DOI:
10.1039/D3DT04379G
(Paper)
Dalton Trans., 2025,
54, 1884-1895
Structural transformation from a 3D hydrophilic pillared-layered framework to 2D hydrophobic MOF nanosheets through ligand replacement exfoliation†
Received
29th December 2023
, Accepted 19th November 2024
First published on 5th December 2024
Abstract
A pillared-layered metal–organic framework (MOF) {[Zn2(atpt)2(bpBTD)]·solv}n (1), where H2atpt = 2-aminoterephthalic acid and bpBTD = bis(pyridin-4-yl)benzothiadiazole, showing a pcu net topology with double interpenetration was successfully constructed. Thermally activated 1 possessed free voids of 15.3% and repeatedly exhibited two-step CO2 adsorption isotherms over three runs, with uptakes of 138.8 cm3 g−1 STP at 195 K and P/P0 = 1. Through a ligand replacement exfoliation approach, metal–organic framework (MOF) nanosheets {[Zn2(atpt)2(4-picoline)2]}n (1-ns) with a 2D sql net topology were obtained by immersing 1 in 4-picoline. Characterization of 1-ns was achieved using energy-dispersive X-ray spectroscopy (EDS), infrared (IR) spectroscopy, X-ray powder diffraction (XRPD) patterns, and elemental analyses (EA) as well as scanning electron microscopy (SEM), atomic force microscopy (AFM), and X-ray photoelectron spectroscopy (XPS). Photoluminescence spectra showed that 1 and 1-ns both emitted blue-light fluorescence in H2O. In addition, measurements of water contact angles indicated that 1 is highly hydrophilic, while 1-ns shows poor hydrophilicity compared with 1. The double-sided hydrophobic surfaces of 1-ns allowed oil (dichloromethane) but not water to pass through, making it a good candidate for oil/water separation. Electrochemical measurement revealed that the 2D metal–organic nanosheet 1-ns modified glassy carbon electrode (1-ns/GCE) possessed much better electron transfer ability than the 3D pillared-layer framework 1 modified glassy carbon electrode (1/GCE), and thus improves the electrochemical performance and sensitivity.
Introduction
The coordination of metal ions or clusters with polytopic bridging organic linkers forms a class of crystalline micro–mesoporous hybrid extended nanostructures termed metal–organic frameworks (MOFs), which not only exhibit framework diversity but also show great potential in a wide range of applications involving gas adsorption and separation, catalysis, sensing, drug delivery etc.1–4 Recently, MOF nanosheets, a class of two-dimensional (2D) layered materials with nanoscale thin film thicknesses, have received much attention.5–9 Compared with their bulk counterparts, i.e., three-dimensional (3D) nanostructures, MOF nanosheets offer unique advantages, such as large surface area, highly accessible active sites, high binding site density, excellent mechanical strength, and good optical transparency,5–8 as with other traditional inorganic layered nanomaterials, such as graphene,10,11 layered double hydroxides (LDHs),12,13 transition metal dichalcogenides (TMDs),14 graphitic carbon nitride (g-C3N4),15 and hexagonal boron nitride (h-BN).16 These characteristics lead to a wide range of applications, such as separation,17–20 sensing,21–23 catalysis,24–26 electronics,27,28 and energy conversion.29,30
There are many synthetic strategies to construct MOF nanosheets, which can be classified into two main types: top-down delamination and bottom-up synthesis.6,8,9 One of the top-down strategies is ligand replacement exfoliation, derived from ligand exchange for the construction of isoreticular nets31,32 and developed by Zhong, Lu, Zhou, and co-workers.33 This is a facile, mild, and efficient method using monodentate ligands with strong coordination ability to replace the bidentate ligands with weaker coordination ability, resulting in dimensionality reduction and structural transformation from 3D MOFs to 2D MOF nanosheets and further adjustment of the surface features.
In this work, a 3D pillared-layered MOF, {[Zn2(atpt)2(bpBTD)]·solv}n (1), where H2atpt = 2-aminoterephthalic acid and bpBTD = bis(pyridin-4-yl)benzothiadiazole, showing a pcu net topology with double interpenetration, was constructed via a dual-ligand synthetic approach by mixing anionic dicarboxylate and neutral bispyridyl ligands. Furthermore, this 3D MOF can be converted to 2D MOF nanosheets, {[Zn2(atpt)2(4-picoline)2]}n (1-ns), with a 2D sql net topology by utilizing ligand replacement exfoliation. The proposed synthetic approach to pillared-layered MOFs and MOF nanosheets is shown in Scheme 1, and the molecular structures of the organic ligands are shown in Scheme 2. Characterization of the 3D pillared-layered MOF and 2D MOF nanosheets was achieved using various spectroscopic techniques. This work demonstrates that ligand replacement exfoliation is a powerful method to construct MOF nanosheets that might be difficult to fabricate through direct synthesis from raw reagents. Moreover, MOF nanosheets 1-ns exhibit hydrophobic properties to potentially separate oil from water, as well as high electrochemical performance to detect catechol.
 |
| Scheme 1 Proposed synthetic approach to pillared-layered MOFs and MOF nanosheets. | |
 |
| Scheme 2 Molecular structures of organic ligands used in this work. | |
Experimental
Materials and instruments
All chemicals were analytical-grade reagents and commercially purchased for use as received. The ligand bis(pyridin-4-yl)benzothiadiazole (bpBTD) was synthesized according to previous reports.34,351H NMR spectra were recorded on a Bruker AMX-300 Solution-NMR spectrometer. X-ray powder diffraction (XRPD) patterns were recorded using a Shimadzu XRD-7000 X-ray diffractometer with Cu Kα radiation (λ = 1.5406 Å) working at 40 kV and 30 mA. Thermogravimetric (TG) analyses were carried out using a Thermo Cahn VersaTherm HS TG analyzer. Fourier transform infrared spectra (FT-IR) were collected on a PerkinElmer Frontier Fourier transform infrared spectrometer by using the ATR (attenuated total reflectance) technique. Fluorescence spectra were monitored using a Hitachi F2700 fluorescence spectrophotometer. Microanalyses of carbon, nitrogen, and hydrogen were conducted on an Elementar Vario EL III analytical instrument. Carbon dioxide adsorption–desorption isotherms were recorded using a Micromeritics TriStar II Plus analyzer at 195 K, and the surface area values were calculated using the Brunauer–Emmett–Teller (BET) method. Water contact angles were measured using a Kruss Easy Drop goniometer. Energy dispersive X-ray spectroscopy (EDS) analyses were performed using an Oxford instruments EDS microanalysis X-MAX 80 equipped with a semiquantitative analysis program. The morphology and microstructure of the samples were examined by field-emission scanning electron microscopy (FESEM) using a JEOL JSM-7800F Prime Schottky Field Emission Scanning Electron Microscope and atomic force microscopy (AFM) using a Bruker Dimension Icon. Particle size distributions were measured on a Scientech NanoPlus instrument. Contact angles were measured using a Kruss Easy Drop goniometer, equipped with a CCD video camera. Electrochemical measurements were carried out using a CHI 6116E electrochemical ap workstation with a three-electrode system. A modified glassy carbon electrode (GCE, 3 mm in diameter) was used a working electrode. A platinum wire was used as a counter electrode. The Ag/AgCl electrode with saturated KCl was used as a reference electrode.
Synthesis of {[Zn2(atpt)2(bpBTD)]·solv}n (1)
A Teflon-lined autoclave was sequentially sealed with an aqueous solution (1 mL) of Zn(NO3)2·6H2O (0.050 mmol), an N,N-dimethylformamide (DMF) solution (2 mL) of 2-aminoterephthalic acid (H2atpt, 0.050 mmol), and a DMF solution (2 mL) of bpBTD (0.025 mmol). Then the autoclave was heated to 100 °C from room temperature over a period of 6 h, held at that temperature for 36 h, and cooled to 30 °C over a period of 48 h. Orange crystals were collected by filtration and washed with DMF. Yield: 60% based on bpBTD (12.9 mg, 0.015 mmol). IR (ATR, cm−1): 3453, 3352, 1657, 1614, 1579, 1492, 1430, 1375, 1253, 1073, 1033, 962, 892, 827, 769, 731, 661, 587, 555. Prior to elemental analysis, 1 was thermally treated under vacuum for 1 day. Anal. calcd for C32H20N6O8SZn2·C3H7NO: C, 49.31; H, 3.19; N, 11.50%. Found: C, 48.86; H, 2.83; N, 10.95%.
Preparation of {[Zn2(atpt)2(4-picoline)2]}n (1-ns)
A crystalline sample of 1 (200 mg, 0.235 mmol) was immersed in 4-picoline (20 mL, 0.206 mol) in a 25 mL scintillation vial. The resulting suspension was stirred at room temperature on a magnetic stirrer for 7 days. The crude solids were collected by filtration and washed with deionized water several times. The naturally dried solid was immersed in dichloromethane to remove impurities. Then, the brownish MOF nanosheets, {[Zn2(atpt)2(4-picoline)2]}n (1-ns), were collected by filtration and naturally dried. Yield: 78% (107 mg, 0.184 mmol). IR (ATR, cm−1): 3452, 3339, 3072, 1608, 1571, 1506, 1439, 1366, 1256, 1142, 1070, 1032, 959, 891, 808, 768, 720, 589, 551. Elemental analysis for the naturally dried MOF nanosheets: C, 50.20; H, 3.56; N, 8.25 S, 0.00%. Anal. calcd required for C28H24N4O8Zn2: C, 49.80; H, 3.58; N, 8.30; S, 0.00%.
Single-crystal X-ray structure determinations
A yellow prism-shaped crystal was selected and mounted on a Bruker D8 Venture Photon III diffractometer. The incident light for the X-ray was Cu Kα radiation (λ = 1.54178 Å). The crystal was kept at a steady T = 100(2) K during data collection. The structure was solved with the SHELXS-97 program using direct methods.36 The model was refined with SHELXL-2014/7,37 incorporated in WINGX-v2021.3,38 using full matrix least squares minimisation on F2. Most non-hydrogen atoms were refined anisotropically except some disordered atoms that were refined isotropically. Hydrogen atom positions were calculated geometrically and refined using the riding model. Experimental details for X-ray data collection and the refinements are summarized in Table 1. CCDC 2322407 (1)† contains the supplementary crystallographic data for this paper.
Table 1 Crystallographic data for 1
|
1
|
R
1 = ∑||Fo| − |Fc||/∑|Fo|.
wR2 = {∑[w(Fo2 − Fc2)2]/∑[w(Fo2)2]}1/2.
|
Empirical formula |
C32H20N6O8SZn2 |
M
w
|
779.34 |
Crystal system |
Monoclinic |
Space group |
C2/m |
a (Å) |
14.7876(9) |
b (Å) |
16.0688(9) |
c (Å) |
18.3696(12) |
β (°) |
90.131(3) |
V (Å3) |
4365.0(5) |
Z
|
4 |
T (K) |
100(2) |
λ (Å) |
1.54178 |
D
calc (g cm−3) |
1.186 |
F
000
|
1576 |
μ (mm−1) |
2.191 |
GOF on F2 |
1.110 |
R
1 a, wR2 b (I > 2σ (I)) |
0.0803, 0.2571 |
R
1 a, wR2 b (all data) |
0.0883, 0.2676 |
Electrochemical measurements
To evaluate the electrochemical properties of 1 and 1-ns, both were applied to modify the glassy carbon electrode (GCE). Then, cyclic voltammetry (CV) and electrochemical impedance spectroscopy (EIS) experiments were carried out in a 5.0 mM [Fe(CN)6]3−/4− and 0.1 M KCl solution. For EIS measurements, the experiments were performed with an equilibrium potential of the open current potential. The frequency was in the range 100 kHz to 0.01 Hz.
For electrode-modification, alumina powder (0.05 μm) was used to polish the bare GCE, which was then ultrasonically cleaned using distilled deionized water. Onto the mirror-like GCE surface, an as-prepared ethanol suspension of 1 (10 μL, 2 mg mL−1) was dropped, and it was then dried under infrared irradiation for 10 min at room temperature. The so-obtained modified electrode is abbreviated to 1/GCE. Similarly, 1-ns and H2atpt modified electrodes, termed as 1-ns/GCE and H2atpt/GCE, were also prepared.
Results and discussion
Synthesis and structures
The pillared-layered MOF {[Zn2(atpt)2(bpBTD)]·solv}n (1, H2atpt = 2-aminoterephthalic acid and bpBTD = bis(pyridin-4-yl)benzothiadiazole was hydro(solvo)thermally prepared from the reaction of Zn(NO3)2·6H2O, H2atpt, and bpBTD in a 2
:
2
:
1 ratio in an N,N-dimethylformamide (DMF)/H2O solution. Single-crystal X-ray diffraction analysis showed that 1 crystallized in the monoclinic space group C2/m. The 3D pillared-layered framework of 1 is built from [Zn2(atpt)2]n 2D grid-like layers with typical [Zn2(COO)4] paddlewheel secondary building units (SBUs) and N-tethering bpBTD pillars (Fig. 1a–c and S1†), and adopts a 6-connected primitive cubic (pcu) lattice topology with the point symbol of 41263 (Fig. 1d–e and S2†). Two identical pcu nets are mutually interpenetrated with each other to yield doubly interpenetrating 3D frameworks with 3D intersection open voids along the [001], [
10], and [1
0] directions, having sufficient extra-framework void spaces of approximately 15.3% (Fig. 1f and S3†), as calculated by the PLATON/SOLV routine.39
 |
| Fig. 1 Crystal structure of 1: (a) the dinuclear [Zn2(COO)4] paddlewheel SBU; (b) schematic representation of the 6-connected node; (c) a single [Zn2(atpt)2]n 2D grid-like layer; (d) a single pillared-layer framework; (e) schematic representation of the pcu framework topology; (f) ball-and-stick and schematic representations of the pillared-layer pcu framework with 2-fold interpenetration. The disordered parts are omitted for clarity. | |
Phase purity, stability, and CO2 adsorption properties
X-ray powder diffraction (XRPD) measurements indicated that the bulk sample of 1 gave satisfactory experimental patterns, matching those of the simulated ones from the single-crystal data (Fig. 2), confirming the pure single phase of the bulk materials. Furthermore, the stability of 1 in H2O and common organic solvents, such as MeOH and DMF, was also checked. After immersing 1 in the above-mentioned solvents for 1 day, the recovered solid samples demonstrated high crystallinity, supported by their qualified XRPD patterns (Fig. 2). In addition, the almost identical XRPD patterns to those of simulated and as-synthesized 1 unambiguously prove that 1 exhibits high solvent stability.
 |
| Fig. 2 Simulated and experimental XRPD patterns of 1 before and after immersing in H2O, MeOH, and DMF as well as after activation and gas adsorption/desorption. | |
The pertinent porosity of 1 was examined by gas adsorption. Prior to the sorption measurements, the as-synthesized samples of 1 were exchanged with methanol and subsequently degassed at 373 K under high vacuum for 24 h. Low-pressure physisorption experiments for N2 and CO2 were conducted and recorded volumetrically at 77 K and 195 K, respectively (Fig. 3). Observations showed that activated 1 exhibited type I isotherms with some hysteresis. The N2 adsorption isotherm showed a rapid increased at a very low pressure up to P/P0 ≈ 0.0013, where the uptake was about 24.5 cm3 g−1 (1.09 mmol g−1). Subsequently, the adsorbed amounts were gradually increased and then saturated at P/P0 = 1, with an uptake of about 41.2 cm3 g−1 (1.83 mmol g−1). Accordingly, the Brunauer–Emmett–Teller (BET) surface area of activated 1 is estimated as 96.6 m2 g−1 (the Langmuir surface area is 155.0 m2 g−1). Conversely, activated 1 exhibited a rapid increase in the CO2 adsorption isotherm at pressures up to P/P0 ≈ 0.043 with an uptake of about 40.8 cm3 g−1 (1.82 mmol g−1). Then the CO2 adsorption uptakes were gradually increased with gradually increasing pressure up to P/P0 approaching 0.57 and subsequently remained almost constant. The maximum uptake of CO2 was about 138.8 cm3 g−1 (6.20 mmol g−1) at P/P0 = 1. As a result, thermally activated 1 possessed a two-step adsorption isotherm for CO2. Based on the CO2 uptakes, the BET surface area of activated 1 is estimated as 126.0 m2 g−1 (the Langmuir surface area is 636.9 m2 g−1). The desorption profile revealed a strong hysteresis loop and drastic desorption at P/P0 ≈ 0.053, suggesting strong CO2–framework interactions. Notably, the CO2 adsorption–desorption isotherms were repeated over three runs without significant changes (Fig. S4†).
 |
| Fig. 3 Gas adsorption isotherms of activated 1 at 77 K for N2 and 195 K for CO2. | |
XRPD patterns of 1 recovered from gas adsorption exhibited broadened patterns (Fig. 2), which could be attributed to activation- and adsorption-induced framework loss. However, the peak patterns were almost identical to that of as-synthesized 1, suggesting high framework stability during gas adsorption.
Preparation and characterization of metal–organic framework nanosheets (1-ns)
Through the ligand replacement exfoliation approach, metal–organic framework (MOF) nanosheets {[Zn2(atpt)2(4-picoline)4]}n (1-ns) were obtained by immersing 1 in 4-picoline (Scheme 1), wherein the bpBTD bridges were replaced by 4-picoline as capping ligands. Energy-dispersive X-ray spectroscopy (EDS) showed the existence of very low contents of S in 1-ns, with average S%
:
Zn% = 1.85%
:
98.15% (Fig. S5†), suggesting the success of the ligand replacement exfoliation. The infrared (IR) spectrum of 1-ns clearly showed the appearance of the methyl C–H stretching vibration at around 3072 cm−1 and large changes in the 1700–1550, 1500–1300, and 900–800 cm−1 regions, ascribed to carboxyl C
O/C–O, aromatic C
N/C–N and C
C/C–C, and N–S vibrations (Fig. S6†), compared with those of 1. This observation indicates the existence of 4-picoline within the metal–organic nanosheets 1-ns. In addition, when ligand replacement exfoliation was achieved, the clean solution was recovered for 1H NMR measurement, which showed only one set of signals in the aromatic region belonging to the free bpBTD ligand (Fig. S7†). Conversely, when 1-ns was decomposed by aqueous HCl solution in D2O, the so-obtained 1H NMR spectrum clearly showed two sets of signals from atpt2− and 4-picoline with a stoichiometry of 1
:
1 (Fig. S8†). These spectral observations provide evidence for the successful replacement of the ligand to form metal–organic nanosheets.
XRPD patterns of 1-ns, collected from the supernatant by natural evaporation revealed significant diffraction peaks (Fig. 4), implying that 1-ns possesses some crystallinity. Three strong diffraction peaks appeared at 9.14, 16.44, and 24.72°, which can be related to the peaks at 8.10, 16.18, and 24.38°, respectively, in the simulated XRPD patterns of 1, corresponding to the lattice planes with Miller indices of (110), (220), and (330), respectively. These lattice planes are perpendicular to the [Zn2(atpt)2]n 2D grid-like layers in 1 (Fig. S9†). Moreover, the three strong and sharp diffraction peaks unambiguously indicate that the [Zn2(atpt)2]n 2D grid-like layers are ordered and stacked very well in 1-ns to give some crystallinity. In comparison, the lattice plane with Miller indices of (002) appearing at 9.60° in the simulated XRPD patterns of 1 is perpendicular to the bpBTD pillars (Fig. S9†), and the intensity is greatly reduced and shifted to 9.96° in 1-ns. Notably the 2-theta values in 1-ns are slightly higher than that in 1, indicating the reduction of plane distances after ligand replacement. However, the high similarity between the diffraction peaks of 1-ns and 1 indicates that the ligand replacement exfoliation processes did not cause drastic packing change and thus the metal–organic nanosheets 1-ns might possess [Zn2(atpt)2]n based grid-like layer structures.33,40
 |
| Fig. 4 Simulated and experimental XRPD patterns of 1 and experimental XRPD patterns of 1-ns. | |
To further check the stability of the nanosheets, as-synthesized 1-ns was collected and immersed in water for 1 day. The recovered solids showed XRPD peaks with a pattern similar to that of as-synthesized 1-ns, and became sharper (Fig. 4), implying that the [Zn2(atpt)2]n 2D grid-like layers are re-orientated. In addition, the representative high crystallinity indicates that nanosheets 1-ns possess high water stability.
Elemental microanalysis data were found to fit the formula {[Zn2(atpt)2(4-picoline)2]}n very well (see the Experimental section), which is in good agreement with the stoichiometry of atpt2− and 4-picoline proposed from the 1H NMR spectrum. As a result, it is reasonable to assume that metal–organic nanosheets 1-ns had a 2D sql layer structure, where the typical [Zn2(COO)4] paddlewheel SBUs were connected by atpt2− ligands to form the 2D grid-like layer of [Zn2(atpt)2]n and the apical position of each Zn center in the [Zn2(COO)4] SBU was ligated by a monodentate 4-picoline ligand. An attempt was made to obtain MOF nanosheets 1-ns through a direct synthesis approach from the solvothermal reactions of Zn2+, H2atpt, and 4-picoline, but unfortunately, it was unsuccessful.
Thermal stability and microstructural analysis
The thermogravimetric (TG) curve of 1 showed a weight loss of 9.4% upon heating from room temperature to approximately 176 °C (Fig. 5), which can be ascribed to the removal of lattice H2O and DMF molecules (calcd 10.5% based on [Zn2(atpt)2(bpBTD)]·DMF·H2O). Then the solvent-free framework was thermally stable until it began to decompose at 344 °C, which continued until 554 °C. The final residues had a weight percentage of 19.1%, reasonably assigned to ZnO components (calcd 18.8%). The TG curve of 1-ns showed poorer thermal stability than that of the mother pillared-layer MOF 1, with a gradual decrease in the TG trace for the weight percentage (Fig. 5). The decomposition of 1-ns ended at 495 °C, leaving ZnO component final residues (found 23.1%, calcd 24.1%).
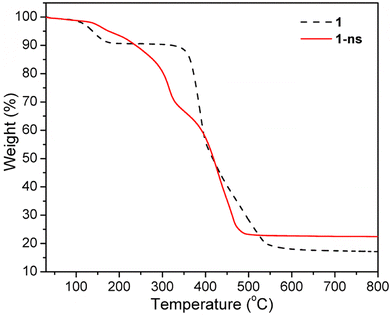 |
| Fig. 5 TG curves of 1 and 1-ns. | |
Scanning electron microscopy (SEM) was utilized to analyze the morphologies of 1 and 1-ns with a concentration of 1 mg per 4 mL H2O. It was observed that 1 exhibited well-shaped rod-like crystals with smooth surfaces and sizes of around 100 μm × 450 μm (Fig. 6a). Comparably, 1-ns exhibited plate-like crystallites with sizes of around 0.4 μm × 0.4 μm × 0.2 μm (Fig. 6b and S10†). Furthermore, SEM images revealed the regular aggregation of the plate-like crystallites. The drastic change in crystal morphology undoubtedly supported the success of the ligand replacement exfoliation strategy to synthesise MOF nanosheets from the pillared-layered framework.24,33,41
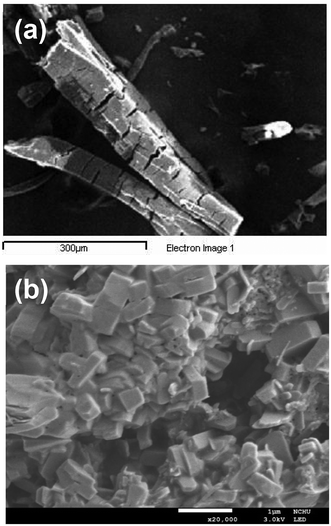 |
| Fig. 6 SEM images of (a) 1 and (b) 1-ns. | |
Microstructural analysis of 1-ns was further carried out by atomic force microscopy (AFM) with a concentration of 1 mg per 8 mL H2O. The AFM image clearly indicated that the nanosheets were well-dispersed at such a concentration. The thickness of the nanosheets is nearly uniform, with a value of approximately 3.5 nm (Fig. 7 and S11†), corresponding to the stacking of two layers of nanosheets (the thickness of a monolayer of nanosheets is estimated to be about 1.59 nm from the crystal structure analysis).
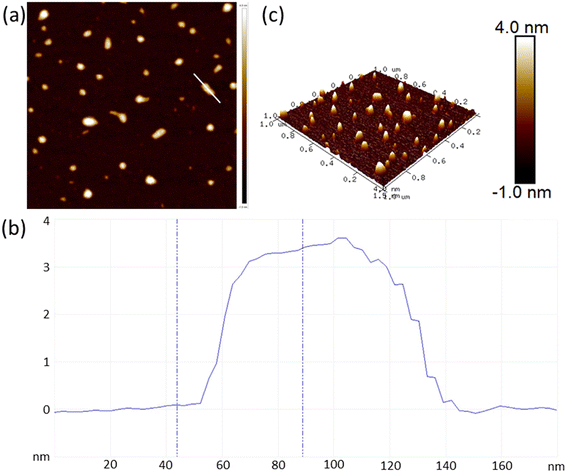 |
| Fig. 7 (a) AFM image of 1-ns showing the surface topography. (b) Height profiles of 1-ns. (c) 3D AFM image of 1-ns. | |
Furthermore, particle size distributions of 1 and 1-ns at a concentration of 1 mg per 8 mL H2O showed that the former has a peak of particle size distribution at a diameter of 1031.0 ± 222.3 nm, while the latter possesses a particle size distribution peak at a diameter of 2.2 ± 0.6 nm (Fig. S12†). It is shown that the particle size of 1-ns is greatly reduced compared with that of 1, implying the success of the structural transformation from a 3D hydrophilic pillared-layered framework to 2D hydrophobic MOF nanosheets through ligand replacement exfoliation.
Wettability
The wettabilities of 1 and 1-ns were assessed using water contact angles. The crystalline samples of 1 and 1-ns were both ground and pressured to give thin-films for contact angle tests. Measurement indicated that 1 displayed highly hydrophilic features, as supported by the non-detectable water contact angle (Fig. 8a and ESI SI_vedio_1†). In comparison, 1-ns possessed a water contact angle of 61.5° (Fig. 8b), and exhibited less hydrophilic characteristics than 1. This is ascribed to the introduction of the hydrophobic 4-picoline as a terminal capping ligand.
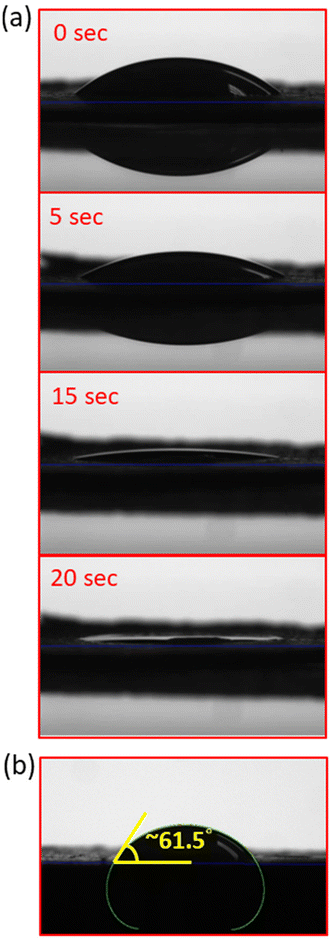 |
| Fig. 8 Water contact angle on (a) 1 and (b) 1-ns thin-film surfaces. | |
Fluorescence properties
Many Zn-based pillared-layered frameworks exhibit fascinating fluorescence properties and are utilized in sensing applications for a wide range of analytes.35,42–46 Therefore, it is interesting to understand the photophysical properties of 1 and 1-ns. Excitation and photoluminescence spectra of bpBTD, H2atpt, 1, and 1-ns were measured in the solid-state and in H2O at room temperature (Fig. 9 and S13†). Solid-state fluorescence properties of 1 and 1-ns showed that pillared-layered framework 1 exhibited a very weak peak at 437 nm upon excitation at 365 nm, whereas MOF nanosheets 1-ns displayed a weak broad peak centered at 452 nm upon excitation at 370 nm (inset in Fig. 9). The emissions were compared to those at 468 nm for bpBTD and 533 nm for H2atpt upon excitation at 365 nm. Although the emissions of 1 and 1-ns seem to be closer to the bpBTD-based intraligand transition, when taking their molecular composites into account, it is reasonable to assume that H2atpt-based intraligand transition is dominant in the fluorescence of 1 and 1-ns modulated by deprotonation and coordination. Fluorescence responses of 1 and 1-ns dispersed in H2O supported this assumption very well. Pillared-layered framework 1 dispersed in H2O displayed a moderate structureless emission centered at 428 nm when excited at 330 nm, while metal–organic nanosheets 1-ns also exhibited a structureless emission with remarkable intensity enhancement centered at 428 nm when excited at 340 nm (Fig. 9). Comparably, H2atpt in H2O revealed a moderate emission centered at 456 nm when excited at 315 nm whereas bpBTD in H2O revealed a relatively weak emission centered at 450 nm when excited at 320 nm. It is clear that the emission of bpBTD is insensitive to its phase, but the emission of H2atpt is sensitive to its phase due to the occurrence of interactions between the H2atpt and the water molecules.47–49 Therefore, the similarity between the two emissions of 1 and 1-ns imply that both of them originated from the ligand-centered emission arising from atpt2−. The blue-shift of close to 30 nm is attributed to coordination. However, for 1, the contribution of bpBTD to the emission cannot be ruled out completely. With the good fluorescence properties in water suspensions, 1 and 1-ns show good potential for sensing applications.
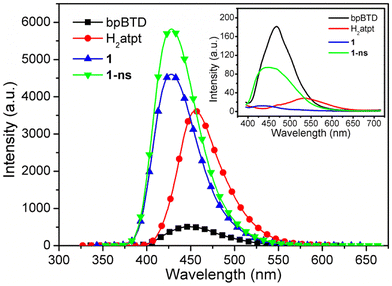 |
| Fig. 9 Photoluminescence spectra of bpBTD, H2atpt, 1, and 1-ns in H2O. Inset: photoluminescence spectra of bpBTD, H2atpt, 1, and 1-ns in the solid-state. Slits are 5.0 nm × 5.0 nm. | |
Electrochemical properties
Pillared-layered MOF 1 and MOF nanosheets 1-ns were both used for electrode modification materials to modify the glassy carbon electrode (GCE). The electrochemical properties of as-fabricated electrodes, 1/GCE and 1-ns/GCE, were explored by cyclic voltammetry (CV) in a 5.0 mM ferricyanide ([Fe(CN)6]3−/4−) and 0.1 M KCl solution. It was observed that bare GCE clearly displayed a reversible redox couple of Fe(III)/Fe(II), with an anionic (oxidation) peak potential (Epa) at 0.299 V and a cathodic (reduction) peak potential (Epc) at 0.159 V (Fig. 10a). Comparably, 1/GCE and 1-ns/GCE both showed no redox peaks, implying that 1 and 1-ns were both non-conductive materials. Electrochemical impedance spectroscopy (EIS) was also investigated to further explore the electrochemical properties of the modified GCEs, and experiments were conducted in a 10.0 mM ferricyanide and 0.1 M KCl solution. The Nyquist plots revealed that bare GCE gave the smallest semicircle with a radius of about 1256 ohms whereas 1/GCE and 1-ns/GCE showed large semicircles with radii of about 7721 and 3513 ohms, respectively, as shown in Fig. 10b. It is well known that a large Nyquist semicircle indicates a large electrical resistance (electroresistance) and thus low electrical conductivity.50,51 Hence, 1-ns/GCE possessed better electrical conductivity than 1/GCE.
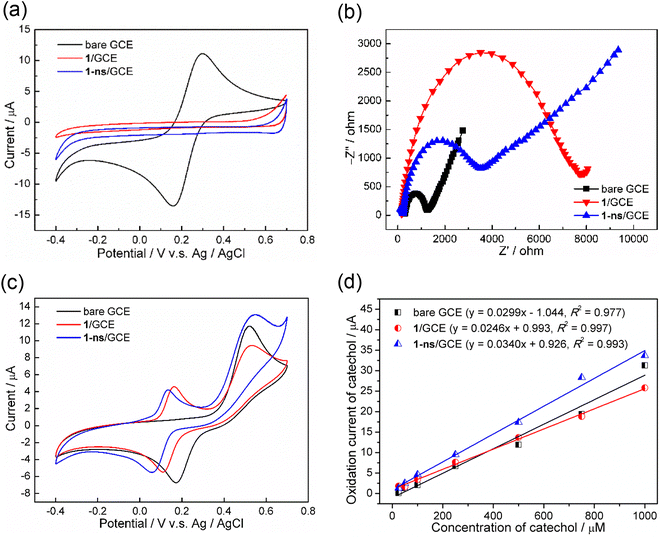 |
| Fig. 10 (a) CV diagrams and (b) Nyquist plots obtained for bare GCE, 1/GCE, and 1-ns/GCE in ferricyanide in 0.1 M KCl solution used as working electrodes at 100 mV s−1. (c) CV diagrams obtained for 1.0 mM catechol at bare GCE, 1/GCE, and 1-ns/GCE in 0.1 M PBS buffer solution (pH 7.0) at 100 mV s−1 scan rate. (d) Linear fitting of the oxidation peak currents of catechol with the concentration of catechol in the range 25–1000 μM. | |
With all the above structural and electrochemical information, we proceeded to further study the electrochemical detection of catechol (CC) using 1 and 1-ns modified GCEs, i.e., 1/GCE and 1-ns/GCE as work electrodes. As-prepared 1/GCE or 1-ns/GCE was immersed into a 0.1 M phosphate-buffered saline (PBS) buffer solution (pH 7.0) containing CC with a concentration of 1.0 mM. The electrochemical detection of CC on the modified GCE surface was performed using CV in a potential window of −0.4 to 0.7 V, with respect to Ag/Ag+, with a scan rate of 100 mV s−1. As shown in Fig. 10c, the CV of CC at the bare GCE showed an anionic peak at 0.521 V, with an anionic current of 10.4 μA, attributed to the oxidation of CC. Comparably, the CV of CC at 1/GCE and 1-ns/GCE showed that the oxidation of CC was at 0.533 and 0.549 V, respectively, with an anionic current of 7.7 and 11.1 μA, respectively. Furthermore, there was also a pair of reversible redox signals, with an anionic peak at 0.163 V and a cathodic peak at 0.110 V for 1 and an anionic peak at 0.134 V and a cathodic peak at 0.057 V for 1-ns. This reversible redox signal was mostly ascribed to the oxidation/reduction of atpt2− instead of bpBTD, as supported by the similar electrochemical response of H2atpt-modified GCE in detecting CC (Fig. S14†). Moreover, the anodic peak of such a reversible redox couple did not occur in the first CV cycle when using 1/GCE and 1-ns/GCE as working electrodes to detect CC (Fig. S15†); this implies that the redox couple was not due to the oxidation of CC but the modified material itself.
To examine the performances of modified GCEs in electrochemically detecting CC, quantitative measurements were performed by using as-prepared modified GCEs as work electrodes in pH = 7.0 PBS buffer containing CC with various concentrations from 25 μM to 1000 μM at a scan rate of 100 mV s−1 (Fig. S16†). Experimental results showed that the oxidation peak currents of CC linearly increased with the increase of the CC concentration for all tested electrodes (Fig. 10d), giving the current–concentration relationships of Iox(CC) = 0.0299 × [CC] − 1.044 (R2 = 0.977) for bare GCE, Iox(CC) = 0.0246 × [CC] + 0.993 (R2 = 0.997) for 1/GCE, and Iox(CC) = 0.0340 × [CC] + 0.926 (R2 = 0.993) for 1-ns/GCE. The slopes of the three straight lines followed the order of 1-ns/GCE (0.0340 μA μM−1) > bare GCE (0.0299 μA μM−1) > 1/GCE (0.0246 μA μM−1), which reflects the order of electrocatalytic sensitivity of the electrode toward CC. As a result, 1-ns/GCE exhibited better electrochemical performance than 1/GCE in detecting CC, indicating that the 2D metal–organic nanosheet 1-ns possesses much better electron transfer ability than the 3D pillared-layer framework 1 and thus enhances the electrochemical sensitivity.
Conclusion
A 3D hydrophilic pillared-layered framework {[Zn2(atpt)2(bpBTD)]·0.75DMF·0.75H2O}n (1) with a pcu net topology has been well prepared. The pillared-layered framework exhibited a two-step adsorption isotherm for CO2 with a maximum uptake of about 138.8 cm3 g−1 (6.20 mmol g−1) at T = 195 K and P/P0 = 1, with high framework stability during CO2 adsorption and desorption. Through ligand replacement exfoliation, pillared-layered framework 1 has been successfully converted to 2D hydrophobic MOF nanosheets {[Zn2(atpt)2(4-picoline)2]}n (1-ns) with a 2D sql net topology. The composition and structural properties have been clearly characterized by numerous spectroscopic techniques. Furthermore, MOF nanosheets 1-ns exhibited high potential for oil separation due to the hydrophobic features and also for improvement of electrochemical sensitivity compared to the pillared-layered framework 1.
Data availability
Crystallographic data for 1 has been deposited at the CCDC under 2322407.†
Conflicts of interest
There are no conflicts to declare.
Acknowledgements
We gratefully acknowledge the National Chi Nan University and the National Science and Technology Council (Grant No. MOST 108-2113-M-260-002-, MOST 109-2113-M-260-007-, MOST 110-2113-M-260-006-, and MOST 111-2113-M-260-004-) for the financial support. We thank Dr Jian-Hong Liao (X-ray facility scientist, Institute of Chemistry, Academia Sinica) for data collection on single-crystal X-ray diffraction. We also thank the NSC for providing valuable assistance on research support (MOST 110-2731-M-260-001-, NMR004800, MS004800, XRD003300, TA000400; MOST 110-2731-M-005-001-, XRD001300, EA000100).
References
- H.-C. Zhou, J. R. Long and O. M. Yaghi, Introduction to Metal–Organic Frameworks, Chem. Rev., 2012, 112, 673–674 CrossRef CAS.
- T. Rasheed and F. Nabeel, Luminescent metal–organic frameworks as potential sensory materials for various environmental toxic agents, Coord. Chem. Rev., 2019, 401, 213065 CrossRef CAS.
- H.-Y. Li, S.-N. Zhao, S.-Q. Zang and J. Li, Functional metal–organic frameworks as effective sensors of gases and volatile compounds, Chem. Soc. Rev., 2020, 49, 6364–6401 RSC.
- L. Zhu, X.-Q. Liu, H.-L. Jiang and L.-B. Sun, Metal−Organic Frameworks for Heterogeneous Basic Catalysis, Chem. Rev., 2017, 117, 8129–8176 CrossRef CAS PubMed.
- R. Sakamoto, K. Takada, X. Sun, T. Pal, T. Tsukamoto, E. J. H. Phua, A. Rapakousiou, K. Hoshiko and H. Nishihara, The coordination nanosheet (CONASH), Coord. Chem. Rev., 2016, 320–321, 118–128 CrossRef CAS.
- Y.-z. Li, Z.-h. Fu and G. Xu, Metal–organic framework nanosheets: Preparation and applications, Coord. Chem. Rev., 2019, 388, 79–106 CrossRef CAS.
- J. Nicks, K. Sasitharan, R. R. R. Prasad, D. J. Ashworth and J. A. Foster, Metal–Organic Framework Nanosheets: Programmable 2D Materials for Catalysis, Sensing, Electronics, and Separation Applications, Adv. Funct. Mater., 2021, 31, 2103723 CrossRef CAS.
- M. Zhao, Y. Huang, Y. Peng, Z. Huang, Q. Ma and H. Zhang, Two-dimensional metal–organic framework nanosheets: synthesis and applications, Chem. Soc. Rev., 2018, 47, 6267–6295 RSC.
- R. Makiura, Creation of metal–organic framework nanosheets by the Langmuir-Blodgett technique, Coord. Chem. Rev., 2022, 469, 214650 CrossRef CAS.
- M. Yi and Z. Shen, A review on mechanical exfoliation for the scalable production of graphene, J. Mater. Chem. A, 2015, 3, 11700–11715 RSC.
- Y. Xu, H. Cao, Y. Xue, B. Li and W. Cai, Liquid-Phase Exfoliation of Graphene: An Overview on Exfoliation Media, Techniques, and Challenges, Nanomaterials, 2018, 8, 942 CrossRef.
- H. Yin and Z. Tang, Ultrathin two-dimensional layered metal hydroxides: an emerging platform for advanced catalysis, energy conversion and storage, Chem. Soc. Rev., 2016, 45, 4873–4891 RSC.
- J. Yu, Q. Wang, D. O'Hare and L. Sun, Preparation of two dimensional layered double hydroxide nanosheets and their applications, Chem. Soc. Rev., 2017, 46, 5950–5954 RSC.
- W. Choi, N. Choudhary, G. H. Han, J. Park, D. Akinwande and Y. H. Lee, Recent development of two-dimensional transition metal dichalcogenides and their applications, Mater. Today, 2017, 20, 116–130 CrossRef CAS.
- W.-J. Ong, L.-L. Tan, Y. H. Ng, S.-T. Yong and S.-P. Chai, Graphitic Carbon Nitride (g-C3N4)-Based Photocatalysts for Artificial Photosynthesis and Environmental Remediation: Are We a Step Closer To Achieving Sustainability?, Chem. Rev., 2016, 116, 7159–7329 CrossRef CAS PubMed.
- K. Zhang, Y. Feng, F. Wang, Z. Yang and J. Wang, Two dimensional hexagonal boron nitride (2D-hBN): synthesis, properties and applications, J. Mater. Chem. C, 2017, 5, 11992–12022 RSC.
- Y. Peng, Y. Li, Y. Ban, H. Jin, W. Jiao, X. Liu and W. Yang, Metal–organic framework nanosheets as building blocks for molecular sieving membranes, Science, 2014, 346, 1356–1359 CrossRef CAS PubMed.
- P. Chandrasekhar, A. Mukhopadhyay, G. Savitha and J. N. Moorthy, Orthogonal self-assembly of a trigonal triptycene triacid: signaling of exfoliation of porous 2D metal–organic layers by fluorescence and selective CO2 capture by the hydrogen-bonded MOF, J. Mater. Chem. A, 2017, 5, 5402–5412 RSC.
- M. Liu, K. Xie, M. D. Nothling, P. A. Gurr, S. S. L. Tan, Q. Fu, P. A. Webley and G. G. Qiao, Ultrathin Metal–Organic Framework Nanosheets as a Gutter Layer for Flexible Composite Gas Separation Membranes, ACS Nano, 2018, 12, 11591–11599 CrossRef CAS PubMed.
- G.-E. Wang and G. Xu, Construction of superhydrophobic MOF membrane for ultrafast alcohol-water separation, Sci. Bull., 2022, 67, 2381–2383 CrossRef CAS.
- M. V. Varsha and and G. Nageswaran, Review–2D Layered Metal Organic Framework Nanosheets as an Emerging Platform for Electrochemical Sensing, J. Electrochem. Soc., 2020, 167, 136502 CrossRef.
- D. J. Ashworth, A. Cooper, M. Trueman, R. W. M. Al-Saedi, S. D. Liam, A. J. Meijer and J. A. Foster, Ultrasonic Exfoliation of Hydrophobic and Hydrophilic Metal–Organic Frameworks To Form Nanosheets, Chem. – Eur. J., 2018, 24, 17986–17996 CrossRef CAS PubMed.
- W.-H. Deng, Q.-H. Li, J. Chen, C.-Z. Wang, Z.-H. Fu, X.-L. Ye, G.-E. Wang and G. Xu, A Humidity-Induced Large Electronic Conductivity Change of 107 on a Metal–Organic Framework for Highly Sensitive Water Detection, Angew. Chem., Int. Ed., 2023, 62, e202305977 CrossRef PubMed.
- J. Huang, Y. Li, R. K. Huang, C. T. He, L. Gong, Q. Hu, L. Wang, Y. T. Xu, X. Y. Tian, S. Y. Liu, Z. M. Ye, F. Wang, D. D. Zhou, W. X. Zhang and J. P. Zhang, Electrochemical Exfoliation of Pillared-Layer Metal–Organic Framework to Boost the Oxygen Evolution Reaction, Angew. Chem., Int. Ed., 2018, 57, 4632–4636 CrossRef CAS PubMed.
- A. Dhakshinamoorthy, A. M. Asiri and H. Garcia, 2D Metal–Organic Frameworks as Multifunctional Materials in Heterogeneous Catalysis and Electro/Photocatalysis, Adv. Mater., 2019, 31, 1900617 CrossRef CAS PubMed.
- X. Hou, T. Jiang, X. Xu, X. Wang, J. Zhou, H. Xie, Z. Liu, L. Chu and M. Huang, Coupling of NiFe-Based Metal–Organic Framework Nanosheet Arrays with Embedded Fe-Ni3S2 Clusters as Efficient Bifunctional Electrocatalysts for Overall Water Splitting, Chin. J. Struct. Chem., 2022, 41, 2207074–2207080 CAS.
- L. Liu, X.-Y. Lu, M.-L. Zhang, Y.-X. Ren, J.-J. Wang and X.-G. Yang, 2D MOF nanosheets as an artificial light-harvesting system with enhanced photoelectric switching performance, Inorg. Chem. Front., 2022, 9, 2676–2682 RSC.
- X. Song, J. Liu, T. Zhang and L. Chen, 2D conductive metal–organic frameworks for electronics and spintronics, Sci. China:Chem., 2020, 63, 1391–1401 CrossRef CAS.
- X. Liu, P. Geng, G. Zhang, Y. Zhang, F. Dou and H. Pang, Synthesis of 2D Co-MOF Nanosheets and Low-Temperature Calcination Activation for Lithium-Ion Batteries, Inorg. Chem., 2023, 62, 6527–6536 CrossRef CAS.
- J. Wan, J. Li, Z. Xiao, D. Tang, B. Wang, Y. Xiao and W. Xu, Transition Bimetal Based MOF Nanosheets for Robust Aqueous Zn Battery, Front. Mater., 2020, 7, 194 CrossRef.
- M. Kim, J. F. Cahill, Y. Su, K. A. Prather and S. M. Cohen, Postsynthetic ligand exchange as a route to functionalization of ‘inert’ metal–organic frameworks, Chem. Sci., 2012, 3, 126–130 RSC.
- C.-C. Chiu, F.-K. Shieh and H.-H. G. Tsai, Ligand Exchange in the Synthesis of Metal–Organic Frameworks Occurs Through Acid-Catalyzed Associative Substitution, Inorg. Chem., 2019, 58, 14457–14466 CrossRef CAS PubMed.
- J.-H. Deng, Y.-Q. Wen, J. Willman, W.-J. Liu, Y.-N. Gong, D.-C. Zhong, T.-B. Lu and H.-C. Zhou, Facile Exfoliation of 3D Pillared Metal–Organic Frameworks (MOFs) to Produce MOF Nanosheets with Functionalized Surfaces, Inorg. Chem., 2019, 58, 11020–11027 CrossRef CAS PubMed.
- Q.-J. Jiang, J.-Y. Lin, Z.-J. Hu, V. K. S. Hsiao, M.-Y. Chung and J.-Y. Wu, Luminescent Zinc(II) Coordination Polymers of Bis(pyridin-4-yl)benzothiadiazole and Aromatic Polycarboxylates for Highly Selective Detection of Fe(III) and High Valent Oxyanions, Cryst. Growth Des., 2021, 21, 2056–2067 CrossRef CAS.
- P.-M. Chuang, Y.-J. Tu and J.-Y. Wu, A thiadiazole-functionalized Zn(II)-based luminescent coordination polymer with seven-fold interweaved herringbone nets showing solvent-responsive fluorescence properties and discriminative detection of ethylenediamine, Sens. Actuators, B, 2022, 366, 131967 CrossRef CAS.
- G. M. Sheldrick, A short history of SHELX, Acta Crystallogr., Sect. A:Found. Crystallogr., 2008, 64, 112–122 CrossRef CAS PubMed.
- G. M. Sheldrick, Crystal structure refinement with SHELXL, Acta Crystallogr., Sect. C:Cryst. Struct. Commun., 2015, 71, 3–8 CrossRef PubMed.
- L. J. Farrugia, WinGX and ORTEP for Windows: an update, J. Appl. Crystallogr., 2012, 45, 849–854 CrossRef CAS.
- A. L. Spek, Structure validation in chemical crystallography, Acta Crystallogr., Sect. D:Biol. Crystallogr., 2009, 65, 148–155 CrossRef CAS PubMed.
- T. Rodenas, I. Luz, G. Prieto, B. Seoane, H. Miro, A. Corma, F. Kapteijn, F. X. L. I. Xamena and J. Gascon, Metal–organic framework nanosheets in polymer composite materials for gas separation, Nat. Mater., 2015, 14, 48–55 CrossRef CAS PubMed.
- C. Zhang, Y. Huang, H. Zhao, H. Zhang, Z. Ye, P. Liu, Y. Zhang and Y. Tang, One-Pot Exfoliation and Functionalization of Zeolite Nanosheets for Protection of Paper-Based Relics, ACS Appl. Nano Mater., 2021, 4, 10645–10656 CrossRef CAS.
- C.-H. Yeh, M.-J. Tsai, P.-C. Lee and J.-Y. Wu, Zinc(II)-Based Ring-and-Rod Coordination Layer as an Excitation-Wavelength-dependent Dual-Emissive Chemosensor for Discriminating Fe3+, Cr3+, and Al3+ in Water, Inorg. Chem., 2023, 62, 13453–13466 CrossRef CAS.
- W.-T. Chen, M.-J. Tsai and J.-Y. Wu, A Thermally Stable Undulated Coordination Layer Showing a Sequentially Interweaving 2D → 3D Net as a Turn-On Sensor for Luminescence Detection of Al3+ in Water, Cryst. Growth Des., 2022, 22, 226–236 Search PubMed.
- P.-M. Chuang and J.-Y. Wu, A highly stable Zn coordination polymer exhibiting pH-dependent fluorescence and as a visually ratiometric and on−off fluorescence sensor, CrystEngComm, 2021, 23, 5226–5240 RSC.
- C.-H. Su, M.-J. Tsai, W.-K. Wang, Y.-Y. Li and J.-Y. Wu, Engineering Tailored Bifunctional Luminescent Pillared-Layer Frameworks for Adsorption of CO2 and Sensitive Detection of Nitrobenzene in Water Media, Chem. – Eur. J., 2021, 27, 6529–6537 CrossRef CAS PubMed.
- Y.-W. Huang, P.-M. Chuang and J.-Y. Wu, Solvent-Induced Controllable Supramolecular Isomerism: Phase Transformation, CO2 Adsorption, and Fluorescence Sensing toward CrO42−, Cr2O72−, MnO4− and Fe3+, Inorg. Chem., 2020, 59, 9095–9107 CrossRef CAS PubMed.
- J. G. Santaclara, F. Kapteijn, J. Gascon and M. A. van der Veen, Understanding metal–organic frameworks for photocatalytic solar fuel production, CrystEngComm, 2017, 19, 4118–4125 RSC.
- S. Y. Song, X. Z. Song, S. N. Zhao, C. Qin, S. Q. Su, M. Zhu, Z. M. Hao and H. J. Zhang, Syntheses, structures and physical properties of transition metal–organic frameworks assembled from trigonal heterofunctional ligands, Dalton Trans., 2012, 41, 10412–10421 RSC.
- P. Xing, D. Wu, J. Chen, J. Song, C. Mao, Y. Gao and H. A. Niu, Cd-MOF as a fluorescent probe for highly selective, sensitive and stable detection of antibiotics in water, Analyst, 2019, 144, 2656–2661 RSC.
- Y. Wang, L. Wang, W. Huang, T. Zhang, X. Hu, J. A. Perman and S. Ma, A metal–organic framework and conducting polymer based electrochemical sensor for high performance cadmium ion detection, J. Mater. Chem. A, 2017, 5, 8385–8393 RSC.
- M. Lu, Y. Deng, Y. Luo, J. Lv, T. Li, J. Xu, S.-W. Chen and J. Wang, Graphene Aerogel−Metal−Organic Framework-Based Electrochemical Method for Simultaneous Detection of Multiple Heavy-Metal Ions, Anal. Chem., 2019, 91, 888–895 CrossRef CAS PubMed.
Footnote |
† Electronic supplementary information (ESI) available: Additional structure figures, EDX analysis, IR spectra. CCDC 2322407 (1). For ESI and crystallographic data in CIF or other electronic format see DOI: https://doi.org/10.1039/d3dt04379g |
|
This journal is © The Royal Society of Chemistry 2025 |
Click here to see how this site uses Cookies. View our privacy policy here.