensive and hazardous materials, and intricate signal-generation mechanisms. Therefore, there is still a need to design efficient, harmless fluorescence assay methods with simple and well-defined tools.
In this work, a ratiometric lanthanide fluorescent probe based on an internal reference was developed to create a fluorescence sensor for ALP, as shown in Scheme 1. Nanosilica (SiO2), which was generated by adding TEOS (as a silicon source) into ethanol and ammonia, encapsulated hydrophilic CIP during synthetic polycondensation to constitute CIP@SiO2 as the reference fluorescence. Then, based on the unique optical properties and intrinsically good biocompatibility of lanthanide/nucleotide coordination polymers, nucleic acid ligand polymers were grown on the surface of the silicon spheres and physically adsorbed onto them. The Ce3+ ion was selected as the central ion, ATP as the coordination molecule, and Tris as the auxiliary ligand to construct Ce/ATP-Tris as the sensing fluorescence. As a natural molecule in living organisms, ATP contains a variety of metal coordination functional groups. Because phosphate groups have a high affinity for lanthanide ions, they can be used as an excellent ligand to provide energy for the luminescence of central ions.21,22 After the addition of ALP to the system, ATP was converted to adenosine and inorganic orthophosphate by enzymatic dephosphorylation, and the “antenna effect” between the Ce3+ ion and ATP was destroyed, resulting in fluorescence quenching at 363 nm attributed to Ce/ATP-Tris. Because the buffer solution maintained the pH and polarity of the test system and encapsulation in SiO2 had a protective effect, the presence of ALP had no effect on the CIP fluorescence intensity at 435 nm. Therefore, the concentration of ALP could be obtained from the ratio of I435/I363. The enzymatic reaction simplified the detection process and did not require a particular or expensive reagent. Taking advantage of the high specificity of the enzymatic reaction, a simple, highly selective, and sensitive assay was achieved, which was easily applied in practice.
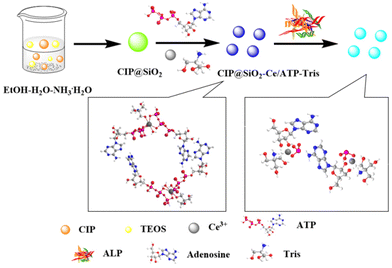 |
| Scheme 1 Schematic diagram of the preparation and sensing process of the CIP@SiO2-Ce/ATP-Tris probe. | |
Experimental section
Chemicals and reagents
Cerium nitrate hexahydrate (Ce(NO3)3·6H2O, 99.99%), BSA, ethyl orthosilicate (TEOS), and metal salts (CuCl2) were obtained from Shanghai Macklin Biochemical Co., Ltd. Glycine (Gly), Vit B13, leucine (Leu), arginine (Arg), pepsin, trypsin, alanine (Ala), tyrosine (Tyr), histidine (His), phenylalanine (Phe), and lactose (Lac) were received from Aladdin Chemical Reagent Co., Ltd. Tris(hydroxymethyl)aminomethane (Tris), ciprofloxacin (CIP), ammonia monohydrate (NH3·H2O), and adenosine triphosphate (ATP) were supplied by Sinopharm Chemical Reagent Co., Ltd. Alkaline phosphatase (ALP) was purchased from Merck Chemical Technology (Shanghai) Co., Ltd.
Apparatus
Field scanning electron microscopy–energy dispersive X-ray spectroscopy (FESEM-EDXS, GeminiSEM 500, Germany) and high-resolution transmission electron microscopy (HR-TEM, JEM-2100, JEOL, Japan) with an acceleration voltage of 100 kV were used to analyze the structural morphology. The chemical structure of the probe was characterized by its FT-IR spectrum using the KBr pellet method (Nicolet 6700 FTIR, Thermo, USA). The spectral properties of the probe were characterized by UV-Vis spectrophotometry (Lambda 950, Varian, USA) and a Lumina fluorescence spectrometer (PE LS-55, PerkinElmer, USA). X-ray photoelectron spectroscopy (XPS, ESCALAB250, Thermo Fisher, UK) was used to determine the elemental composition of the probe.
Preparation of CIP@SiO2-Ce/ATP-Tris
In 1968, Stöber et al. developed the well-known Stöber synthesis method,23 which used TEOS as a silicon source to prepare homogeneously dispersed spherical SiO2 nanoparticles in an ethanol–water mixture containing ammonia. The synthesis method included two stages, namely a hydrolysis reaction and a polycondensation reaction. CIP@SiO2 was prepared according to this method. 331.34 mg of CIP was dissolved in 201.5 mL of mixed solution (anhydrous ethanol
:
deionized water
:
ammonia monohydrate = 160 mL
:
40 mL
:
1.5 mL). A transparent liquid was obtained by treatment with ultrasound for 30 min. The reaction mixture was then mechanically agitated at room temperature for an overnight period while 0.4 mL of TEOS was added drop by drop. After the reaction was finished, it was centrifuged to collect the product, which was then cleaned with deionized water and ethanol. For later use, CIP@SiO2 was dispersed in 3 mL of deionized water.
Next, 2.171 g of Ce(NO3)3·6H2O and 634 mg of ATP were completely dissolved by ultrasound in deionized water (20 mL) and Tris-HCl solution (10 mL, 50 mmol L−1, pH = 7.1), respectively. 8.33 mL of ATP solution was added to 2 mL of the above CIP@SiO2 dispersion solution under magnetic stirring for 5 min. Then, 16.67 mL of Ce(NO3)3·6H2O solution was added drop by drop and stirred for 60 min at room temperature. Finally, CIP@SiO2-Ce/ATP-Tris was collected and washed with deionized water. The probe was mixed with deionized water and kept at 4 °C in a refrigerator.
Fluorescence spectral analysis of ALP
The CIP@SiO2-Ce/ATP-Tris probe solution (0.012 g mL−1) was mixed with standard ALP solutions of varying concentrations (0–120 U L−1). Then, a solution of the mixture was made up to 3 mL with Tris-HCl buffer (50 mmol L−1, pH 7.1). The mixture was then incubated at 37 °C for 60 min. Finally, the incubated mixture was investigated through fluorescence spectroscopy with excitation at 295 nm.
Results and discussion
Characterization of CIP@SiO2-Ce/ATP-Tris
Fig. 1 shows the structural morphology images of CIP@SiO2, Ce/ATP-Tris, and CIP@SiO2-Ce/ATP-Tris by SEM (Mag = 50
000×, WD = 4.4 mm, EHT = 3.00 kV, Signal A = InLens) and TEM. It can be seen that CIP@SiO2 exhibited smooth spheres with a diameter of about 100 nm. Meanwhile, Ce/ATP-Tris nanomaterials consisted of spherical particles with particle sizes in the range of 10 to 30 nm. Tris was added as an auxiliary ligand to form a network structure, which enabled their aggregation. Because of the form of network structure aggregation by nanomaterials, the aggregation had no effect on the fluorescence response of the CIP@SiO2-Ce/ATP-Tris sensing system. The images of each element of CIP@SiO2 are clearly shown in Fig. S1† through TEM. The N and F elements are derived from the CIP molecules. The CIP was encapsulated using the Stöber synthesis method to form a silicon sphere, and then the nucleic acid ligand polymers were grown on the surface of the silicon spheres and physically adsorbed onto them, resulting in the formation of a ratiometric fluorescent probe with internal reference ratios.
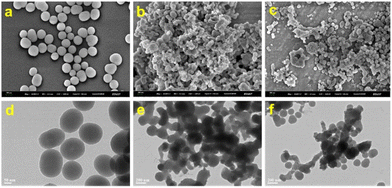 |
| Fig. 1 SEM images of CIP@SiO2 (a) (the scale bar is 100 nm), Ce/ATP-Tris (b) (the scale bar is 200 nm) and CIP@SiO2-Ce/ATP-Tris (c) (the scale bar is 200 nm); and TEM images of CIP@SiO2 (d) (the scale bar is 50 nm), Ce/ATP-Tris (e) (the scale bar is 200 nm) and CIP@SiO2-Ce/ATP-Tris (f) (the scale bar is 200 nm). | |
The elemental compositions of CIP@SiO2 and CIP@SiO2-Ce/ATP-Tris were characterized by EDS (Fig. 2). From Fig. 2a, CIP@SiO2 consisted mainly of C, N, O, F and Si elements, with contents of 6.76, 0.75, 37.66, 0.13 and 54.69 wt%. The successful synthesis of CIP@SiO2-Ce/ATP-Tris resulted in a significant increase in N, P and Ce content, which were 3.7, 7.67 and 14.35 wt%, respectively (Fig. 2b). The elemental compositions were also characterized by the XPS spectra, as shown in Fig. S2 and S3.† The high-resolution spectrum of Ce 3d was generally composed of two multi-peaked broad bands (V and U bands), corresponding to 3d5/2 and 3d3/2 spin–orbit splitting, which could be decomposed into four peaks.24–26 The peaks at 904.3 eV and 885.5 eV with higher binding energies correspond to U′ and V′, which were attributed to the Ce 3d94f1 O 2p6 final state. The peaks with lower binding energies of 900.8 eV and 882.2 eV correspond to U0 and V0, which were attributed to Ce 3d94f2 O 2p5. Two significant peaks can be seen in the high-resolution spectra of C 1s at 284.7 eV (C–OH/C–O–C) and 286.5 eV (C
N). The existence of C–N/C
N and N–H was detected in the high-resolution spectrum of N 1s. The presence of PO4 was detected in the high-resolution spectrum of P 2p.
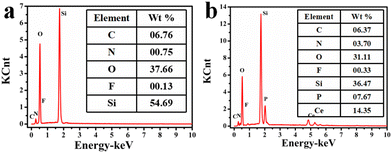 |
| Fig. 2 Energy dispersive spectrometer (EDS) spectra of CIP@SiO2 (a) and CIP@SiO2-Ce/ATP-Tris (b). | |
The FT-IR spectra of the probe are displayed in Fig. 3(i). The absorption peaks at 1700, 1500 and 1410 cm−1 correspond to the stretching vibration of the phosphate hydroxyl groups in the ATP molecule and nucleic acid bases N7–C8, for which the absorption peaks were slightly shifted due to the involvement of coordination (lines b, c and e). The stretching vibration of the CIP ring structure was responsible for the absorption peaks at 1650 and 1625 cm−1 (lines d and e). By comparing the locations of the typical absorption peaks, it can be seen that the CIP was physically encapsulated in SiO2 and had no discernible impact on the coordination between Ce3+ and the ligand. UV-Vis absorption spectra were further used to confirm the formation of the CIP@SiO2-Ce/ATP-Tris probe and to investigate the mechanism of interaction between the probe and ALP from Fig. 3(ii). The two significant absorption peaks at 330 and 375 nm shown by CIP can be attributed to the n–π* transition of the C
C–C
O group and the π–π* transition of the C
N and C
O group, respectively (lines a and e). CIP also showed a minor absorption peak at 277 nm. ATP showed strong absorption at 275 nm, which is mainly ascribed to the π–π* transition in adenine in the nucleic acid base adenosine. It shifted slightly to 260 nm after participating in coordination (lines c and e). When ALP was added, the coordination structure of Ce/ATP-Tris was disrupted, and Tris was released. Lines e and f show that the absorption peak at 210 nm was strengthened while that at 260 nm was weaker.
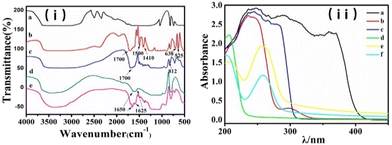 |
| Fig. 3 (i). FT-IR spectra of Ce(NO3)3 (a), ATP (b), Ce/ATP-Tris (c), CIP@SiO2 (d) and CIP@SiO2-Ce/ATP-Tris (e); (ii). UV-Vis absorption spectra of CIP (a) (10 mM), Ce3+ (b) (10 mM), ATP (c) (10 mM), Tris (d) (50 mM), CIP@SiO2-Ce/ATP-Tris (e) (3.33 mg mL−1) and CIP@SiO2-Ce/ATP-Tris with ALP (f) (3.33 mg mL−1). | |
Fluorescence property of CIP@SiO2-Ce/ATP-Tris
To optimize the emission signal of the internal reference fluorescent probe, the fluorescence spectra of the probe were tested with different excitation wavelengths from 250 nm to 350 nm, as shown in Fig. S4.† When the excitation was at 280 nm, the intensity of emission at 435 nm assigned to CIP was most robust. However, the emission at 363 nm assigned to Ce/ATP-Tris was the most robust with excitation at 295 nm. As shown in Fig. 4A, the fluorescence intensity ratio of I435/I363 of these two sites was chosen to reach the highest value, and the excitation wavelength was chosen to be 295 nm. Fig. 4B shows the fluorescence spectra of different components at the optimal excitation wavelength. The fluorescence intensity of Ce3+ ions (Fig. 4Ba) was weak and lacked nearly all luminescence, but there was an intense emission peak at 363 nm after forming a coordination polymer with the ligand (Fig. 4Bc) in contrast to Ce3+ ions (Fig. 4Ba). Due to the large π-bond conjugated system and strong planar stiffness of the CIP molecule, which may produce distinctive and stable fluorescence via the intramolecular proton transfer principle, there was an outstanding emission peak at 435 nm (Fig. 4Bb). The fluorescence intensity of Ce/ATP-Tris was obviously decreased after the addition of ALP (Fig. 4Bd) in contrast to Ce/ATP-Tris (Fig. 4Bc). While the fluorescence intensity of CIP@SiO2-Ce/ATP-Tris at 435 nm assigned to CIP remained unchanged, and that at 363 nm assigned to Ce/ATP-Tris obviously decreased with the addition of ALP (Fig. 4Bf) in contrast to the CIP@SiO2-Ce/ATP-Tris probe (Fig. 4Be). Thus, the ratio of I435/I363 was chosen as the response signal.
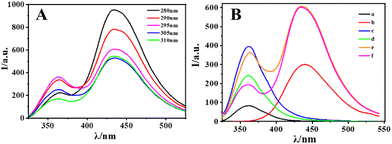 |
| Fig. 4 Emission spectra of CIP@SiO2-Ce/ATP-Tris solution (12 mg mL−1) with different excitation wavelengths (A) and emission spectra of different component solutions (B) (a: 10 mM Ce3+, b: 10 mM CIP, c: 10 mM Ce/ATP-Tris, d: 10 mM Ce/ATP-Tris + ALP, e: 3.33 mg mL−1 CIP@SiO2-Ce/ATP-Tris, f :3.33 mg mL−1 CIP@SiO2-Ce/ATP-Tris + ALP). | |
Optimization of the fluorescence experimental parameters
The experimental conditions were designed to acquire the optimal parameters in order to achieve sensitive detection of ALP, such as the ratio of Ce3+ to CIP, pH value, enzymatic time and probe stability. Fig. S5a† shows the fluorescence intensities of the CIP@SiO2-Ce/ATP-Tris probe at different Ce3+/CIP ratios in the range 325–525 nm. In order to achieve an appropriate fluorescence intensity and ratio, Ce3+/CIP = 5 was finally selected as the best ratio for the preparation of the material. It is known that ALP shows excellent hydrolytic catalytic ability only in an alkaline solution environment, while nano silica will be soluble in strongly alkaline solutions. The pH level of the reaction system will also alter the fluorescence of CIP at the same time, which has a significant impact on the outcomes of the detection. Therefore, the range of pH for the buffer solution went from 7.0 to 9.0. As shown in Fig. S5b,† the probe fluorescence intensity ratio reached its maximum at pH = 7.1; therefore, the pH value of 7.1 was selected for further studies. The enzymatic reaction needs to be incubated at a specific temperature for a period, which differs from the rapid response time of small molecules. The results are shown in Fig. S6a.† Following the addition of ALP, the fluorescence intensity ratio of the reaction system gradually increased along with the lengthening of the enzymatic reaction time. At 363 nm, Ce/ATP-Tris fluorescence steadily declined and stabilized after 60 minutes. The optimal enzymatic reaction time was therefore established to be 60 minutes. Furthermore, after being kept at 4 °C for 28 days, the fluorescence intensity of the CIP@SiO2-Ce/ATP-Tris probe solution was well maintained (Fig. S6b†).
Selectivity and interference for ALP detection
To verify the sensing ability of this method in real samples, the specificity of CIP@SiO2-Ce/ATP-Tris for the detection of ALP needed to be investigated (Fig. 5). Some common enzymes and proteins (pepsin, trypsin, BSA), amino acids (Gly, Leu, His, Tyr, Phe, Arg, Ala) and other interfering substances (Cu2+, Lac, Vit B13) were selected as potential interferences. The trial was conducted under identical conditions, with an ALP concentration of 50 U L−1, enzyme and protein concentrations of 2 mg mL−1, and the concentration of other substances was 1 mmol L−1. Based on the specific enzymatic hydrolysis under alkaline conditions, the results showed that the probe responded only to ALP. As expected, the interfering substances could not affect the stable CIP@SiO2-Ce/ATP-Tris structure, showing satisfactory selectivity for the detection of ALP, which also indirectly indicated the specificity of the enzymatic action.
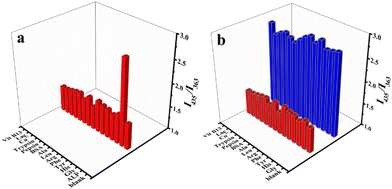 |
| Fig. 5 Selectivity experiments of various potential interfering substances (a) and the effects of different interfering substances on the fluorescence intensity of CIP@SiO2-Ce/ATP-Tris with (blue) and without (red) ALP (b) (ALP concentration of 50 U L−1; enzyme and protein concentrations of 2 mg mL−1; other substances concentration of 1 mmol L−1). | |
Analytical performance and applications
The fluorescence response of the CIP@SiO2-Ce/ATP-Tris sensing system to various concentrations of ALP (0–120 U L−1) was examined under optimal conditions with satisfactory selectivity. The investigated results are shown in Fig. 6. As the ALP concentration in the Tris-HCl buffer (pH 7.1) of the probe (0.012 g mL−1) was increased from 0 to 120 U L−1, the “antenna effect” of ATP to the central cerium ion was disrupted due to the dephosphorylation of ALP. The luminescence Ce/ATP-Tris was gradually dissociated, resulting in a gradual decrease in the fluorescence emission at 363 nm. In the meantime, the fluorescence of CIP at 435 nm remained unchanged because of the protection from silica and the stable solution environment. According to Fig. 6b, there was a good linear relationship between ALP concentration and the ratio (I435/I363) of the fluorescence intensity of this probe at 435 nm and 363 nm, which was in the range of 0.1 to 20 U L−1. The regression equation was y = 0.0694x + 1.458, and the correlation coefficient (R2) was 0.9983. The detection limit of ALP was calculated to be 0.0025 U L−1 based on the 3σ/m criterion, and the probe showed good sensitivity compared with previously reported ALP assays. As shown in Table 1, the working ranges and detection limits of each detection method were compared. Introducing CIP as the internal reference fluorescence improves the sensitivity and competitiveness of this approach.
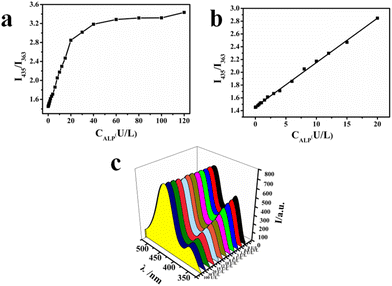 |
| Fig. 6 Fluorescence responses of CIP@SiO2-Ce/ATP-Tris solution to various ALP concentrations (a) (0, 0.1, 0.4, 0.6, 0.8, 1.0, 1.5, 2, 3, 4, 6, 8, 10, 12, 15, 20, 30, 40, 60, 80, 100, 120 U L−1); linear relationship of fluorescence intensity at 435 and 363 nm as the response to the ALP concentration (b); and emission spectra of CIP@SiO2-Ce/ATP-Tris solution with ALP of different concentrations (c). | |
Table 1 Performance of the new approach compared to previously published ALP assays from other literature
Analytical method |
Linear range (U L−1) |
Detection limit (U L−1) |
Ref. |
DNA-templated CuNPs |
0.01–5 |
0.0098 |
27
|
Ru(bpy)32+@ZIF-90 |
0.5–100 |
0.04 |
28
|
BSA-CdS QDs |
0–0.95 |
0.05 |
29
|
G4/NMM |
2.5–25 |
0.81 |
30
|
β-CD-CQDS |
3.4–100 |
0.9 |
31
|
AuNPs/PEDOT:PSS |
0.1–120 |
0.03 |
32
|
Fe/NC-SAs |
0.1–1.5 |
0.05 |
33
|
CIP@SiO2-Ce/ATP-Tris |
0.1–20 |
0.0025 |
This work |
A standard addition experiment measured ALP in fetal bovine serum and human serum samples to further evaluate the practical applicability of CIP@SiO2-Ce/ATP-Tris. The original serum samples were diluted up to 1000 times with Tris-HCl buffer under the previously established ideal experimental conditions for the assay (Table 2). The concentrations of ALP in fetal bovine serum and human serum were 83.1 and 60.4 U L−1 with our fluorescence detection, respectively. The relative standard deviations were less than 5.8%, and the ALP recoveries ranged from 88.0% to 101.5%. The results verified the high accuracy and excellent performance of the proposed internal reference fluorescent probe in fundamental sample analysis, which has the potential to be applied to complex samples.
Table 2 Recovery experiment results of ALP determination in fetal bovine serum and human serum samples using the proposed method
Sample |
Added ALP (U L−1) |
Found ALP (U L−1) |
Average recovery (%) |
RSD (%, n = 3) |
Fetal bovine serum (0.1%) |
— |
0.08 |
— |
2.6 |
1.00 |
0.96 |
88.0 |
4.7 |
1.20 |
1.28 |
99.4 |
1.6 |
1.60 |
1.61 |
95.6 |
4.9 |
2.00 |
2.08 |
100.1 |
5.8 |
Human serum (0.1%) |
— |
0.06 |
— |
3.0 |
0.40 |
0.43 |
92.5 |
2.5 |
1.40 |
1.39 |
94.7 |
4.8 |
1.80 |
1.86 |
100.1 |
0.3 |
2.00 |
2.09 |
101.5 |
0.7 |
Conclusions
In this study, a novel biocompatible ratiometric lanthanide fluorescent probe based on an internal reference (CIP@SiO2-Ce/ATP-Tris) was constructed with Ce3+ as the central ion, adenosine triphosphate (ATP) as the ligand, Tris as the auxiliary ligand and ciprofloxacin (CIP) encapsulated into SiO2 nanoparticles as the reference signal. ATP was recognized as a natural substrate for phosphatases and acted as an excellent “antenna” with the help of Tris to sensitize the luminescence of Ce3+. The catalytic hydrolysis of ALP led to fluorescence signal quenching. The introduction of CIP improved the detection sensitivity and stability, and I435/I363 demonstrated good correlation with ALP activity over a broad range of 0.1–20 U L−1 with a detection limit of 0.0025 U L−1, which was equivalent to or lower than several previously reported approaches. The ensuing steps of substrate hydrolysis and signal production could be carried out simultaneously in one step because the fluorescence sensor was pre-synthesised using a straightforward self-assembly reaction, making the method straightforward and tractable. The effective use of quantitative ALP detection in fetal bovine serum samples showed great application for enzyme activity assay and biosensing research.
Data availability
The authors confirm that the data supporting the findings of this study are available within the article or its ESI.†
Conflicts of interest
The authors declare no competing financial interest.
Acknowledgements
This work was financially supported by the Scientific Instrumentation Program of Shanghai Science and Technology Innovation Action Plan (21142201600), the National Key R&D Program of China (No. 2021YFF0701900), the National Natural Science Foundation of China (21707034), and the Science and Technology Commission of Shanghai Municipality (No. 20142200900).
References
- J. L. Millan, Alkaline Phosphatases: Structure, Substrate Specificity and Functional Relatedness to Other Members of A Large Superfamily of Enzymes, Purinergic Signalling, 2006, 2(2), 335–341 CrossRef
.
- U. Sharma, D. Pal and R. Prasad, Alkaline Phosphatase: An Overview, Indian J. Clin. Biochem., 2014, 29(3), 269–278 CrossRef PubMed
.
- C. Jin, J. X. He and J. M. Zou,
et al., Phosphorylated Lipid-conjugated Oligonucleotide Selectively Anchors on Cell Membranes with High Alkaline Phosphatase Expression, Nat. Commun., 2019, 10, 2704 CrossRef PubMed
.
- A. L. Brichacek and C. M. Brown, Alkaline Phosphatase: A Potential Biomarker for Stroke and Implications for Treatment, Metab. Brain Dis., 2019, 34(1), 3–19 CrossRef PubMed
.
- Y. Liu, P. M. Cavallaro and B. M. Kim,
et al., A Role for Intestinal Alkaline Phosphatase in Preventing Liver Fibrosis, Theranostics, 2021, 11(1), 14–26 CrossRef
.
- N. Alonso, B. Larraz-Prieto and K. Berg,
et al., Loss-of-Function Mutations in the ALPL Gene Presenting with Adult Onset Osteoporosis and Low Serum Concentrations of Total Alkaline Phosphatase, J. Bone Miner. Res., 2020, 35(4), 657–661 CrossRef
.
- Y. Y. Xiao, J. Lu and W. Chang,
et al., Dynamic Serum Alkaline Phosphatase is An Indicator of Overall Survival in Pancreatic Cancer, BMC Cancer, 2019, 19(1), 785 CrossRef PubMed
.
- M. K. Wang, S. Wang and X. M. Xie,
et al., Ag-Ion-Modified Au Nanoclusters for Fluorometric Analysis of Alkaline Phosphatase, ACS Appl. Nano Mater., 2020, 3(6), 6034–6042 CrossRef
.
- J. Zheng, D. D. Song and H. Chen,
et al., Enhanced Peroxidase-like Activity of Hierarchical MoS2 -decorated N-doped Carbon Nanotubes with Synergetic Effect for Colorimetric Detection of H2O2 and Ascorbic Acid, Chin. Chem. Lett., 2020, 31(5), 1109–1113 CrossRef
.
- Z. W. Tang, H. F. Zhang and C. B. Ma,
et al., Colorimetric Determination of the Activity of Alkaline Phosphatase Based on the Use of Cu(II)-modulated G-quadruplex-based DNAzymes, Microchim. Acta, 2018, 185(2), 109 CrossRef
.
- H. Liu, L. Wei and J. H. Hua,
et al., Enzyme Activity-modulated Etching of Gold Nanobipyramids@MnO2 Nanoparticles for ALP Assay Using Surface-enhanced Raman Spectroscopy, Nanoscale, 2020, 12(18), 10390–10398 RSC
.
- W. B. Wang, J. Lu and L. L. Hao,
et al., Electrochemical Detection of Alkaline Phosphatase Activity through Enzyme-catalyzed Reaction Using Aminoferrocene as An Electroactive Probe, Anal. Bioanal. Chem., 2021, 413(7), 1827–1836 CrossRef CAS PubMed
.
- X. C. Huang, X. B. Bian and L. F. Chen,
et al., Highly Sensitive Homogeneous Electrochemiluminescence Biosensor for Alkaline Phosphatase Detection Based on Click Chemistry-Triggered Branched Hybridization Chain Reaction, Anal. Chem., 2021, 93(29), 10351–10357 CrossRef CAS PubMed
.
- C. X. Chen, Q. Yuan and P. J. Ni,
et al., Fluorescence Assay for Alkaline Phosphatase Based on ATP Hydrolysis-triggered Dissociation of Cerium Coordination Polymer Nanoparticles, Analyst, 2018, 143, 3821–3828 RSC
.
- J. Sun, J. H. Zhao and X. F. Bao,
et al., Alkaline Phosphatase Assay Based on the Chromogenic Interaction of Diethanolamine with 4-Aminophenol, Anal. Chem., 2018, 90(10), 6339–6345 CrossRef CAS PubMed
.
- Z. Y. Ding, Z. Li and X. X. Zhao,
et al., Self-deposited ultrasmall Ru nanoparticles on carbon nitride with high peroxidase-mimicking activity for the colorimetric detection of alkaline phosphatase, J. Colloid Interface Sci., 2023, 631, 86–95 CrossRef CAS
.
- X. M. Huang, M. J. Lan and J. Wang,
et al., A Dual-mode Strategy for Sensing and Bio-imaging of Endogenous Alkaline Phosphatase Based on the Combination of Photoinduced Electron Transfer and Hyperchromic Effect, Anal. Chim. Acta, 2021, 1142, 65–72 CrossRef CAS
.
- L. Liu, H. Jiang and X. M. Wang, Alkaline Phosphatase-responsive Zn2+ Double-triggered Nucleotide Capped Gold Nanoclusters/Alginate hydrogel with Recyclable Nanozyme Capability, Biosens. Bioelectron., 2021, 173, 112786 CrossRef PubMed
.
- T. Xiao, J. Sun and J. H. Zhao,
et al., FRET Effect between Fluorescent Polydopamine Nanoparticles and MnO2 Nanosheets and Its Application for Sensitive Sensing of Alkaline Phosphatase, ACS Appl. Mater. Interfaces, 2018, 10(7), 6560–6569 CrossRef
.
- D. D. La, S. V. Bhosale and L. A. Jones,
et al., Tetraphenylethylene-Based AIE-Active Probes for Sensing Applications, ACS Appl. Mater. Interfaces, 2018, 10(15), 12189–12216 CrossRef
.
- L. Lu, J. C. Si and Z. F. Gao,
et al., Highly Selective and Sensitive Electrochemical Biosensor for ATP Based on the Dual Strategy Integrating the Cofactor-dependent Enzymatic Ligation Reaction with Self-cleaving DNAzyme-amplified Electrochemical Detection, Biosens. Bioelectron., 2015, 63, 14–20 CrossRef PubMed
.
- C. Xu, Z. Liu and L. Wu,
et al., Nucleoside Triphosphates as Promoters to Enhance Nanoceria Enzyme-like Activity and for Single-Nucleotide Polymorphism Typing, Adv. Funct. Mater., 2014, 24(11), 1624–1630 CrossRef
.
- W. Stöber, A. Fink and E. Bohn, Controlled Growth of Monodisperse Silica Spheres in the Micron Size Range, J. Colloid Interface Sci., 1968, 26, 62–69 CrossRef
.
- E. Bêche, P. Charvin and D. Perarnau,
et al., Ce 3d XPS investigation of cerium oxides and mixed cerium oxide (CexTiyOz), Surf. Interface Anal., 2008, 40, 264–267 CrossRef
.
- D. Weihua, S. Naoto and Z. Haibo,
et al., Fluorescent sensing of colloidal CePO4: Tb nanorods for rapid, ultrasensitive and selective detection of vitamin C, Nanotechnology, 2010, 21, 365501 CrossRef
.
- J. J. He, Y. H. Xu and W. Wang,
et al., Ce(III) nanocomposites by partial thermal decomposition of Ce-MOF for effective phosphate adsorption in a wide pH range, Chem. Eng. J., 2020, 379, 122431 CrossRef
.
- Y. Wang, Y. Yan and X. F. Liu,
et al., An Exonuclease I-Aided Turn-Off Fluorescent Strategy for Alkaline Phosphatase Assay Based on Terminal Protection and Copper Nanoparticles, Biosensors, 2021, 11(5), 139 CrossRef
.
- C. P. Du, Y. Wang and K. L. Pei,
et al., An electrochemiluminescence dual “turn-on” strategy for alkaline phosphatase detection using a dual quenching Ru(bpy)32+ encapsulated zeolite imidazole metal organic framework, Chem. Commun., 2022, 58(86), 12114–12117 RSC
.
- Y. Upadhyay, S. Bothra and R. Kumar,
et al., Mimicking biological process to detect alkaline phosphatase activity using the vitamin B6 cofactor conjugated bovine serum albumin capped CdS quantum dots, Colloids Surf., B, 2020, 185, 110624 CrossRef PubMed
.
- L. Y. Ma, Y. Xiao and H. J. Fang,
et al., Highly Sensitive Alkaline Phosphatase Biosensor Based on Internal Filtration Effect between G-Quadruplex/N-methylmesoporphyrin IX and p-Nitrophenol, Anal. Sci., 2021, 37(11), 1487–1489 CrossRef CAS PubMed
.
- C. Tang, Z. S. Qian and Y. Y. Huang,
et al., A fluorometric assay for alkaline phosphatase activity based on β-cyclodextrin-modified carbon quantum dots through host-guest recognition, Biosens. Bioelectron., 2016, 83, 274–280 CrossRef CAS PubMed
.
- J. S. Lei, J. Kang and J. F. Liu,
et al., A Novel Electrochemical Sensing Strategy Based on Poly (3,4-ethylenedioxythiophene): Polystyrene Sulfonate, AuNPs, and Ag+ for Highly Sensitive Detection of Alkaline Phosphatase, Nanomaterials, 2022, 12(19), 3392 CrossRef CAS PubMed
.
- X. L. Xie, Y. F. Wang and X. B. Zhou,
et al., Fe-N-C single-atom nanozymes with peroxidase-like activity for the detection of alkaline phosphatase, Analyst, 2021, 146(3), 896–903 RSC
.
|
This journal is © The Royal Society of Chemistry 2025 |
Click here to see how this site uses Cookies. View our privacy policy here.